Abstract
One of the challenges for toxicological assessment of inhaled aerosols is to accurately predict their deposited and absorbed dose. Transport, evolution, and deposition of liquid aerosols are driven by complex processes dominated by convection-diffusion that depend on various factors related to physics and chemistry. These factors include the physicochemical properties of the pure substance of interest and associated mixtures, the physical and chemical properties of the aerosols generated, the interplay between different factors during transportation and deposition, and the subject-specific inhalation topography. Several inhalation-based physiologically based pharmacokinetic (PBPK) models have been developed, but the applicability of these models for aerosols has yet to be verified. Nicotine is among several substances that are often delivered via the pulmonary route, with varied kinetics depending upon the route of exposure. This was used as an opportunity to review and discuss the current knowledge and state-of-the-art tools combining aerosol dosimetry predictions with PBPK modeling efforts. A validated tool could then be used to perform for toxicological assessment of other inhaled therapeutic substances. The Science Panel from the Alliance of Risk Assessment have convened at the “Beyond Science and Decisions: From Problem Formulation to Dose-Response Assessment” workshop to evaluate modeling approaches and address derivation of exposure-internal dose estimations for inhaled aerosols containing nicotine or other substances. The discussion involved PBPK model evaluation criteria, challenges, and choices that arise in such a model design, development, and application as a computational tool for use in human toxicological assessments.
Introduction
Pulmonary delivery of substances is of great interest to the medical and scientific community and for public health. The route of aerosol administration offers several advantages, such as faster onset of action, improved bioavailability, easier noninvasive administration, high permeability, and a large absorptive surface area in the lungs, importantly, avoiding first-pass metabolism in the liver (Labiris and Dolovich Citation2003). Performing toxicity assessments, as well as efficacy testing for substances of interest, is of equal importance. Inhalation, however, is not a typical route of administration for drugs outside the realm of respiratory diseases, and as a result, progress on aerosol drug development has been limited and primarily focused on treating airway diseases, such as asthma, cystic fibrosis, and chronic obstructive pulmonary disease (COPD) (Labiris and Dolovich Citation2003). Typically, achieving intravenous (IV)-like plasma concentrations with oral administration is challenging and may require large oral doses, but these doses would lead to higher systemic and gastrointestinal (GI) exposures and potential toxicity. Pulmonary delivery enables rapid absorption and IV-like plasma concentrations with a smaller dose. Simultaneously, certain substances such as fluticasone are deposited in the lung and are not instantaneously absorbed into the systemic circulation or cleared (Borghardt et al. Citation2018). Hence, for therapeutic substances without instantaneous pulmonary absorption and requiring prolonged exposures, inhalation may result in a favorable benefit/risk ratio. With advancements in science and technology, the respiratory tract (RT) is being studied as a route of drug delivery for a wide variety of substances, including therapeutics for diabetes and preventive vaccines (Valdespino-Gomez et al. Citation2006).
The major challenge in inhalation toxicity or efficacy assessment is the appropriate and accurate determination of the delivered dose. An inhaled dose is more difficult to quantify than the dose delivered via other routes of administration, as the complex physiological process of inhalation makes the estimation of delivered dose challenging (Alexander et al. Citation2008). For example, inhalation of dry solid particles would result in a certain dose depending on factors such as the properties of both the aerosol (e.g. particle size distribution and their abilities concerning hygroscopic growth) and the individual (e.g. exertion level, breathing patterns, and RT morphology and physiology). After deposition in the lung, some particles dissolve and absorb into the systemic/pulmonary circulation, while others are cleared from the lung by pulmonary metabolism and mucociliary clearance (MCC). Similarly, substances forming liquid aerosols may change their phase presence by evaporation process and become absorbed from the gas phase modulating the delivered dose. The U.S. Environmental Protection Agency has derived methods for determining inhalation reference concentrations for dosimetry adjustments, but the applicability for inhaled aerosols still needs to be evaluated (IPCS Citation2005; U.S.EPA Citation2014). To further review, a panel of experts discussed the model structure, evaluation criteria, and applicability of inhalation physiologically based pharmacokinetic (PBPK) modeling for aerosolized nicotine or other substances in a toxicological assessment framework. In this review, various factors and associated challenges that were identified and need to be considered for PBPK modeling of inhaled aerosol are discussed. Nicotine was chosen as an example because of its varied pharmacokinetics depending upon route of administration and public interest. A well developed and validated model for nicotine could be used to investigate the challenges associated in delivery of therapeutic substances. After a brief summary of nicotine pharmacokinetics (PK), aerosol characteristics, and influencing factors, inhalation PBPK models representing various complexities are described. Further, given the highly complex process and incomplete development, future approaches for PBPK modeling of inhaled aerosol are outlined. This review is expected to help regulatory bodies understand the limitations of existing models and the potential influence of various factors in deriving dose for toxicological assessment, and further bridge the gap between aerosol dosimetry and PBPK modeling researchers for developing improved strategies.
Nicotine PK
Nicotine, one of the most abundant tobacco alkaloids, has been reported to exert several pharmacological effects on the brain and GI system (Benowitz et al. Citation2009). Nicotine permeates the blood-brain barrier, and its effects are mediated through binding to nicotinic acetylcholine receptor (nAChR) subtypes, particularly those located on dopaminergic neurons in the ventral tegmental area. Upon stimulation, dopamine is released in the shell of the nucleus accumbens, which is an important mechanism in drug-induced reward-motivated behavior. Changes in dopamine levels are also supported by nicotine-induced release of other neurotransmitters, such as glutamate (that facilitates the release of dopamine) and γ-aminobutyric acid (that inhibits dopamine release), in these brain areas. The absorption, distribution, metabolism, and excretion (ADME) properties of nicotine have been studied in humans and pre-clinical species (Benowitz et al. Citation2009). Nicotine has a very low plasma protein binding and is rapidly metabolized by the liver, primarily by the cytochrome P450 (CYP) 2A6 (and to a lesser extent by CYP2B6 and CYP2E1), to cotinine, its main metabolite (80% of nicotine conversion) (Benowitz et al. Citation2009).
Inhaling nicotine in cigarette smoke is the most rapid form of nicotine delivery known and peaks at the completion of smoking (). The arterial boli and high venous blood nicotine concentrations are produced within seconds and minutes, respectively, as the lungs offer a large surface area for absorption and a favorable dissolution pH of 7.4 (Benowitz et al. Citation2009). Further inhalation enables faster delivery of nicotine to the brain next to IV administration (Benowitz et al. Citation2009). The rapid central nervous system delivery facilitates binding to nAChRs and increases dopamine levels, resulting in reward-motivated behavior. Cigarettes, however, are a dangerous nicotine delivery system. Smokers are far more likely than nonsmokers to develop heart disease, lung cancer, and COPD (U.S. DHHS Citation2014). The best way to avoid the harms of smoking is never to start, and for smokers, the best way to reduce the harms of smoking and the risk of tobacco-related disease is to quit. For decades, the goal of reducing the harm caused by smoking has, therefore, focused on preventing smoking initiation and promoting smoking cessation.
Figure 1. Nicotine concentrations in blood during and after consumption of cigarette, oral snuff, chewing tobacco, nicotine gum, nicotine patch, and THS 2.2. Data obtained from Benowitz et al. (Citation2009), Cipolla and Gonda (Citation2015), and Picavet et al. (Citation2015).
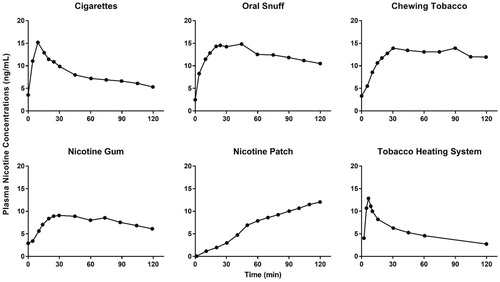
Chewing tobacco and oral snuff lead to absorption of nicotine in oral mucosa and by the small intestine rather than in the stomach because of its pH-dependent ionization. Although the peak levels attained after chewing tobacco may approximate those attained by smoking, the shape of the plasma concentration versus time is different, and the time required to reach peak level is longer (). Nicotine plasma levels are maintained transiently after the cigarette smoke is inhaled, rather than the more gradual and sustained elevation after oral ingestion (Benowitz et al. Citation2009). In addition, oral snuff can damage the linings of the oral cavity and oropharynx, causing cells to grow more rapidly to repair DNA damage and leading to oral cancer (ACS Citation2015).
Nicotine replacement therapy (NRT) aims to reduce the motivation to consume tobacco and the physiological and psychomotor withdrawal symptoms while still delivering nicotine and has been shown to be less harmful than consuming tobacco (Silagy et al. Citation2004; Prochaska Citation2015). NRT products are delivered in various forms, including gum, transdermal patch, nasal spray, oral inhaler, and tablet. Transdermal delivery of nicotine via a patch is successful, as the nicotine penetrates rapidly, allowing the delivery of fairly large doses. The nicotine patch is a slow, sustained-release form of nicotine delivery that is intended to gradually lower users’ dependence on tobacco and ultimately eliminate this dependence () (Benowitz et al. Citation2009). Acute dosing products allow users to titrate the timing and dose of nicotine. Products such as nicotine gum and lozenges are buffered to alkaline pH to facilitate increased absorption. Despite their formulations, NRTs were unable to mimic the plasma concentrations of nicotine following inhalation, as the absorption of nicotine from the buccal cavity is slower, and a portion of the dose is swallowed and subjected to first-pass metabolism (Benowitz et al. Citation2009). Nicotine nasal spray is absorbed more rapidly than other NRTs but does not reach the maximum nicotine concentration (Cmax) of inhaled nicotine, thus requiring larger doses (Lunell et al. Citation2000).
To accelerate the decline in smoking prevalence and reduce smoking-related population harm, candidate modified risk tobacco products (MRTP) are being developed (U.S.FDA Citation2012). To effectively encourage smokers to switch to MRTPs, sufficient delivery of nicotine is an important attribute (Institute of Medicine Citation2012). For this reason, the U.S. Food and Drug Administration has also recommended that MRTP Applications must provide an assessment of the product’s “speed and efficiency of nicotine delivery” (U.S.FDA Citation2012). Philip Morris Products S.A. has developed the Tobacco Heating System (THS), a candidate MRTP (U.S.FDA Citation2017). The THS consists of a device that heats, but does not burn, a proprietary tobacco stick (HeatSticks®), creating an inhalable nicotine-containing aerosol that provides a range of consumer sensory attributes that appeal to adult smokers. The THS aerosol contains significantly reduced quantities and numbers of toxicants in comparison with cigarette smoke (Schaller et al. Citation2016). PK and pharmacodynamics (PD) studies were designed to assess the nicotine uptake profile in adult smokers who use THS. During the clinical studies, the extent and rate of nicotine absorption during single-stick ad libitum use of THS were measured and compared with those of cigarettes (Picavet et al. Citation2015; Brossard et al. Citation2017). These studies also evaluated the relationship between plasma nicotine concentrations and the suppression of the urge to smoke in adult smokers and yielded initial safety data on product use (e.g. vital signs, clinical biochemistry, hematology, spirometry, electrocardiography, and adverse events). shows that nicotine from THS has a similar PK profile (e.g. time to reach Cmax [Tmax] and Cmax) to nicotine from cigarettes. Therefore, when taken together, the studies demonstrated that THS provided similar amounts of nicotine as combustible cigarettes (CCs) (i.e. the area under the curve for THS was 77.4% of CCs) and at a similar rate to CCs (the Tmax for THS was same as CCs and the Cmax of THS was 70.3% of CCs) (Picavet et al. Citation2015; Brossard et al. Citation2017). Inhalation of an liquid aerosol containing nicotine provided rapid systemic delivery; however, further understanding of the fate of inhaled aerosol is needed to improve toxicological assessment of substances beyond nicotine.
Aerosol delivery
Aerosol delivery is complex, spans over many scientific disciplines and has been studied thoroughly over the past decades. A comprehensive introductory overviews of the aerosol delivery have been written by Hinds (Citation2012), Ruzer and Harley (Citation2012), Phalen (Citation2008) and Finlay (Citation2001). In this section, we will briefly guide the reader through the main topics relating to aerosol inhalation dosimetry, starting with a brief description of aerosol generation and characterization methods followed by a discussion of aerosol evolution, transport and deposition in the lungs.
Aerosol generation and characterization
Various ways of aerosol formation (e.g. nebulization, nucleation, atomization, and dispersion of manufactured spray-dried powders) cause solid and/or liquid particles to become suspended in the carrier gas. Methods for characterizing aerosol particles can generally be divided into chemical and physical methods. Chemical aerosol characterization includes methods for evaluating substances in all phases (gas, solid, liquid) of the aerosol mixture and methods for measuring the most important physiochemical properties (e.g. viscosity, surface tension, boiling point, solubility) of substances used to generate and deliver an aerosol. Physical characterization methods for determining aerosol particle densities, morphologies, surface properties and interaction forces (most important for solid particles) and methods for determining the aerosol particle size distribution, which is widely considered the most important aerosols in terms of inhalability and deposition. The particle size distribution gives information about the number and sizes of a flowing polydisperse aerosol. The amount of the delivered aerosol dose is directly linked to the particle size distribution because aerosol deposition is mainly controlled by the physical mechanisms that are dependent on the physical sizes of the particles (Nordlund and Kuczaj Citation2015).
The chemical composition and physical properties of an aerosol will affect the transport, evolution, and deposition of the aerosol mixture in the RT, as is further discussed below. One of the key barrier to the delivery of a substance through inhalation is the complex relationship between the physicochemical characteristics of the aerosol formulation and potential performance of aerosol in the humid environment of the RT (Haddrell et al. Citation2017). The properties of liquid and solid substances in aerosols (e.g. molecular weight, lipophilicity (log P), solubility, pKa, protein binding, vapor pressure, surface tension, polar surface area, and electrostatic charge) will affect deposition of the substances in the RT. For example, increasing the pH markedly improves absorption of nicotine as base form is non-ionized and more hydrophilic (Benowitz et al. Citation2009).
Devices using different principles (e.g. time-of-flight, light-scattering and electrical mobility) have been developed to determine the particle sizes in aerosols () (Allen Citation2013). To deliver significant doses, aerosols are generated with higher particle density leading altered particle size distributions due to increased coalescence. Furthermore, liquid aerosols are complex chemical mixtures containing various substances, such as the active ingredient, solvents, wetting agents, anti-oxidants, preservatives, stabilizers, volatile liquids, surfactants, flavors, and inert materials. The range of substances present affects the particle size and, for solid particles, the particle density, shape, surface roughness, and surface area. The factors mentioned above can be used to manipulate the aerodynamic properties of an aerosol.
Aerosol evolution, transport and deposition
Aerosols produced from nicotine and other therapeutic delivery systems are affected by external conditions that can change the particle size distribution, shift in phase and composition of a multispecies aerosol through phase partitioning. Furthermore, the transport, evolution, and deposition of aerosols are dependent on the surrounding thermal and chemical environment and the flow conditions. Linking the local exposure in the respiratory system with the measured dose and target tissue dose is, therefore, the ultimate goal of aerosol dosimetry research. The physicochemical mechanisms that govern the transport and deposition of aerosols in the RT are described below. Detailed discussions of aerosol dosimetry aspects, particle kinetics, and lung interaction mechanisms are available in a number of publications (Schulz et al. Citation2000; Phalen and Hoover Citation2006; Phalen et al. Citation2010; Tsuda et al. Citation2013; Phalen and Raabe Citation2016). Here, we briefly describe key points relating to inhaled liquid aerosols.
Aerosol evolution
The main physical mechanisms governing the evolution of a liquid aerosol during inhalation are evaporation (caused by dilution with inhaled air), condensation (caused by the high relative humidity in the airways), and coalescence (coagulation) () (Hinds Citation2012). Evaporation of the liquid phase decreases the particle size or, for very small particles, decreases the particle number density because some liquid particles completely evaporate, particularly particles at the lower end of a polydisperse aerosol distribution. Condensation of vapors onto existing particles increases particle size, resulting in increased upper airway RT deposition. Condensation can also result in deposition of aerosol particles on the walls of the RT, caused in particular by warm and humid conditions. It is important to mention that in the case of liquid multispecies mixtures, the physicochemical properties of the mixture (e.g. surface tension, boiling point) depend on the mixture composition itself and often cannot be superimposed as a simple function of the species properties.
Most aerosols are delivered with intended high particle number density (directly linked with the amount of delivered substance), causing simultaneous coalescence, and a process where larger particles are formed as a result of collisions between 2 or more smaller particles. The kinematic forces (i.e. impact of external forces or aerodynamic effects) and the Brownian motion of the particles can influence coalescence. Because of the complexity involved in aerosol evolution, several studies have assumed constant particle size during residence in the lungs, perhaps leading to inaccurate prediction of deposition.
Aerosol transport
Transport or uptake of an aerosol into the RT is driven by the negative pressure (with respect to the ambient atmosphere) caused by the contraction of the diaphragm that results in expansion of the chest cavity and intrapleural space (Hinds Citation2012). The resulting pressure gradient between the atmosphere and the thoracic cavity generates airflow by which the aerosol particles enter the RT. The flow rate is relatively high in the upper airways as the geometric dimensions are large causing to a convection-driven downward flow. Whereas, airflow in the mouth, nose and pharynx is often complex causing turbulence and possible circulating vortices during exhalation. Further down the RT, the flow rate is lower in each branch and the geometric dimensions are smaller, making convection less pronounced and diffusion becomes the driving force. The inhalation/exhalation flow rates vary depending on breathing rates, inhalation patterns and geometric RT dimensions; these factors will strongly affect particle transport (Kleinstreuer and Zhang Citation2010). The mechanisms governing aerosol transport in the RT are shown in . Aerosol transport will directly affect both the evolution and deposition of the aerosol particles because the factors affecting transport will act as size-dependent filtration mechanisms.
Transport of an evolving aerosol is influenced by several factors; the dominant factors include the humidity and spatio-thermal conditions in the RT. The chemical composition of the aerosol and external factors will affect partitioning of the different phases in an aerosol mixture and will, therefore, affect particle deposition and absorption which in turn affect the dose-response of the substance under investigation.
Aerosol deposition
The total deposited dose of an aerosol is dependent on both particulate (i.e. solid and/or liquid) and gaseous phases. Primary mechanisms of particle deposition include impaction, diffusion, interception, sedimentation, and electrostatic precipitation, as shown in (Hinds Citation2012; Nordlund and Kuczaj Citation2015). It must be noted that modulation of the physical aerosol characteristics mentioned earlier (particle size distribution) directly affects the magnitude and subsequently the physical location of the aerosol deposition governed by these mechanisms. The absorption of gases depends on several factors, such as solubility, diffusivity, surrounding temperature, humidity, and concentration (Nordlund and Kuczaj Citation2015). Combination of both phenomena (aerosol particles deposition and gas phase absorption) contributes to the overall substance-specific dosimetry quantification. These substances are then either further absorbed into the lung tissue structure or cleared by MCC.
Factors influencing inhalation PBPK modeling
Studies of aerosol delivery systems largely focus on the formulation of the substance and the device used to generate and deliver the aerosol, or the PK and the efficacy of the inhaled substance. As a direct result, the aerosol characteristics determined using a benchmark system (i.e. product characteristics determined in laboratory tests) will not represent the actual performance during real inhalation conditions (i.e. studies in clinic and real world application). In recent years, significant efforts have been dedicated to improving our understanding of delivered aerosol doses and our ability to extrapolate doses from experimental systems using computational methods. The challenges involved in of predicting and understanding the key processes that determine pulmonary exposure to inhaled substances remain, and are the subject of ongoing research and validation (Phalen et al. Citation2010). One of the key challenges in developing and validating an evolving aerosol model for nicotine, as well as other substances, is the lack of experimental tools available for measuring aerosol characteristics and deposition in living subjects. Understanding how the chemical composition and physical characteristics of aerosols influence deposition and drug absorption (permeability, tissue affinity) in complex lung geometries, and their impact on physiological processes (including MCC and metabolism), would be beneficial. Computer-based mechanistic modeling and aerosol dosimetry provide an opportunity to explore these questions, but significant gaps still exist. The challenges are broadly grouped into various categories, as shown in and are discussed below.
Figure 4. Specific aspects that need to be considered in a PBPK model of an inhaled aerosol to perform a toxicological assessment include physicochemical characterization of the aerosol (particle size distribution, gas/liquid/solid phase partitioning), assessment of the inhalation topography (the temporal flow rate distribution during lung ventilation), subject or population-specific assessment of the lung morphology (volume, dimensions and airway structure), PBPK modeling (using a compartmental model structure with relevant parameters), coupling of dosimetry and PBPK models, and assessing the risks involved in translating information from in vitro to in vivo and rodent to human models.
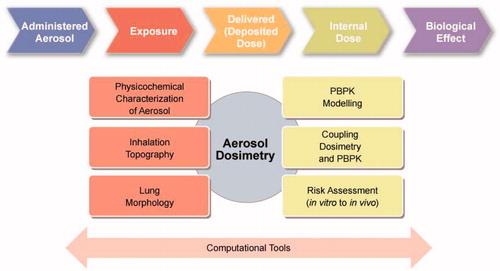
Inhalation topography
Inhalation topography determines the amount of a substance available for absorption and will be different for voluntary and involuntary exposures. Involuntary exposures (e.g. occupational or environmental exposure) typically lasts for several hours to days, and modeling of inhalation patterns is probably not very important because the concentrations tend to reach a steady state. However, for a self-administered, ultra-short, or short exposures ranging from few seconds to couple of minutes, the inhalation patterns influence the delivered dose and the PK significantly (. The inhalation topography is comprised of several stages, such as puff volume/duration, mouth hold time, inhalation volume/duration, breath hold, and exhalation volume/duration (Vas et al. Citation2015). The duration and volumes at each stage are known to vary not only across individuals but also within an individual puffing pattern. The rates of inspiration for a normal tidal breathing pattern could result in a differential deposition compared to a slow inspiration rate with a breath hold or a forced inspiration without a breath hold. Clinically, these breathing patterns have been tested for various products, such as metered dose inhalers, and guidance is available for efficiently delivering the substance (. However, there is no guidance available for other commercial products, resulting in free self-administration and varied PK. For example, studies have reported that changes in product design can impact puffing intensity. In particular, when switching from a high- to low-yield smoking product, people tend to take larger puffs with an increase in puffing frequency. Further, during cigarette consumption, a smoker may have large inspiratory volumes for a few initial puffs and lower volumes at the end of puffing (Benowitz et al. Citation2009). Clearly, inhalation patterns vary across individual subjects, resulting in different exposures. Thus, there is a requirement to analyze and benchmark inhalation patterns for ADME following aerosol exposures to develop and validate a population-based PBPK model.
Anatomy and physiology of the RT
Differences in the RT anatomy exist both within species and among species at each level of the RT (. There is limited knowledge on the influence and deposition of mouth and nose geometries on the delivery of aerosols for rodents and humans. Rodents predominantly exhibit a monopodial lung branching system, whereas humans have an irregular dichotomous and trichotomous branching pattern of the airways (Harkema et al. Citation2013). The effects of these various branching patterns on airflow distribution, gas uptake, and the deposition of particles have not been sufficiently studied to determine the extent to which branching patterns impart regional non- homogeneities or local variations in the deposition of inhaled substances. To date, detailed morphometric data used to calculate aerosol particle deposition are very limited (Rostami Citation2009). The scientific community have been using 3D reconstruction techniques to examine various aspects of lung structure. Studies vary from the reconstruction of individual cells to reconstructing conducting airway and alveolar duct branching systems. The human whole-lung measurements are obtained based on limited subjects and are currently the gold standard predictions for modeling purposes (Raabe et al. Citation1976). However, these data are limited and are not representative of a population-level difference. Obtaining realistic geometry including the branching system is critical for mathematical calculations of aerosol dosimetry. The branching system from trachea to terminal bronchioles involves an average path length of 16 branches, while shorter path lengths may be 8 to 10 branches (Weibel Citation1963a; Raabe et al. Citation1976; Yeh et al. Citation1979). The tissue thickness decreases down the RT, and the expression of cells varies along the RT. This may lead to differential partitioning, retention, absorption, and clearance rates via the mucociliary escalator. After deposition, the compound may be subjected to phagocytosis by different cell types such as pulmonary alveolar macrophages and alveolar epithelial cells, or it may enter the alveolar interstitium which would increase compound retention. The compound retention is mainly driven by the physicochemical properties of the compound and to some extent aerosol particle redistribution (Snipes Citation1989). Mucus is a highly viscous gel-like fluid lining the RT with an estimated replenishment rate of 3-5 mm/min and a clearance time of 15 min to 2 h for trapped substances (Duncan et al. Citation2016). To this end, preliminary dissolution experiments to investigate chemical-specific dissolution rates of aerosol mixtures in artificial mucus (Jug et al. Citation2018) and in vitro experiments using 3D tissue models to determine diffusion would be beneficial (Hoffmann et al. Citation2018). Further in vitro evaluation of mechanistic biology, such as expression of CYP enzymes, active transporters, and lysosomal trapping mechanisms, would enable a more reliable prediction of pulmonary PK.
Aerosol dosimetry
Predicting dose due to inhalation processes is a difficult task. The methods/approaches described in this section are based on various modeling efforts and issues that need to be further developed and validated to be applicable for inhalation, in particular for evolving aerosols. A tremendous amount of work has been done by several groups to understand dosimetry for inhalation exposures, but most of it was focused on gas exposure or for non-evolving aerosols containing solid particles (Ramsey and Andersen Citation1984; Csanady et al. Citation1994; U.S.EPA Citation1994; Anjilvel and Asgharian Citation1995; Kumagai and Matsunaga Citation1995; Sarangapani, Teeguarden, et al. Citation2002; Phalen et al. Citation2010; Boger and Wigstrom Citation2018).
The Association of Inhalation Toxicologists have proposed a methodology for determining delivered dose in rats based on concentration of the substance in air, respiratory minute volume, duration of exposure, body weight, and an arbitrary inhalation fraction (Alexander et al. Citation2008). A more holistic determination of dose calculation must account for at least the aerosol physicochemical properties, inhalation topography, and RT anatomy. Several varieties of the so-called whole-lung models have been developed for estimating deposited dose. These models are based on simplified transport processes, including lung morphometry data and semi-empirical aerosol deposition correlations (). They can be classified into groups concerning the level of scrutiny at which the geometrical upper RT branching is taken into account (i.e. single/multiple-path models) and 2 distinct ways to deal with complexities related to the lower RT (i.e. deterministic/stochastic models). Among these, the historic International Commission on Radiological Protection (ICRP) 66 inhalation model is based on the particle filtration efficiency at different regions of the lung (IRCP Citation1994). This approach is purely driven by particle size and does not account for any aerosol evolution during its transport along the RT. Similarly, the multiple-path particle dosimetry (MPPD) model developed by Applied Research Associates and started by pioneering work performed by Asgharian (Anjilvel and Asgharian Citation1995; Asgharian and Anjilvel Citation1998) resulted in the release of the publicly available MPPD software package (Price et al. Citation2002). This is one of the most advanced models capturing solid particle physics deposition mechanisms (e.g. impaction, sedimentation, and diffusion phenomena) for a large number of flow scenarios and various airway geometries. Progress in understanding the aerosol evolution processes and their influence on deposition for multispecies aerosol mixtures is ongoing. Developed Computational Fluid Dynamics (CFD) approaches are still far away from applicability to inhalation case scenarios, including full lung geometry, but can be used to validate simplified models. Growing computational capabilities allow for application of CFD, in which transport, evolution, and deposition equations are solved directly and locally in the human- or rodent-specific geometry of the upper RT (Haghnegahdar et al. Citation2019). In this case, it is possible to obtain the exact numerical solutions with respect to the flow. The accounted aerosol physics still needs to contain modeling simplifications due to limitations concerning computational feasibility. Two generally distinct fundamental approaches were developed. The Lagrangian method allows tracking of each particle separately, usually treating them mathematically as point particles containing mass, while the Eulerian method computes particle fluxes through the computational domain, approximating particle physics on the basis of phenomenological models (e.g. mass transfer correlations around a single particle in a flow, taking into account heat transfer equations). Both methods have been successfully applied and validated for non-evolving aerosols while being under continuous development and improvement concerning evolving liquid aerosols that are closely linked and dependent on the aerosol chemistry. To particularly tackle the liquid evolving multispecies aerosols, an open-source code method, AeroSolvedTM, was developed to simulate aerosol flows (see the Aerosolved (Citation2019) reference for the repository code). With the applied Eulerian approach, particular attention was paid to the ability to simulate high particle number density flows at varying thermodynamic conditions. Although CFD models are able to provide accurate calculations of the deposited dose for an aerosol, the reliability of these tools still remain to be validated with respect to assumptions standing behind them.
Rodent exposure studies for human extrapolation
Animal models have been essential and have provided a wealth of information for aerosol studies that needs to be extrapolated to humans (Phalen et al. Citation2008). The exposure systems for inhalation toxicological testing of liquid aerosols on rodents depends on the experimental question. While several systems have been developed for evaluating nicotine and other substances in preclinical rodents, these can be divided into (1) aerosol nebulizer, (2) smoking machine and (3) a vacuum based system (Miliano et al. Citation2019). Aerosol nebulization is a the most preferred as it delivers precise amount to the animal and but lacks the heating element found in the devices. In general, the temperature of the heating element is controlled to generate aerosol with minimum heat-induced chemical degration. Aerosol from smoking machines captures testing of any heating induced chemical degration by-products and will need regular cleaning for long term exposure studies. Vacuum exposure systems involve replacement of ambient air with aerosol by creating a negative pressure in the chamber housing the rodents. Although, these systems are preferred for long term studies, the delivered dose is challeneging to determine given the different inhalation pattern or exposure type (nose-only or whole body). Preclinical inhalation studies performed to evaluate the pulmonary pharmacokinetics of a substance usually involve intratracheal administration (Gunerka et al. Citation2015; Hendrickx et al. Citation2018). These studies need to be performed taking into consideration the conditions and types of exposures, including non-physiological deposition patterns during intratracheal administration and, more importantly, the different breathing patterns of humans and animals exposed to aerosols. Humans can be exposed by either nasal or oral breathing, while most rodents are obligatory nasal breathers. In the case of rodents, the nose acts as an efficient particle filter, thereby limiting the particle size range that can be delivered to the lungs compared to humans (Forbes et al. Citation2011). Humans can be cooperative subjects and can participate in protocols involving variable breathing rates and tidal volumes as well as both nasal and oral breathing. Human subjects can also perform tests of physiological response that are less feasible for test animals. By contrast, most animal inhalation studies have been restricted to quiescent nasal breathing. Human studies are limited to those focused on short-term exposures and transient physiological responses. By contrast, animal studies can include long-term exposures, serial termination, and pathological assays. Experimentation on rodents usually involves using the same aerosol developed for human inhalation experiments. As rodents have much smaller airways, the penetration of the same size particles is then much more limited than in the case of humans. The differences in aerosol inhalaibility and experimental variability caused by the whole-body (or nose-only) exposure of rodents may lead to varied exposure and PK profiles. Moreover, a direct extrapolation using the regional deposited dose ratio from rodents to human could lead to different reference doses (U.S.EPA Citation1994).
Dosimetry-relevant compartmental PBPK model structure requirements
The PBPK modeling approach uses a classical 1 D system-level simulations in which the physiologically relevant parts of the body (i.e. compartments) are selected and the effects of these compartments on the selected substance distribution characteristics (i.e. PK) are simulated by solving (coupled ordinary differential) equations for transport between the compartments. Once the PBPK model is parametrized and validated, it will delivers robust, simplified, and useful estimates of PK data under various conditions that will support regulatory decisions (Zhao et al. Citation2011). Ongoing advances in the physiology field allows the relevant processes to be better captured and allow increasingly complex models to be developed while delivering more refined outcomes for the users (Hester et al. Citation2011). The increasing complexity must be balanced by relevant and required outcomes. There remains several challenges when developing PBPK models of inhalation (Forbes et al. Citation2011). Inhalation is a complex process, and the necessary fit-for-purpose modeling compartmental framework still needs to be determined. Inhalation PBPK models for an inhaled aerosol should include an RT and GI tract along with other compartments representing tissues of relevance for the substance in question. Initial inhalation PBPK models developed for occupational or environmental exposures are comprised of either 1, 3, or 4 respiratory compartments (). Lately, PBPK models are being developed that include all 24 generations of the RT (Backman et al. Citation2018; Boger and Wigstrom Citation2018), but the necessity for inclusion of this level of detail remains to be validated and benchmarked with other models. Moreover, inhalation PBPK models coupled to computational dosimetry approaches (whole-lung or CFD-coupled) are recommended for development while simultaneously accounting for accuracy versus feasibility and practical use.
Existing PBPK models for inhalation
PBPK modeling is a mechanistic approach for predicting concentration-time profiles derived from pulmonary PK (e.g. dissolution, absorption) and systemic PK characteristics (e.g. distribution, excretion) by incorporating various anatomical and physiological aspects (e.g. tissue volumes, blood flow rates) and drug formulation characteristics (e.g. pKa, solubility, partition coefficients, protein binding). These models are often advanced and require considerable prior information to increase the credibility of predictions. Moreover, in human health risk assessment, we assume a 100% absorption of the deposited fraction in the RT in the absence of an absorption factor and a 75% absorption for indirect exposures (HERAG Citation2007; ECETOC Citation2010), but in reality, the chemical is either absorbed, precipitated, or cleared, and PBPK modeling enables prediction of systemic available/internal doses after inhalation of complex chemical mixtures in various forms. Previously developed PBPK methods and their limitations in modeling of inhaled aerosols are described below and categorized in the following sections by the level of anatomical lung representation.
PBPK model with non-anatomical lung representation
Early PBPK models, developed for inhalation of chemicals such as styrene, described the lung compartment as a single uniform tissue with gas-exchange dynamics at the portal entry and closed-form equilibrium relations () (Ramsey and Andersen Citation1984; Csanady et al. Citation1994). The PBPK model captured the arterial blood and tissue concentrations but assumed that inhaled air flow rate was equal to the alveolar ventilation rate while attaining a rapid equilibrium with capillary blood per the specified blood:air partition coefficient.
Kumagai and Matsunaga further developed the PBPK model for acetone exposure by including the mucus layer of the inhaled and exhaled air tract and a compartment for gas exchange within the lung compartment () (Kumagai and Matsunaga Citation1995). The mucus layer representing the outermost mucus lining in the wall of the air tract was 5–10 µm in thickness, but the model assumed it to be 2 µm with a volume of 1 ml. The model also omitted the deeper mucous layer and the wall tissue, failing to capture instantaneous equilibrium of acetone between the deeper portion of the mucous layer and air. A simulation performed to assess the impact of the mucous layer volume of an air tract showed that an increase in mucous volume lowered acetone concentrations in blood and increased concentrations in exhaled air, indicating the importance of accurate descriptions and volumes. Although the plasma PK profiles were fitted, predictions and extrapolation to other substances, formulations, or populations could be challenging, owing to the simplicity of the model and the lack of sufficient description of the airway region. Gajewska et al. used the same formulation to study the impact of daily nicotine intake from cigarette smoke on heart rate (Gajewska et al. Citation2014). The model predicted nicotine blood concentrations for cigarettes containing low, typical, and high levels of nicotine. The model could also predict exposures as smoke reached deeper lung regions (i.e. alveoli), where more than 90% of nicotine is absorbed. The model assumed that there was constant inhalation of nicotine of an absolute dose of 0.05 mg/L and an average smoking time of 3 to 4 min.
These models did not account for the rapid changes in pulmonary blood concentrations that may arise during the first few seconds after initiation or cessation of an inhalation exposure, for the bidirectional cyclic nature of actual alveolar air flow in the lungs, or for clearance. As the time frames of interest are sufficiently large, the dynamic interactions between alveolar air and pulmonary blood were captured.
PBPK model with anatomical lung representation
Major advancements were made by Bogdanffy et al., who constructed a PBPK 5-compartment model of the rat nasal cavity and a 4-compartment model of the human nasal cavity (Bogdanffy et al. Citation1999) to describe vinyl acetate exposures. The nasal uptake-focused model not only represented the species-specific differences in anatomy but also incorporated air-phase resistance to mass transfer from the lumen to the air–mucus interface. This inclusion led to absorption of the chemical from the airway lumen to mucus, epithelial layer, submucosa, and blood. Sarangapani et.al constructed a multi-compartmental RT model () by dividing the RT into 3 regions (nasal cavity, conduction airways, and pulmonary airway representing the alveolar gas exchange region) to describe exposures of inhaled vapors (Sarangapani, Clewell, et al. Citation2002). The nasal cavity and conducting airways had a 3-layer substructure, similar to that of the Bogdanffy et al. model (Bogdanffy et al. Citation1999). Inhaled air ventilated the lumen of these airway compartments. A chemical in the lumen was available for uptake into the epithelial lining tissues or passage to the subsequent airway compartment. The submucosal tissue layer was perfused by blood and cleared the absorbed volatiles from the airway compartments.
A similar PBPK model, but with 4 lung regions, was developed by Sarangapani et al. with special emphasis on characterizing the transitional bronchiolar compartment for inhaled gases (Sarangapani, Teeguarden, et al. Citation2002). This model assumed that the air flow rate in the upper and conducting airways was equal to the respiratory minute volume and that the flow rate in the transitional and pulmonary airways was equal to the alveolar ventilation. A fraction of inhaled chemical was absorbed in the nasal cavity, conducting airways, and transitional airways. The dominant process that delivered the substance to epithelial tissues in the nose was airflow. By developing a more complete description of the regions of the lungs, it now has become possible to assess the role of airway equilibration in controlling dose-response curves for metabolism in tissues all along the airways of the RT.
These models were suitable for volatile substances, with an assumption that drug concentrations in the air are homogeneous and that passive inhalation occurs for long durations, but they did not hold true for short-duration, voluntarily inhaled aerosolized substances, as the inhalation rates and drug concentrations vary.
PBPK models with extensive anatomical lung representation
Several mechanistic pulmonary PBPK models were developed primarily to guide the development of inhaled medicines. Boger et al. predicted the fate of a poorly soluble inhaled drug, fluticasone propionate, in rats (Boger et al. Citation2016). A combined framework of drug- and formulation-specific properties and system-specific inputs were investigated using a computational PBPK model to predict lung selectivity (ratio of local to systemic target occupancy). The deposition of inhaled particles was assumed to occur only in the nose and lung, as the exposure was a nose-only inhalation. The lung compartment was divided into 3 sub-compartments where the deposition of particles may occur (nose [representing only nasal cavity], central [tracheobronchial] region, and peripheral [alveolar] region). The deposited dose was subjected to MCC, resulting in a plasma PK profile that is based on parallel absorption from the lung, nose, and GI tract. The time course of glucocorticoid receptor occupancy in the lungs was measured in vivo following inhalation and IV administration of the drug, and the model predicted the drug’s PK and receptor occupancy.
Recently, Merck developed a mechanistic model to predict pulmonary PK during respiration (Cabal, Mehta, et al. Citation2016; Caniga et al. Citation2016). The model included deposition in various lung generations, substance dissolution, MCC, and a PD module to a traditional PBPK model. PK of inhaled dry powders of Mometasone furoate, Budesonide, Salbutamol, and Formoterol obtained from pre-clinical species and humans were validated using the developed model. The particle deposition of these dry powders was obtained by performing a series of in vitro experiments (Caniga et al. Citation2016). The model did not account for MCC and the spatial aspects of the deposition but instead used a fixed value of the drug distribution in airways and alveoli. Predicting the systemic exposures of multiple puffs may be challenging, as the deposition and clearance may vary. Cabal et al. also developed a similar model by coupling flow and deposition equations to understand deposition, MCC, dissolution, absorption, transport, distribution, partition, and action of the chemical (Cabal et al. Citation2016). This lung model consisted of 24 airway branches implemented in a series of parallel semi-rigid cylindrical tubes and then connected to a series of bifurcation trees. Although the model details are not fully published, it seems to be comprehensive for predicting tissue exposures.
Commercial software packages with anatomical lung representation
GastroPlus™ (GastroPlus™ Nasal-Pulmonary Compartmental Absorption and Transit Model, SimulationsPlus Inc., Lancaster, CA, USA) is currently the only commercially available mechanistic computer program that combines a physiological PBPK model with mechanistic models accounting for pulmonary deposition, dissolution, and absorptive and non-absorptive clearance (. The program considers 3 distinct pulmonary regions (large and small conducting airways, the alveolar interstitium [AI]) and 1 extrathoracic (ET) compartment, essentially based on the Weibel lung model (Weibel Citation1963b). The regions are further subdivided into an airway liquid compartment and an epithelial/lung tissue compartment, allowing the user to input parameters. The deposition of inhaled drug is estimated based on an inbuilt whole-lung ICRP deposition model (IRCP Citation1994). The model allows input of user-defined deposition rates for the 4 compartments only. Following particle deposition, the software uses Noyes-Whitney principles to mechanistically model dissolution based on actual particle and mucus characteristics. The particles in the ET compartment are cleared to the GI tract, and their absorption is then calculated by an advanced compartmental absorption and transit model. Aspects such as region-specific MCC, metabolism, and mucus binding are already included in the software. The model lacks inclusion of dose fractions that could be deposited in the GI tract during nose-only inhalation of aerosols in pre-clinical species. A description of lung geometry and deposition for pre-clinical species needs to be included to perform any translation across species.
Figure 7. Schematic diagram for the inhalation models in commercially available software. (A) GastroPlus™, (B) PulmoSim™, (C) SimCyp Simulator™. Adapted from Borghardt et al. (Citation2015).
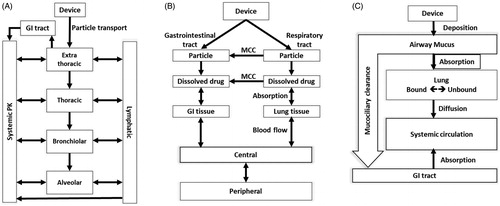
Commercial software packages with extensive anatomical lung representation and no PBPK model description
Several generic software packages, such as PulmoSim™, have been developed to model PK of inhaled substances. In PulmoSim™, the inhaled fraction is separated into 2 fractions, one entering the lung and the other entering the GI tract () (Collingwood et al. Citation2012). Particles entering the lung are either absorbed to the lung tissue post-dissolution in mucus or cleared by the MCC into the GI tract. The model consists of 15 differential equations describing dissolution, absorption, tissue binding, systemic distribution, and clearances. The absorption of the drug by pulmonary tissue is influenced by the physiochemical properties of the substance. For example, a substance or formulation with lower dissolution rates tends to be cleared rather than being absorbed by the tissue. Similarly, the unbound fraction of the substance and its affinity to lung tissue will have an effect on pulmonary retention and transit times. Unfortunately, there are no publications describing pulmonary and systemic exposures using PulmoSim™ software. Collingwood et al. have mentioned the correlation of estimated pulmonary concentrations to regional concentrations to be challenging, leading to an under-prediction of pulmonary concentrations (Collingwood et al. Citation2012).
Other non-mechanistic inhalation PK model software packages incorporating a model of deposition, dissolution, non-absorptive clearance, and absorptive clearance include SimCyp Simulator™ (Certara), PK-SIM™ (Computational Systems Biology: Bayer AG), and Mimetikos Preludium™ (Emmace consulting). SimCyp Simulator™ uses a first-order absorption non-mechanistic inhalation model to describe absorption across the lung for any substrate and/or inhibitors. This model can be used with the full PBPK model as well as the 1-compartment distribution model (. Stass et al. used PK-SIM™ to deconvolute PK data obtained in healthy volunteers after inhalation of ciprofloxacin, a locally acting antibiotic, to obtain the relative contribution of oral-, tracheobronchial- (BB and bb), and AI-deposited drug to the total systemic exposure (Stass et al. Citation2013). The authors did not mechanistically model local absorptive and non-absorptive clearance processes. They assumed the AI dose to behave as an IV dose and the conducting airway dose to behave as a delayed oral dose and then used PK-SIM™ to fit the systemic PK profiles based on these assumptions. Similar to SimCyp Simulator™, the PK-SIM™ PBPK model also requires an input of deposited dose, whereas the Mimetikos Preludium™ software package estimates the regional deposition of inhaled aerosols in the human RT and includes a PK module. The regional deposition of mono- or polydisperse aerosols in the airways of adults and children ranging from 0.01–50 µm particle size can be calculated. Mechanistic description of pulmonary processes, such as dissolution, barrier permeation, and MCC, are included, but a 2-compartmental model is used for estimating distribution and clearances.
Future of inhalation PBPK models
How to best combine CFD and PBPK models
From the previous sections, it is clear that PBPK modeling of prolonged inhalation of gases and single-puff non-evolving aerosols has already been quite successful. In our schematic categorization, depicted in , we have distinguished between 3 main approaches that use 1) simple dose estimations, 2) whole-lung modeling approaches, or 3) CFD modeling versus increasing levels of complexity (number of compartments), delivered information, and computational costs. The simple dose estimation approach was touted as a way forward for PBPK modeling of inhaled substances such as gases (Sarangapani, Teeguarden, et al. Citation2002). In this case, models represented the RT in 1 to 3 compartments and while reasonable predictions were obtained, the influence of formulation alterations on performance on a purely virtual basis has proven elusive. More recently, several groups have started developing inhalation PBPK models by coupling CFD and PBPK (Backman et al. Citation2018; Boger and Wigstrom Citation2018). Although these are more informative, the coupled models are computationally intensive, and their application needs extensive validation efforts due to complexity. The approach using whole-lung modeling by identifying and tuning the key influencing components using CFD, followed by their implementation in a simplified manner in the PBPK model to capture the relevant responses, is probably the most effective and recommended way aiming at practicality and accuracy. This approach can bridge the gap to predict dose exposures based on physicochemical properties and practical application of PBPK in today’s risk assessment paradigms.
Validation of lung deposition
Lung deposition data can be obtained by either experimental or computational approaches (in this case in particular for the upper RT), or in specific situations, by a combination of both. Existing computational approaches have been often developed and validated against animal data. Several in vivo experimental methods, such as single-photon emission computed tomography, positron emission tomography, and γ-scintigraphy, require a radioactively labeled substance to determine particle deposition in the lung (Frohlich et al. Citation2016). These methodologies often require highly technically skilled personnel and specific tracers, and may need alterations of substance or formulation to prevent clearance (Frohlich et al. Citation2016). Experimental approaches are limited in accuracy and resolution due to the limitations of experimental methods, difficulties in the administration of inhalable products (potential contamination caused by radio-labeled substances), and ethical reasons (limited information concerning humans). Three-dimensional imaging techniques allow various types of information to be extracted (Tay et al. Citation2018), including the penetration index, which represents the extent of particles reaching the alveoli (Clark Citation2012), but data are mostly indicative of the total lung deposition and low-resolution. Currently, obtaining such data for humans is challenging, and advances in technology over the next few years may enable collection of such information. Hence, the focus is placed on either measuring concentrations upstream or downstream, such as in plasma, sputum, or bronchoalveolar lavage fluid, to later deconvolute using a computational model to predict deposited fractions (Smith et al. Citation1986; Clewe et al. Citation2015; Kolli et al. Citation2019). The growing computational capabilities have allowed development and application of numerical models with increasing levels of complexity that are overcoming the aforementioned experimental limitations, as described in the section concerning dosimetry.
Predicting variability across the population
Systemic bioavailability of certain substances is greater upon delivering via inhalation compared to the oral route, as it avoids first-pass metabolism but often exhibits a high degree of between- and within-subject variability. In addition to the variability due to differential expression of enzymes across the population and disease conditions, inhalation topography leads to a varied amount of inhaled dose. For example, a pulsed delivery of nitric oxide improved uptake efficiency compared to constant concentration delivery, and different pulse timing led to intra-inter-subject variability in dosing (Martin et al. Citation2014). Similarly, cigarette smokers switching from high- to low-yield nicotine cigarettes are known to optimize smoking patterns to extract different levels of nicotine (Benowitz et al. Citation2009). Further, absorption of the substance is affected by physiological factors and the physiochemical properties of the substance. For example, nicotine in base form is highly permeable and rapidly absorbed, whereas quaternary amines, such a tiotropium, ipratropium, and glycopyrronium, are retained in the lung tissue either due to significantly slower passive cellular permeability or OCT1-mediated transport (Hendrickx et al. Citation2013). Moreover, an increased understanding of MCC and the influence of mucus rheology on dissolution of formulations in mucus needs to be further studied (Lai et al. Citation2009). A mechanistic model capturing the intrinsic and extrinsic factors affecting inhalation delivery including levels of variability and covariates could improve the prediction of variability across the population. Efforts are being focused towards improving our understanding of the factors that affect dosimetry in diseased conditions such as COPD (Ganguly et al. Citation2019).
Evaluating criteria for inhalation PBPK modeling success
Prior to evaluating PBPK success, it is important to establish the evaluation criteria for success of inhalation PBPK models. Although the primary goal would be to fully predict the plasma and tissue concentration-time profiles for inhaled substances, the model structure is determined by the objectives for which a PBPK model is being developed. If data following IV and oral administration are available and the model parameters are estimated, it should be possible to deconvolute the amount of inhaled compound absorbed from the GI tract and the RT (Kolli et al. Citation2019). The model predictive performance for a substance can be evaluated by assessing the model’s ability to predict clinical outcomes with a consistent set of parameters, and in the case of further refinement, validation using independent data set will enable assessment of model performance. Because of biological variations or experimental errors, PBPK model estimated parameters will always have some variance; hence, performing sensitivity, uncertainty and variability analysis improves the confidence in PBPK models.
Concluding remarks
Toxicological assessment of inhaled aerosols is challenging due to a high degree of uncertainty in determining delivered doses of substances. Aerosols are continuously evolving during transport and are influenced by various factors, such as physical properties (e.g. particle size distribution), chemical composition, inhalation topography, and lung morphology. There is a need to develop a reliable inhalation PBPK model integrating the physicochemical properties of aerosol delivery, in vitro data, and relevant physiology to predict dose responses. Such models should be in a position to predict dose responses based on changes in the physicochemical properties of substances present in the aerosol mixture (i.e. gas and liquid/solid phases), which in turn are dependent on the inhalation characteristics of the aerosol mixture. For development of such models, substances like nicotine can be used. Nicotine can be present in all phases simultaneously, significantly affecting the PK and thus enabling the development of a framework that can be applied to other substances.
A new inhalation PBPK modeling method for dose exposure predictions may need to be developed taking the aforementioned factors into account, as current inhalation PBPK modeling methods are aimed at occupational/environmental dose exposure predictions for either prolonged exposures (of solid particles, vapors, or gases) or short exposures (solid powders). These models are simplified and suffer from the lack of mechanistic details of the physicochemical and physiological aspects involved in self-administration of inhaled aerosols. The recommendations are to further invest in the development of the whole-lung modeling approach, taking advantage of detailed CFD simulations and novel experimental methods for validation of data acquisition. The recommendation also comes from the practical gain of information versus accuracy resolution. From this perspective, the level of detail obtained on the dosimetry side should match required and existing biological modeling capabilities (e.g. airway generation number versus number of PK compartments for the lungs).
Over the years, very little information has been obtained on lung morphology and inhalation topography. Recent advancements in imaging technologies could help to reconstruct lung morphology for the wide variety within the population. Pharmaceutical companies might generate large amount of data, archive it upon program termination and not publicly available. Such information complied into a large database could aid validation of PBPK models for identifying dosimetry paradigms and toxicological outcomes.
To advance the understanding of toxicological assessment of inhaled substances, PMI Research and Development has worked on various fronts, including development of advanced in vitro models, in vivo characterization, and quantitative modeling approaches. The development of advanced in vitro platforms, such as InHALES (Steiner et al. Citation2018), facilitates the determination of regional deposition based on different inhalation patterns, and the lung/liver-on-a-chip platform (Bovard et al. Citation2018) captures the transport and metabolism of a deposited aerosol to predict exposure responses for any given substance. Further, to translate in vivo experimental outcomes across species, it is key to obtain reliable airway morphology measurements, capturing the differences, and to validate the deposited dose using a quantitative methodology. To this extent, measurements for tracheobronchial airway geometry from 4 different strains of mice were measured (Hoeng et al. Citation2019). Several mathematical approaches have been developed to understand the delivered dose of an evolving aerosol in an in vitro system and in vivo cast models for toxicological assessments (Frederix et al. Citation2018; Lucci et al. Citation2018). Recently, a more realistic segmented upper RT cast model was constructed and housed to control temperature during air flow (Asgari et al. Citation2019). The thermal equilibrium of the air flow in the system was evaluated using experimental and computational approaches to enable a more reliable prediction of deposited dose for an evolving aerosol. The development of these dosimetry models paved a path for development and validation of semi-mechanistic inhalation PBPK models by implementing the key features required to relate exposure, delivered dose, and internal dose. Among those, there is a PBPK model developed to understand the regional absorption of inhaled aerosol based on the PK profile (Kolli et al. Citation2019). The upcoming versions of inhalation PBPK models for evolving aerosol could include the evolution of aerosol in a simplified representation of the RT, capturing the key geometries and physiological processes.
The development and validation of such models require coordinated efforts from academia and industry to address the associated challenges. Further, pooling information and challenges could lower method development costs while increasing productivity. Due to the various levels of complexities and multidisciplinary nature, it would be beneficial if a continued dialogue between leading experts and regulators across various fields could take place to ensure that relevant experiments are performed and critical parameters are accounted for in development of novel methods and models. Such a highly collaborative environment should ensure quality and reproducibility of models along with details describing their applicability and limitations. An aerosol inhalation PBPK model estimating internal dose would be beneficial for toxicological assessment for a wide variety of inhaled substances.
Declaration of interest
Aditya Reddy Kolli, Arkadiusz K. Kuczaj, Florian Martin, Manuel C. Peitsch and Julia Hoeng are employees of Philip Morris International (PMI). A.Wallace Hayes is a paid consultant for PMI. PMI is the sole source of funding and sponsor of this research. Prior to the submission, the manuscript was submitted to PMI for internal review for the sole purpose to check that the article did not violate PMI’s proprietary information. The internal review did not result in any changes in the content of the article.
Manuel C. Peitsch is the Chief Scientific Officer of PMI, which he joined in 2008 following 15 years in the pharmaceutical industry (GSK and Novartis). MCP is responsible for all scientific matters pertaining to the assessment of candidate Modified Risk Tobacco Products (MRTP, as defined by the U.S. FDA) developed by PMI, which include heated tobacco products as well as e-cigarettes. MCP led the submission of the regulatory dossier for IQOS, PMI’s leading candidate MRTP, to the U.S. FDA as well as to other regulatory bodies world-wide. MCP leads the product assessment teams across pre-clinical toxicology, systems toxicology and clinical studies. MCP regularly gives scientific presentations on Tobacco Harm Reduction and the scientific assessment of PMI’s candidate MRPTs at conferences and at other scientific fora. MCP is fully paid by PMI and owns PMI shares (NYSE:PM), which is his sole source of revenue.
Julia Hoeng (JH) is Global Head of Systems Toxicology department at PMI R&D. Arkadiusz K. Kuczaj (AKK), Florian Martin (FM) and Aditya Reddy Kolli (ARK) are Manager, Principal Scientist and Scientist respectively in Department of Systems Toxicology at PMI R&D. AKK holds an Associate Professor position at the Department of Applied Mathematics, University of Twente, the Netherlands. JH, AKK and FM have contributed towards scientific matters and regulatory submissions pertaining to candidate Modified Risk Tobacco Products (MRTP, as defined by the U.S. FDA) developed by PMI, which include heated tobacco products as well as e-cigarettes. JH, AKK and FM attend scientific conferences to present on Tobacco Harm Reduction and the scientific assessment of PMI’s candidate Modified Risk Tobacco Products (MRTP, as defined by the U.S. FDA). ARK has not been involved in the last five years with regulatory or legal proceedings related to the contents of this paper. ARK presents data and models related to nicotine and other research findings at scientific conferences. JH, AKK, FM and ARK are fully paid by PMI.
A.Wallace Hayes is a professor at University of South Florida, Tampa, USA. Previously, AWH was Vice President and Corporate Toxicologist for RJR Nabisco with responsibility for all regulatory and toxicology issues related to the safety of ingredients and food contact substances for food and drink products worldwide. Subsequently, as Corporate Vice-President of Product Integrity at the Gillette Company, AWH had management responsibility for the safety evaluation and regulatory compliance of a variety of consumer products, plant safety, environmental stewardship, and quality control. AWH served on numerous committees and expert panels for the National Academy of Sciences, the National Institutes of Health, the Food and Drug Administration, the Environmental Protection Agency and the Department of Defense. AWH is currently a member of the Food Advisor Committee, US Food and Drug Administration and Food Quality Protection Act Science Review Board, US EPA. AWH has served on a number of GRAS Expert Panels. AWH is the editor-in-chief, Human and Experimental Toxicology, Cutaneous and Ocular Toxicology and Toxicology Research and Application, and coeditor of the Target Organ Toxicity Series and Hayes’ Principles and Methods of Toxicology 6th Edition. AWH is a past Secretary General of IUTOX (two terms), past treasurer and board member of the American Board of Toxicology, a past president of the American College of Toxicology, the Toxicology Education Foundation, and the Academy of Toxicological Sciences and a past member of the council of the Society of Toxicology.
This article captures the discussions from the Alliance of Risk Assessment (ARA) conducted workshop series titled “Beyond Science and Decisions: From Problem Formulation to Comprehensive Risk Assessment” and is sponsored by a number of organizations through case study reviews, donations or grants. The purpose of the workshop is to develop risk methods compendium that could serve as a resource for regulators and scientists while applying dose-response or exposure assessment techniques for various problems. The members of the “Beyond Science and Decisions” Science Panel at the ARA workshop evaluate the research case study methods to enhance the risk evaluation framework. The Science Panel members also discuss the input on utility of case study and further method development but are not limited to a specific chemical. A list of current sponsors include American Chemistry Council, Center for Food Safety and Applied Nutrition of the US Food and Drug Administration, Consortium for Environmental Risk Management LLC (CERM), E Risk Sciences, LLP, Exponent, Georgia Pacific, Gradient, International Association of Plumbing and Mechanical Officials – Research and Testing, The LifeLine Group, Lucy Fraiser Toxicology Consulting LLC, National Institute of Occupational Safety and Health, National Institute of Environmental Health Sciences, Nickel Producers Environmental Research Association, Ramboll, Summit Toxicology, Texas Commission on Environmental Quality, Toxicology Excellence for Risk Assessment, US Army Public Health Center. PMI provided financial contribution to ARA for the review and discussion of a research case study on physiologically based pharmacokinetic (PBPK) modeling of inhaled aerosols and the placement of a summary of this case study on the ARA website. This contribution also supports the continued review and discussion of a research case study, the development of a report describing these discussions, and the placement of a summary of this case study on the ARA research risk assessment framework, subject to Science Panel approval. The financial contribution solicited for this research event was restricted to its associated expenses. The ARA is a collaboration of many groups, with Toxicology Excellence for Risk Assessment (TERA), a 501c3 nonprofit corporation, being its financial administrator. The ARA and TERA provided no goods or services to PMI, other than intangible scientific and social benefits, in return for this contribution.
Acknowledgements
We would like to thank the Beyond Science and Decisions Science Panel from the Alliance of Risk Assessment Workshop and all the participants for their discussions. We thank Michael L. Dourson and Jean Binder for coordinating the case study preparation and workshop organization. We also thank Samantha Elmhurst for the graphical illustrations. The authors acknowledge the useful comments of the reviewers selected by the Editor and not known to the authors.
References
- ACS. 2015. Patient Pages. Health risks of smokeless tobacco. J Okla State Med Assoc. 108(11):518–520.
- Aerosolved. 2019. Philip Morris Products SA; [accessed June 2019]. http://aerosolved.com/.
- Alexander DJ, Collins CJ, Coombs DW, Gilkison IS, Hardy CJ, Healey G, Karantabias G, Johnson N, Karlsson A, Kilgour JD. 2008. Association of Inhalation Toxicologists (AIT) working party recommendation for standard delivered dose calculation and expression in non-clinical aerosol inhalation toxicology studies with pharmaceuticals. Inhal Toxicol. 20(13):1179–1189.
- Allen T. 2013. Particle size measurement. Netherlands: Springer.
- Anjilvel S, Asgharian B. 1995. A multiple-path model of particle deposition in the rat lung. Toxicol Sci. 28(1):41–50.
- Asgari M, Lucci F, Bialek J, Dunan B, Andreatta G, Smajda R, Lani S, Blondiaux N, Majeed S, Steiner S, et al. 2019. Development of a realistic human respiratory tract cast representing physiological thermal conditions. Aerosol Sci Technol. 53:860–870.
- Asgharian B, Anjilvel S. 1998. A multiple-path model of fiber deposition in the rat lung. Toxicol Sci. 44(1):80–86.
- Backman P, Arora S, Couet W, Forbes B, de Kruijf W, Paudel A. 2018. Advances in experimental and mechanistic computational models to understand pulmonary exposure to inhaled drugs. Eur J Pharm Sci. 113:41–52.
- Benowitz NL, Hukkanen J, Jacob P. 3rd. 2009. Nicotine chemistry, metabolism, kinetics and biomarkers. Handb Exp Pharmacol. (192):29–60.
- Bogdanffy MS, Sarangapani R, Plowchalk DR, Jarabek A, Andersen ME. 1999. A biologically based risk assessment for vinyl acetate-induced cancer and noncancer inhalation toxicity. Toxicol Sci. 51(1):19–35.
- Boger E, Evans N, Chappell M, Lundqvist A, Ewing P, Wigenborg A, Friden M. 2016. Systems pharmacology approach for prediction of pulmonary and systemic pharmacokinetics and receptor occupancy of inhaled drugs. CPT Pharmacometrics Syst Pharmacol. 5(4):201–210.
- Boger E, Wigstrom O. 2018. A partial differential equation approach to inhalation physiologically based pharmacokinetic modeling. CPT Pharmacometrics Syst Pharmacol. 7(10):638–646.
- Borghardt JM, Kloft C, Sharma A. 2018. Inhaled therapy in respiratory disease: the complex interplay of pulmonary kinetic processes. Can Respir J. 2018:1.
- Borghardt JM, Weber B, Staab A, Kloft C. 2015. Pharmacometric models for characterizing the pharmacokinetics of orally inhaled drugs. AAPS J. 17(4):853–870.
- Bovard D, Sandoz A, Luettich K, Frentzel S, Iskandar A, Marescotti D, Trivedi K, Guedj E, Dutertre Q, Peitsch MC, et al. 2018. A lung/liver-on-a-chip platform for acute and chronic toxicity studies. Lab Chip. 18(24):3814–3829.
- Brossard P, Weitkunat R, Poux V, Lama N, Haziza C, Picavet P, Baker G, Ludicke F. 2017. Nicotine pharmacokinetic profiles of the Tobacco Heating System 2.2, cigarettes and nicotine gum in Japanese smokers. Regul Toxicol Pharmacol. 89:193–199.
- Cabal A, Jajamovich G, Mehta K, Guo P, Przekwas A. 2016. In-silico lung modeling platform for inhaled drug delivery. Drug Delivery to the Lungs Conference (DDL) 7-9 Dec. [assessed 2019 Nov 28]. https://aerosol-soc.com/assets/uploads/2016/11/03.Cabal_.pdf
- Caniga M, Cabal A, Mehta K, Ross DS, Gil MA, Woodhouse JD, Eckman J, Naber JR, Callahan MK, Goncalves L, et al. 2016. Preclinical experimental and mathematical approaches for assessing effective doses of inhaled drugs, using mometasone to support human dose predictions. J Aerosol Med Pulm Drug Deliv. 29(4):362–377.
- Cipolla D, Gonda I. 2015. Inhaled nicotine replacement therapy. Asian J Pharma Sci. 10(6):472–480.
- Clark AR. 2012. Understanding penetration index measurements and regional lung targeting. J Aerosol Med Pulm Drug Deliv. 25(4):179–187.
- Clewe O, Karlsson MO, Simonsson US. 2015. Evaluation of optimized bronchoalveolar lavage sampling designs for characterization of pulmonary drug distribution. J Pharmacokinet Pharmacodyn. 42(6):699–708.
- Collingwood S, Coe D, Pryde D, Lock R. 2012. Respiratory drug discovery, current developments and future challenges: Highlights from the Society of Medicines Research Symposium, held on June 14th, 2012—Horsham, UK. Drugs Fut. 37(8):619.
- Csanady GA, Mendrala AL, Nolan RJ, Filser JG. 1994. A physiologic pharmacokinetic model for styrene and styrene-7,8-oxide in mouse, rat and man. Arch Toxicol. 68(3):143–157.
- Duncan GA, Jung J, Hanes J, Suk JS. 2016. the mucus barrier to inhaled gene therapy. Mol Ther. 24(12):2043–2053.
- ECETOC. 2010. Guidance on Assessment Factors to Derive a DNEL. Brussels: European Centre for Ecotoxicology, Toxicology of Chemicals.
- Finlay WH. 2001. The mechanics of inhaled pharmaceutical aerosols: an introduction. London: Academic press.
- Forbes B, Asgharian B, Dailey LA, Ferguson D, Gerde P, Gumbleton M, Gustavsson L, Hardy C, Hassall D, Jones R, et al. 2011. Challenges in inhaled product development and opportunities for open innovation. Adv Drug Deliv Rev. 63(1–2):69–87.
- Frederix EMA, Kuczaj AK, Nordlund M, Bělka M, Lizal F, Jedelský J, Elcner J, Jícha M, Geurts BJ. 2018. Simulation of size-dependent aerosol deposition in a realistic model of the upper human airways. J Aerosol Sci. 115:29–45.
- Frohlich E, Mercuri A, Wu S, Salar-Behzadi S. 2016. Measurements of deposition, lung surface area and lung fluid for simulation of inhaled compounds. Front Pharmacol. 7:181.
- Gajewska M, Worth A, Urani C, Briesen H, Schramm KW. 2014. The acute effects of daily nicotine intake on heart rate – a toxicokinetic and toxicodynamic modelling study. Regul Toxicol Pharmacol. 70(1):312–324.
- Ganguly K, Carlander U, Garessus ED, Friden M, Eriksson UG, Tehler U, Johanson G. 2019. Computational modeling of lung deposition of inhaled particles in chronic obstructive pulmonary disease (COPD) patients: identification of gaps in knowledge and data. Crit Rev Toxicol. 49(2):160–173.
- Gunerka P, Hucz-Kalitowska J, Turowski P, Zagozda M, Dziachan M, Sekular M, Bujak A, Wieczorek M. 2015. Pharmacokinetics of selective PI3Kδ inhibitors after intratracheal instillation in mice. Eur Res J. 46(suppl 59):PA2123.
- Haddrell AE, Lewis D, Church T, Vehring R, Murnane D, Reid JP. 2017. Pulmonary aerosol delivery and the importance of growth dynamics. Ther Deliv. 8(12):1051–1061.
- Haghnegahdar A, Zhao J, Kozak M, Williamson P, Feng Y. 2019. Development of a hybrid CFD-PBPK model to predict the transport of xenon gas around a human respiratory system to systemic regions. Heliyon. 5(4):e01461.
- Harkema JR, Nikula KJ, Haschek WM. 2013. Chapter 51 – Respiratory System. In: Haschek WM, Rousseaux CG, Wallig MA, editors. Haschek and Rousseaux's Handbook of Toxicologic Pathology. 3rd ed. Boston: Academic Press; p. 1935–2003.
- Hendrickx R, Johansson JG, Lohmann C, Jenvert RM, Blomgren A, Borjesson L, Gustavsson L. 2013. Identification of novel substrates and structure-activity relationship of cellular uptake mediated by human organic cation transporters 1 and 2. J Med Chem. 56(18):7232–7242.
- Hendrickx R, Lamm Bergstrom E, Janzen DLI, Friden M, Eriksson U, Grime K, Ferguson D. 2018. Translational model to predict pulmonary pharmacokinetics and efficacy in man for inhaled bronchodilators. CPT Pharmacometrics Syst Pharmacol. 7(3):147–157.
- HERAG. 2007. Health risk assessment guidance for metals: Assessment of occupational inhalation exposure and systemic inhalation absorption. EBRC; [accessed 2019 Nov 15]. https://www.ebrc.de/downloads/HERAG_FS_02_August_07.pdf.
- Hester RL, Brown AJ, Husband L, Iliescu R, Pruett D, Summers R, Coleman TG. 2011. HumMod: a modeling environment for the simulation of integrative human physiology. Front Physiol. 2:12.
- Hinds WC. 2012. Aerosol technology: properties, behavior, and measurement of airborne particles. New York: John Wiley & Sons.
- Hoeng J, Foong C, Oldham MJ, Lucci F, Cockram S, Luke S, Yeo D, Chua J, Peitsch MC, Kuczaj A. 2019. A method for determination of tracheobronchial airway geometries from four different strains of mice. FASEB J. 33(1):lb107–lb107.
- Hoffmann W, Gradinaru J, Farcal L, Caul-Futy M, Huang S, Wiszniewski L, Parissis N, Morath S, Fortaner S, Cole T, et al. 2018. Establishment of a human 3D tissue-based assay for upper respiratory tract absorption. Appl Vitro Toxicol. 4(2):139–148.
- Institute of Medicine. 2012. Scientific standards for studies on modified risk tobacco products. Washington, DC: The National Academies Press.
- IPCS. 2005. Chemical-specific adjustment factors for interspecies differences and human variability: guidance document for use of data in dose/concentration-response assessment. Vol. 2. World health organization.
- IRCP. 1994. Human respiratory tract model for radiological protection. A report of a Task Group of the International Commission on Radiological Protection. Ann ICRP. 24(1–3):1–482.
- Jug M, Hafner A, Lovrić J, Kregar ML, Pepić I, Vanić Ž, Cetina-Čižmek B, Filipović-Grčić J. 2018. An overview of in vitro dissolution/release methods for novel mucosal drug delivery systems. J Pharm Biomed Anal. 147:350–366.
- Kleinstreuer C, Zhang Z. 2010. Airflow and particle transport in the human respiratory system. Annu Rev Fluid Mech. 42(1):301–334.
- Kolli A, Martin F, Xiang Y, Titz B, Wong E, Schlage WK, Veljkovic E, Vanscheeuwijck P, Hoeng J. 2019. Physiologically based pharmacokinetic modeling of nicotine from inhaled aqueous aerosol predicts marked gastrointestinal absorption in rats. American society for clinical pharmacology and therapeutics. Clin Pharm Ther. 105(S1):S5–S121.
- Kumagai S, Matsunaga I. 1995. Physiologically based pharmacokinetic model for acetone. Occup Environ Med. 52(5):344–352.
- Labiris NR, Dolovich MB. 2003. Pulmonary drug delivery. Part I: physiological factors affecting therapeutic effectiveness of aerosolized medications. Br J Clin Pharmacol. 56(6):588–599.
- Lai SK, Wang YY, Wirtz D, Hanes J. 2009. Micro- and macrorheology of mucus. Adv Drug Deliv Rev. 61(2):86–100.
- Lucci F, Castro ND, Rostami AA, Oldham MJ, Hoeng J, Pithawalla YB, Kuczaj AK. 2018. Characterization and modeling of aerosol deposition in Vitrocell® exposure systems – exposure well chamber deposition efficiency. J Aerosol Sci. 123:141–160.
- Lunell E, Molander L, Ekberg K, Wahren J. 2000. Site of nicotine absorption from a vapour inhaler – comparison with cigarette smoking. Eur J Clin Pharmacol. 55(10):737–741.
- Martin AR, Jackson C, Katz IM, Caillibotte G. 2014. Variability in uptake efficiency for pulsed versus constant concentration delivery of inhaled nitric oxide. Med Gas Res. 4(1):1.
- Miliano C, Scott ER, Murdaugh LB, Gnatowski ER, Faunce CL, Anderson MS, Reyes MM, Gregus AM, Buczynski MW. 2019. Modeling drug exposure in rodents using e-cigarettes and other electronic nicotine delivery systems. J Neurosci Methods.:108458.
- Nordlund M, Kuczaj AK. 2015. Aerosol dosimetry modeling using computational fluid dynamics. In: Julia H, Manuel CP, editors. Computational systems toxicology. New York, NY: Springer; p. 393–427.
- Phalen R, Raabe O. 2016. The evolution of inhaled particle dose modeling: a review. J Aerosol Sci. 99:7–13.
- Phalen RF. 2008. Inhalation studies: foundations and techniques. Boca Raton: CRC Press.
- Phalen RF, Hoover MD. 2006. Aerosol dosimetry research needs. Inhal Toxicol. 18(10):841–843.
- Phalen RF, Mendez LB, Oldham MJ. 2010. New developments in aerosol dosimetry. Inhal Toxicol. 22(sup2):6–14.
- Phalen RF, Oldham MJ, Wolff RK. 2008. The relevance of animal models for aerosol studies. J Aerosol Med Pulm Drug Deliv. 21(1):113–124.
- Picavet P, Haziza C, Lama N, Weitkunat R, Lüdicke F. 2015. Comparison of the pharmacokinetics of nicotine following single and ad libitum use of a tobacco heating system or combustible cigarettes. NICTOB. 18(5):557–563.
- Price O, Asgharian B, Miller F, Cassee F, de Winter-Sorkina R. 2002. Multiple Path Particle Dosimetry model (MPPD v1. 0): a model for human and rat airway particle dosimetry. RIVM Rapport: 650010030.
- Prochaska JJ. 2015. Nicotine replacement therapy as a maintenance treatment. JAMA. 314(7):718–719.
- Raabe OG, United S, Energy R, Development A, National Institute of Environmental Health S, Lovelace Foundation for Medical E, Research, Commission USAE, Division of B, Environmental R. 1976. Tracheobronchial geometry: human, dog, rat, hamster: a compilation of selected data from the project respiratory tract deposition models.
- Ramsey JC, Andersen ME. 1984. A physiologically based description of the inhalation pharmacokinetics of styrene in rats and humans. Toxicol Appl Pharmacol. 73(1):159–175.
- Rostami AA. 2009. Computational modeling of aerosol deposition in respiratory tract: a review. Inhal Toxicol. 21(4):262–290.
- Ruzer LS, Harley NH. 2012. Aerosols handbook: measurement, dosimetry, and health effects. Boca Raton: CRC press.
- Sarangapani R, Clewell HJ, Cruzan G, Andersen ME. 2002. Comparing respiratory-tract and hepatic exposure-dose relationships for metabolized inhaled vapors: a pharmacokinetic analysis. Inhal Toxicol. 14(8):835–854.
- Sarangapani R, Teeguarden JG, Cruzan G, Clewell HJ, Andersen ME. 2002. Physiologically based pharmacokinetic modeling of styrene and styrene oxide respiratory-tract dosimetry in rodents and humans. Inhal Toxicol. 14(8):789–834.
- Schaller J-P, Keller D, Poget L, Pratte P, Kaelin E, McHugh D, Cudazzo G, Smart D, Tricker AR, Gautier L, et al. 2016. Evaluation of the tobacco heating system 2.2. Part 2: chemical composition, genotoxicity, cytotoxicity, and physical properties of the aerosol. Regul Toxicol Pharmacol. 81(Suppl 2):S27–S47.
- Schulz H, Brand P, Heyder J. 2000. Particle deposition in the respiratory tract. Lung Biol Health Dis. 143:229–277.
- Silagy C, Lancaster T, Stead L, Mant D, Fowler G. 2004. Nicotine replacement therapy for smoking cessation. Cochrane Database Syst Rev. 11(3):CD000146.
- Smith MJ, White LO, Bowyer H, Willis J, Hodson ME, Batten JC. 1986. Pharmacokinetics and sputum penetration of ciprofloxacin in patients with cystic fibrosis. Antimicrob Agents Chemother. 30(4):614–616.
- Snipes MB. 1989. Long-term retention and clearance of particles inhaled by mammalian species. Crit Rev Toxicol. 20(3):175–211.
- Stass H, Nagelschmitz J, Willmann S, Delesen H, Gupta A, Baumann S. 2013. Inhalation of a dry powder ciprofloxacin formulation in healthy subjects: a phase I study. Clin Drug Investig. 33(6):419–427.
- Steiner S, Herve P, Majeed S, Pak C, Frentzel S, Hoeng J. 2018. Development of a novel in vitro aerosol exposure system: The Independent Holistic Air-Liquid aerosol exposure system (InHALES). Vol. 295. Toxicology Letters.
- Tay ZW, Chandrasekharan P, Zhou XY, Yu E, Zheng B, Conolly S. 2018. In vivo tracking and quantification of inhaled aerosol using magnetic particle imaging towards inhaled therapeutic monitoring. Theranostics. 8(13):3676–3687.
- Tsuda A, Henry FS, Butler JP. 2013. Particle transport and deposition: basic physics of particle kinetics. Compr Physiol. 3(4):1437–1471.
- U.S. DHHS. 2014. The health consequences of smoking – 50 years of progress: a report of the Surgeon General. Atlanta, GA: US Department of Health and Human Services, Centers for Disease Control and Prevention, National Center for Chronic Disease Prevention and Health Promotion, Office on Smoking and Health
- U.S.EPA. 1994. Methods for derivation of inhalation reference concentrations and application of inhalation dosimetry. EPA/600/8-90/066F October 1994.
- U.S.EPA. 2014. Guidance for Applying Quantitative Data to Develop Data‐Derived Extrapolation Factors for Interspecies and Intraspecies Extrapolation. EPA/R-14/002F September 2014.
- U.S.FDA. 2012. Guidance for Industry: Modified Risk Tobacco Product Applications. Draft Guidance. Washington, DC: US Government Printing Office.
- U.S.FDA. 2017. Philip Morris products SA modified risk tobacco product (MRTP) applications. https://wwwfdagov/TobaccoProducts/Labeling/MarketingandAdvertising/ucm546281htm
- Valdespino-Gomez JL, de Lourdes Garcia-Garcia M, Fernandez-de-Castro J, Henao-Restrepo AM, Bennett J, Sepulveda-Amor J. 2006. Measles aerosol vaccination. Curr Top Microbiol Immunol. 304:165–193.
- Vas CA, Yurteri CÜ, Dickens CJ, Prasad K. 2015. Development and Characterisation of a Smoking Behaviour Measurement System. Beiträge Zur Tabakforschung/Contributions to Tobacco Research. 26(5):219–231.
- Weibel ER. 1963a. Morphometry of the human lung. Berlin, Heidelberg: Springer. Chapter 10, Geometry and dimensions of airways of conductive and transitory zones; p. 110–135.
- Weibel ER. 1963b. Principles and methods for the morphometric study of the lung and other organs. Lab Invest. 12:131–155.
- Yeh HC, Schum GM, Duggan MT. 1979. Anatomic models of the tracheobronchial and pulmonary regions of the rat. Anat Rec. 195(3):483–492.
- Zhao P, Zhang L, Grillo J, Liu Q, Bullock J, Moon Y, Song P, Brar S, Madabushi R, Wu T, et al. 2011. Applications of physiologically based pharmacokinetic (PBPK) modeling and simulation during regulatory review. Clin Pharmacol Ther. 89(2):259–267.