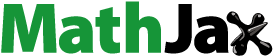
Abstract
Percutaneous occupational exposure to industrial toxicants can be assessed in vitro on excised human or animal skins. Numerous factors can significantly influence skin permeation of chemicals and the flux determination. Among them, the vehicle used to solubilize the solid substances is a tricky key step. A “realistic surrogate” that closely matches the exposure scenario is recommended in first intention. When direct transposition of occupational exposure conditions to in vitro experiments is impossible, it is recommended that the vehicle used does not affect the skin barrier (in particular in terms of structural integrity, composition, or enzymatic activity). Indeed, any such effect could alter the percutaneous absorption of substances in a number of ways, as we will see. Potential effects are described for five monophasic vehicles, including the three most frequently used: water, ethanol, acetone; and two that are more rarely used, but are realistic: artificial sebum and artificial sweat. Finally, we discuss a number of criteria to be verified and the associated tests that should be performed when choosing the most appropriate vehicle, keeping in mind that, in the context of occupational exposure, the scientific quality of the percutaneous absorption data provided, and how they are interpreted, may have long-range consequences. From the narrative review presented, we also identify and discuss important factors to consider in future updates of the OECD guidelines for in vitro skin absorption experiments.
Introduction
Occupational exposure to chemicals through the skin has become a veritable occupational health and regulatory issue, recognized as such in the United States (Anderson and Meade Citation2014) and in Europe (van Hemmen Citation2004). In occupational exposure assessments, it is therefore important to consider dermal absorption, which is a route of exposure in a number of workplaces. Percutaneous exposure to industrial toxicants can be assessed through in vitro experiments on excised human or animal skin sections. OECD guidelines (OECD Citation2004a, Citation2004b, Citation2011) have been published to help researchers to define experimental setups, and to set the various parameters for in vitro skin permeability experiments. However, these guidelines are considered too imprecise (Fabian et al. Citation2017; Esposto Biondo et al. Citation2021), and neither harmonize the experimental setups nor comprehensively address methodological issues (Sullivan et al. Citation2017; Hopf et al. Citation2020). Indeed, numerous factors can significantly influence how chemicals permeate the skin and the flux measured. Among them, selection of the vehicle used to solubilize the test substance is a delicate step, as the vehicle itself may affect the skin barrier, and consequently influence percutaneous absorption of substances in a variety of ways. If the question of the vehicle arises in this type of assay, it is generally because the substance to be tested is not a liquid. Homogeneous distribution of solid substances is effectively difficult to ensure without using a vehicle. In this narrative review, we have mainly focused on liquid monophasic vehicles. As recommended by the OECD guidelines, the vehicle should be a “realistic surrogate,” which means that for occupational dermal risk assessment purposes, it should closely match the exposure scenario. However, the exposure scenario is often complex, diverse, and difficult to mimic experimentally, making transposition of occupational exposure conditions to in vitro experiments non-trivial. Indeed, workers are for example rarely exposed to the neat chemical or to the substance dissolved in water.
We start our review by presenting some theoretical aspects of the skin permeation, we then address the main potential effects on percutaneous absorption of five vehicles, selected because they are the most commonly used (water, ethanol, and acetone) or the most realistic (artificial sebum and artificial sweat). We particularly emphasize the cutaneous barrier function. Finally, we present a number of criteria to help choose the most appropriate vehicle, and tests that should be carried out to guide refinement of this choice.
General information on the skin and skin absorption
The cutaneous barrier
The skin has a double protective function: it prevents loss of water and ions from the body, ensuring water homeostasis; but it also acts as a more or less effective barrier to the entry of xenobiotics into the body. The skin has a heterogeneous structural organization. It is made up of three main distinct layers, which are, from the outside to the inside: the epidermis, the dermis, and the hypodermis. All three layers are associated with numerous appendages, such as the sweat glands and pilosebaceous follicles. The dermis and hypodermis are connective tissues containing blood vessels (Prost-Squarcioni Citation2006; Geerligs Citation2009; Kendall et al. Citation2017), whereas the dermal-epidermal junction serves as a selective barrier controlling molecular and cellular exchanges between the two compartments (Geerligs Citation2009).
As shown in , the epidermis is a coating epithelium. The viable epidermis, with a thickness varying roughly from 30 to 100 µm, consists of the stratum basale, the stratum spinosum, and the stratum granulosum (SG). It is separated from the dermis by the basement membrane. The viable epidermis is innervated but not vascularized, and its nutritional needs are met through diffusion from the basement membrane. One of its functions is as a barrier against environmental aggression. The majority (80%) of epidermal cells are the keratinocytes; the other cell types found in the epidermis are melanocytes, Langerhans cells, and Merkel cells. Specialized junctions called corneodesmosomes or desmosomes (to which the tonofilaments are tethered) (Prost-Squarcioni Citation2006) link keratinocytes together and contribute to the outside-in epidermal barrier. The highly protective outermost layer of the epidermis – the stratum corneum (SC) – is constantly renewed. It is about 10–40 µm thick and composed of 15–25 layers of corneocytes, i.e. dead and keratinized cells resulting from the terminal differentiation of keratinocytes that have migrated to the skin’s surface.
Figure 1. Cell layers and cells making up the epidermis. Modified from Servier Medical Art, licensed under a Creative Commons Attribution 3.0 Generic License. http://smart.servier.com/.
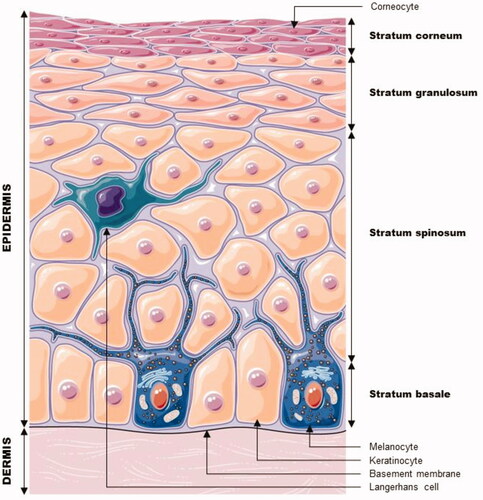
The SC is often compared to a brick-and-mortar structure with water- and protein-containing cells – the keratin and filaggrin-filled corneocytes – surrounded by a lipophilic cement covalently linked to proteins in the cells’ membranes ().
The extracellular matrix of the SC is composed of cholesterol, free fatty acids, and a large number of ceramide species () that are exported from the cells during cornification of the skin, and produced by transformation of the secreted lamellar body (LB) lipids or LB sheets (polar lipids precursors) (Coderch et al. Citation2003; Lafforgue Citation2008; Bolzinger et al. Citation2012). This extracellular matrix also contains aqueous pockets surrounded by polar lipids (Roberts et al. Citation2002; Wertz Citation2004).
The human SC is itself heterogeneous. The first layer is the compact, impermeable, lower SC, whereas the second corresponds to the upper desquamating layers. These upper layers are not impermeable as the desmosomal links between corneocytes are degraded. The SC contains a number of lipids, which have been described in terms of composition, lipid bilayer organization, physical state, and role (Elias Citation1983; Schurer and Elias Citation1991; Coderch et al. Citation2003; Bouwstra and Gooris Citation2010; Bolzinger et al. Citation2012; Menon et al. Citation2012). The mixture of lipids in the SC seems to play a major role maintaining the barrier properties of the epidermis (Coderch et al. Citation2003).
The SC is composed of 20% of water, which is present in several “states.” The natural moisturizing factor corresponds to 25–35% of the water content, it is considered to be “bound” to hygroscopic molecules (mixture of amino acids, amino acids derivatives, and salts resulting from the breakdown of filaggrin) (Verdier-Sévrain and Bonté Citation2007; Georgel Citation2008). Water in the SC is also hydrogen-bonded to the polar head-group regions of the lipid lamellae in intercellular spaces, and free water may be present between these lamellae, depending on the overall degree of hydration of the SC (Wertz Citation2004).
The specific barrier properties of the skin are mainly ensured by the SC, together with the seals between corneocytes/keratinocytes in the various layers, these seals correspond to tight junctions encircling the corneodesmosomes/desmosomes (Igawa et al. Citation2011; Crawford and Dagnino Citation2017). Tight junction proteins play an important role in preventing loss of water and solutes (Verdier-Sévrain and Bonté Citation2007).
Several methods exist to assess skin barrier integrity or to check whether a skin sample is diseased or damaged. No consensus barrier integrity test has been established, and methods have yet to be harmonized, therefore several methods are accepted. These include electrical resistance (RET) to an alternating current, Trans Epidermal Water Loss (TEWL), defined as the passage of water from the skin to the outside environment, and assessment of percutaneous tritiated water flux (USEPA Citation2004; OECD Citation2011; Guth et al. Citation2015). The specificities of each method are discussed in Guth et al. (Citation2015); Hopf et al. (Citation2020). For the dermatomed human skin commonly used in assays, the TEWL value for intact barrier is less than 10 g.m−2.h−1 (Guth et al. Citation2015).
Cutaneous absorption of a substance, pathways and mechanisms of interaction
The main steps of percutaneous absorption are illustrated in . In vivo, the SC is covered by a hydrolipidic film, consisting of water and fatty acids that is derived from sebaceous secretions (sebum), sweat, and breakdown of keratinocytes. The first step in diffusion through the skin thus requires the substance to dissolve in this film and diffuse through it (A) (). Once at the surface of the skin, the substance diffuses through the epidermis followed by the dermis. In the SC, two routes may coexist: molecules can diffuse via the extracellular cement (B) – this route can be taken by both hydrophilic and lipophilic substances (Trommer and Neubert Citation2006) – or by transcellular passage, also known as the lipid corneocyte pathway (C). In transcellular passage, the chemical traverses alternating layers of extracellular lipids and the aqueous phases of corneocytes (El Maghraby Citation2017), it is generally used by hydrophilic compounds. This “polar pathway” takes advantage of “defects” in the SC lipids and may involve the corneodesmosomes. In viable epidermis, transcellular diffusion (D) is considered a major pathway compared to the extracellular route (E) (Brown et al. Citation2016). Diffusion also occurs in the dermis until the substance comes into contact with the capillary vascular system, where it can be absorbed. In the hypodermis, and even through subcutaneous tissues, diffusion can also continue (Georgel Citation2008). In vivo, the dermis appears to play a negligible role in percutaneous absorption, since compounds rapidly enter the bloodstream (Hoang Citation1992). Nevertheless, this is a simplified picture, and Kretsos et al. (Citation2008) underline the need for further studies to clarify transport at the level of the dermis, for example considering protein binding and partitioning in dermal lipids. An alternative to extracellular and transcellular routes is the shunt pathways, whereby compounds exploit skin appendages such as hair follicles or glands (F). Passage via with these routes generally occurs during the initial transient phase of absorption, and continues for the duration of contact, it is favored by highly hydrophilic compounds or larger molecules that would otherwise have difficulty penetrating the skin (Kasting et al. Citation2005). In terms of chemical characteristics, the content of the eccrine sweat gland is mainly hydrophilic, whereas the follicular duct produces a lipophilic secretion due to the presence of sebum. However, the orifices of sweat ducts, with their much smaller dimensions, are believed to play a minor role in substance absorption, and it is generally assumed that the main shunts are hair follicles (Meidan et al. Citation2005).
Figure 3. Major percutaneous absorption routes (according to Brown et al. (Citation2016)).
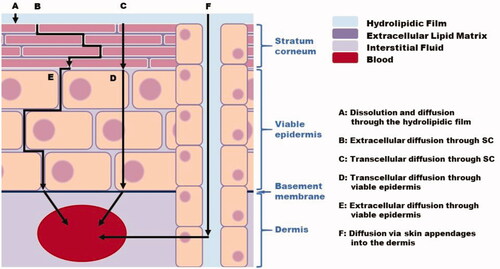
Percutaneous absorption can be experimentally assessed in vitro, using static vertical Franz diffusion cells (Franz Citation1975). These devices are composed of a donor and a receptor compartment, containing a receptor fluid. The skin sample is placed between the two compartments as presented in .
The substance is applied to the skin sample in the donor compartment, either directly or after dissolving it in a vehicle. Only solubilized substances can diffuse within the vehicle. Because of pretreatments applied to the skin (e.g. human skin preparation before surgery), by extrapolation from the work of Rode et al. (Citation2000), the thin hydrolipidic layer may well be absent in vitro. Once at the interface of the SC, test substances can partition between the vehicle and the skin. This process is governed by the partition coefficient of the substance, Ksv (SC/vehicle partition coefficient). When using a volatile vehicle, especially when a small volume is applied, only a minute amount of substance can partition before the vehicle evaporates (Gajjar and Kasting Citation2014).
Because the SC (or total epidermis) is considered to be the rate-limiting barrier to diffusion for most compounds, split-thickness skin samples are generally used for in vitro tests (Hoang Citation1992). However, readers should be wary of excision and/or splitting of the skin with a dermatome for in vitro experiments as these techniques may introduce bias in the follicular pathway compared to in vivo studies (Meidan et al. Citation2005). Indeed, Patzelt et al. (Citation2008) observed a reduced follicular reservoir in skin models used in vitro, probably due to contraction of the elastic fibers around hair follicles when skin is excised.
The preliminary stage of release (or partitioning) of a molecule depends on its relative affinity for the SC and the vehicle, characterized by Ksv (Idson Citation1983), which corresponds to the equilibrium partition coefficient for distribution of a substance. For an ideal solution, this parameter is defined as:
(1)
(1)
where
is the concentration of the solute in the SC, and
its concentration in the vehicle.
This equilibrium partition coefficient is influenced by thermodynamic effects (Azzi et al. Citation2005). Thus, a lipophilic substance dissolved in a polar vehicle, such as water, will have a high affinity for the SC (high Ksv). Conversely, a lipophilic substance dissolved in a non-polar vehicle will have a lower affinity for the SC (low Ksv).
Once in the SC, a substance can diffuse passively within each layer of the skin. However, between the SC and the hydrophilic layers of viable epidermis/dermis another partition step takes place. In addition, the substance applied can partition into the lipid or/and protein domains of the SC (), with more polar and hydrophilic molecules entering the protein domain, and more lipophilic molecules entering the lipid domain (Hui et al. Citation2005).
The concentration of the molecules applied decreases in a linear fashion between the surface and the deepest layers. The ease with which a molecule moves through the various membrane strata is reflected by the diffusion coefficient, D. This factor, also called “apparent” (overall) diffusivity, is usually estimated from experimental data or predicted by models (Atobe et al. Citation2015; Rothe et al. Citation2017). According to the rule of thumb “Lipinski's rule of five,” substances that penetrate best are soluble in both lipids and water. Compounds that are soluble in only one or the other are not considered good penetrants. This explains why solutes with a balanced partition coefficient – log Kow between 1 and 3 – are associated with optimum skin permeation.
In addition to the phenomenon of passive diffusion, substances can be actively transported through the skin by protein transporters. Active transport has been a topic of interest, although most of the studies we identified sought to identify these transporters (mostly at the RNA level), rather than to study their influence on percutaneous absorption of substances (Dancik et al. Citation2008; Osman-Ponchet et al. Citation2017).
According to the infinite dosing scenario (no depletion of the substance in the donor compartment whatever the vehicle’s volatility and the molecule’s flux), the flux of a substance at steady-state (Jss) through a homogenous membrane of thickness d, is determined by Fick’s first law as:
(2)
(2)
where
is the concentration difference for the substance on either side of the membrane. EquationEquation (2)
(2)
(2) assumes ideal solutions, as the concentration gradient is used as an approximation of the thermodynamic activity gradient.
This equation can be used to describe transport of a molecule by passive diffusion through the skin under steady-state conditions, assuming that the skin behaves like a pseudo-homogeneous membrane and that its barrier properties are stable over time and position (Brown et al. Citation2016). EquationEquation (2)(2)
(2) applied to the skin is frequently expressed as:
(3)
(3)
where A is the surface area of the exposed skin,
is the concentration gradient between the two sides of the barrier, d is either the thickness of the SC or the diffusion path length, and
is the dermal permeability coefficient.
The dermal permeability coefficient relates to the partitioning and diffusion behavior of the permeant and can be defined as (Idson Citation1983):
(4)
(4)
Theoretically, depends only on the membrane and the substance, and for a given substance,
is independent of the dose applied, rather it depends on the vehicle.
illustrates determination of Jss from in vitro experimental data obtained using Franz cells. Since in vivo an absorbed substance rapidly enters the bloodstream (with the body considered as a sink), and in vitro in infinite dose configurations, the donor concentration far exceeds the receptor concentrations, is often replaced by the concentration of the substance in the vehicle (Cv) (donor concentration).
Figure 5. Theoretical curve showing the accumulation of a substance in the receptor compartment per cm2 of skin over time (permeation profile for an infinite dose regimen).
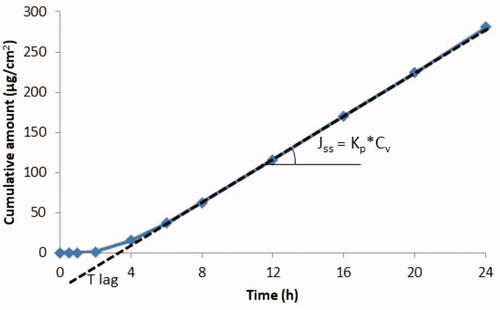
Note that, when the substance is applied in pure form (neat liquid) or at its saturated concentration in the vehicle (
values – also defined as Jmax – should theoretically be identical whatever the vehicle used. However, the barrier properties of the skin must not be directly altered by the vehicle or the substance. If the vehicle does not affect the skin, D and
(the solubility of the substance in the skin) remain unchanged (Roberts et al. Citation2002). When
increases, it is compensated by a decrease of
(cf. EquationEquations (5)
(5)
(5) and (Equation6
(6)
(6) )), resulting in a constant flux.
Jmax is defined by:
(5)
(5)
and EquationEquation (1)
(1)
(1) becomes:
(6)
(6)
Permeation through the epidermal barrier is thus affected by the partition coefficient between the vehicle and the skin (), a thermodynamic effect, and the diffusion or diffusivity of the permeant (D), which is a kinetic effect.
However, it is important to keep in mind that numerous assumptions are made that are not entirely exact: e.g. in reality the SC is structurally heterogeneous, and binding or skin-altering phenomena occur. Consequently, despite its mathematical simplicity, Fick’s law (EquationEquation (2)(2)
(2) and (Equation3
(3)
(3) )) does not accurately represent diffusion in the SC. In addition, the phenomena and mechanisms mentioned above only refer to the parent substances (in unaltered form), completely neglecting metabolic processes occurring in the skin, which could also modify how a substance is absorbed (Pannatier et al. Citation1978; Hoang Citation1992; OECD Citation2011; Bourgart Citation2019). Indeed, all the major metabolic enzymes found in the liver and other tissues have also been identified in the skin. The skin’s greatest metabolic capability is located in the epidermal layer, the pilosebaceous glands, and the upper dermis. Except for certain enzymes – such as the esterases – metabolic processes can only be investigated with viable excised skin. In these conditions, Eilstein et al. (Citation2014); Manevski et al. (Citation2015) characterized the metabolic activity of excised human skin, and reported a high esterase activity (phase I metabolizing enzyme) along with a number of phase II metabolizing enzymatic activities. These enzymes generally process lipophilic compounds to make them more hydrophilic, which may help them to penetrate the skin. Application of some substances may also induce or inhibit enzymes present in the skin (Bashir and Maibach Citation2005).
Potential effects of vehicles on the skin
When attempting to determine dermal absorption of a substance in vitro, the vehicle used is one of the critical parameters. It determines the Ksv and may affect the skin barrier and consequently the diffusivity D (Riviere Citation2005), to promote skin absorption (enhancer), or on the contrary, delay it.
The pharmaceutical and cosmetic industries have been developing vehicle formulations for several decades, generally as a medium for drug delivery, to enhance passage of substances through the skin. They define a vehicle as the sum of ingredients in which the drug is delivered to the skin. Thus, the vehicle may contain chemical penetration enhancers without pharmacological activity (e.g. solvents, surfactants, alkalis, strong acids, oleic acid, or azone (Trommer and Neubert Citation2006; Lane Citation2013; Abd et al. Citation2019)). An enhancer promotes drug transport across the skin barrier through numerous mechanisms, such as altering partitioning and/or diffusion, by interacting with the drug and/or the skin’s constituents. In fact, the role of an enhancer is to improve the delivery of drugs that otherwise would have difficulty reaching the deep skin layers (for topical/transdermal formulations), and ultimately the circulation (transdermal formulations). Increased delivery appears to be linked to modification of thermodynamic (partition) rather than kinetic (diffusion) properties (Rosado et al. Citation2003). The OECD guidelines define a penetration enhancer as “an adjuvant, which facilitates penetration of the test substance through skin” (OECD Citation2004a). Substance-vehicle-skin interactions may be explained by the “push-pull” effect (Haque and Talukder Citation2018): “push” resulting from the activity or excess free energy of the substance in the donor phase, and “pull” observed when the solvent permeating through the skin ‘drags’ the permeant with it, as represented in .
The numerous studies performed in dermatology or pharmacology on enhancers and their effects on the skin’s barrier properties can be very useful in guiding selection of a vehicle suitable for toxicological approaches. A major difference between the fields of dermatology/pharmacology and occupational exposure assessment is the limited number of vehicles suitable for use in the latter. Indeed, the test substance must be used in the same “formulation” as it is used in the workplace to reproduce the real exposure conditions. Experimentally, these can be tricky to accurately reproduce, and a surrogate vehicle must be selected which does not alter or damage the skin’s barrier properties, to avoid modifying absorption of the test substance.
Effects on the SC
Hydration/dehydration/occlusion
In vivo, the water content of naturally hydrated skin is around 30–50% (expressed as a water:protein mass ratio in the tissue; in grams of water per 100 g wet tissue) (Caspers et al. Citation2001). Its distribution differs in the viable epidermis and in the SC, at 70% and 15–30%, respectively (Verdier-Sévrain and Bonté Citation2007). This distribution causes water to diffuse from the deeper layers of the epidermis toward the surface of the skin.
Increased SC hydration can be encountered in several cases, firstly when water, an aqueous solution, or any other moisturizing substance is used as a vehicle (depending on the volume used), and secondly in case of occlusion. In vitro, occlusion includes use of a transdermal device (e.g. parafilm or screw cap) to cover the top of the donor compartment, generally to prevent loss of the substance or vehicle through evaporation; it may also be due to an effect of the vehicle itself (e.g. greases or oils). Occlusion increases the relative humidity close to the skin and the skin’s water content, thus increasing the amount of free water present (Lockley et al. Citation2004; Akhter and Barry Citation2011). Several other types of vehicle can also modify SC hydration.
A number of authors have investigated the effect of SC hydration on its structure and permeability. To do so, they varied the SC hydration level between 6% w/w (almost dry SC) and 60% (Barry Citation1987; Bouwstra et al. Citation1991, Citation1992) under conditions that were very different to those of percutaneous absorption experiments. With hydration levels up to 40% w/w, no lipid disorders – such as modification of the lateral packing of alkyl chains – or swelling of the bilayers were observed (Bouwstra et al. Citation1991; Mak et al. Citation1991; Bouwstra et al. Citation1992). However, at higher hydration levels (60% w/w), the lipids became disordered, resulting in a more fluid lipid structure (Barry Citation1987; Bouwstra et al. Citation1991). Freeze-fracture electron microscopy experiments performed on “fully hydrated” human SC revealed structures with a vesicle-like appearance, rough structures, and water pools present as a separate phase in the head-group regions of the intercellular lipids, with an increase in the mobility of the chains (Van Hal et al. Citation1996).
The proteinaceous regions of the SC took up most of the water (Barry Citation1987; Bouwstra et al. Citation1991; Haque and Talukder Citation2018), reducing diffusional resistance for intracellular content. In support of this observation, Van Hal et al. (Citation1996) reported that water binds to keratin filaments, which, in hydrated corneocytes, would lead to a change in the conformation of this protein (Lee et al. Citation2018) as water starts competing for the hydrogen binding sites on the proteins. This effect causes the corneocytes to swell (Van Hal et al. Citation1996), resulting in thickening of the SC (d in EquationEquation (2)(2)
(2) ). At the same time, permeation of molecules via the transcellular pathway increases (Haque and Talukder Citation2018). Thus, extensive hydration not only results in an increase in the value of the diffusion constant D, it also acts as a solvent for hydrophilic molecules, improving their solubility in the SC, and modifying their partition coefficient. Consequently, the presence of water decreases resistance of the skin barrier compared to dry skin. However, it is difficult to extrapolate these effects – observed following soaking the skin or in high humidity conditions – to the usual conditions of deposit of aqueous solution in in vitro percutaneous absorption experiments. In an attempt to elucidate the differences, Kaushik and Michniak-Kohn (Citation2010) deposited 50 µL of water to cover the entire surface of the SC on a skin sample placed in a Franz cell, and compared the vehicle-induced effects with those obtained with an untreated SC after incubation for 30 h. Their results suggest water-treatment leads to SC lipid disruption and extraction, as well as fluidization of lipid bilayers. Strong hydrogen bonding interactions between ceramides within the SC were also observed.
At the other extreme, some solvents (e.g. pure ethanol or higher alcohols, glycol ethers) and amphiphilic substances can dehydrate the SC, or at least its outer portion (Berner et al. Citation1989). Dehydration causes intercellular SC lipids to organize in a vertical compressed arrangement (dense, compact structure), and decreases the proportion of free mobile water as well as the volume of the aqueous lacunar domains (pores) between the lipids. Dehydration reduces SC permeability by increasing the efficiency of the barrier properties (Berner et al. Citation1989; Iwai et al. Citation2013; Frasch et al. Citation2014).
Modification of intercellular lipids/extraction of the lipid fraction
The intercellular lipid domains of SC constitute a major transport pathway for penetration of exogenous compounds. Thus, perturbation of the lamellar lipids lowers the skin’s resistance to permeation. Moreover, the presence of lipids is essential to the structural integrity of the protein-lipid matrix. Without lipids, with altered lipids, the skin is a fairly porous, nonselective membrane allowing low-energy diffusion pathways. Another consequence of lipid modifications is that they alter the tissue’s capacity to bind large amounts of water (Scheuplein and Ross Citation1970).
Solvents can mix with SC lipids – disrupting their organization, or even extracting some – which modifies both the overall lipid content of the SC and the lipid composition of the skin (Williams and Barry Citation2012).
Haque and Talukder (Citation2018) summarized the effects of some chemical enhancers and listed the main substances affecting lipids. Among them, due to their effects on lipids, some can completely disrupt the skin barrier, inducing a variety of biological responses (Grubauer et al. Citation1989; Denda et al. Citation1996).
For example, ethanol and other substances, were found to generate concentration-dependent effects: at low concentrations, “only” the lipid head groups may be disrupted; whereas at high concentrations, certain lipids may be extracted (Suhonen et al. Citation1999; Kwak et al. Citation2012). Acetone can be used to deliberately disrupt the skin barrier (e.g. when seeking to reproduce skin diseases), and is known to induce structural defects in the SC by reducing its lipid content and altering the structure of the intercellular lipid bilayers. This treatment thus enhances the skin’s permeability to both hydrophilic and amphipathic substances. The effects of ethanol and acetone on the skin’s barrier function are further detailed below. Other aprotic polar solvents such as dimethylsulfoxide (DMSO) can extract some lipids, causing irreversible damage (Scheuplein and Ross Citation1970), and increasing penetration of hydrophilic and lipophilic permeants. These effects are concentration-dependent, but have been observed with concentrations above 60% DMSO (Williams and Barry Citation2012).
Effects on lipids are not restricted to organic solvents. For example, anionic surfactants including sodium dodecyl sulfate (SDS), sodium lauryl sulfate (SLS), and linear alkyl benzene sulfonate (LAS) cause various degrees of lipid disordering (Froebe et al. Citation1990; Ribaud et al. Citation1994; Moghadam et al. Citation2013; Barba et al. Citation2015). In addition to alterations caused by partitioning of the surfactant in SC lipid bilayers (Downing et al. Citation1993; Ananthapadmanabhan et al. Citation2004), surfactants could deplete certain SC lipids (Froebe et al. Citation1990; Rawlings et al. Citation1994; Bolzinger et al. Citation2012). Concentration-dependent effects were reported for SLS and LAS, with increasing amounts of lipids removed when the concentration applied exceeded its critical micelle concentration (0.24 and 0.04%, respectively). An effect of time was also reported, with lipid removal by SLS increasing for incubation times between 30 s and 10 min (Froebe et al. Citation1990). Some authors also suggest that, rather than damaging the skin by delipidation, SDS might alter the synthesis of new lipids (Fartasch Citation1997; Ananthapadmanabhan et al. Citation2004).
In studies with large molecules (1 kDa or more), cutaneous absorption is generally relatively low. Some vehicles (e.g. solvents and surfactants) may increase chemical-induced lipid disorders, but do not necessarily correlate with increased absorption as the vehicles applied may decrease the drug’s solubility in the SC (Moghadam et al. Citation2013; Moghimipour et al. Citation2013).
Interaction with intracellular keratins
In addition to effects on water and lipid content, vehicles can also denature intracellular keratin or modify its conformation in the SC, leading to swelling and increased hydration. As previously explained for hydration of the SC, the proteinaceous intracellular region takes up water. At high water levels, the proteins become disordered and water starts competing for hydrogen binding sites on the proteins, decreasing interactions between them (Barry Citation1987). Water is not the only chemical enhancer that interacts with keratin fibrils. Aprotic solvents such as DMSO and N-methyl-2-pyrrolidone (NMP), some glycols like propylene glycol (PG) and polyethylene glycol (PEG), or anionic surfactants such as SLS also interact with keratin, binding to its positively charged and hydrophobic sites (Morris et al. Citation2019). This binding potentially disrupts its ordered arrangement (Barry Citation1987; Moghimipour et al. Citation2013; Salimi et al. Citation2015; Haque and Talukder Citation2018). DMSO interacts with keratins even when present at low concentrations (20%). In addition, SLS causes the alpha keratin filaments to uncoil – denaturing them to produce beta keratin – and thus opens up the polar pathway. As a result, the presence of anionic surfactants in the vehicle enhances water-absorption by the skin. The degree of enhancement depends on the surfactant (Morris et al. Citation2019).
Accumulation of vehicle in the SC or vehicle uptake into the SC
Water accumulation in the SC and its effects are described above. Although little documented, it has been suggested that other solvents or vehicles permeating the skin could “drag” a permeant with them thanks to a “pull” mechanism, as explained by Kadir et al. (Citation1987); Haque and Talukder (Citation2018) ().
A vehicle with good solubilizing properties that remains in the skin during the experiment will allow the chemical to be absorbed (Lane et al. Citation2012). For example, Zhang et al. (Citation2011) observed high uptake of vehicle into the SC, which promoted partitioning of the substance into the SC and changed its solubility in this compartment, possibly because the substance is present within the vehicle fraction inside the skin. However, comparing the effect of different 5% w/v formulations of niacinamide applied to skin for 48 h, Mohammed et al. (Citation2014) observed that a high solvent uptake did not systematically result in high flux. In both these percutaneous absorption studies, relatively large volumes were deposited on the skin −2.3 ml/cm2 (Zhang et al. Citation2011) and 1 ml/cm2 (Mohammed et al. Citation2014) – which could facilitate solvent uptake.
With lower volumes, or if the solvent permeates rapidly into and across the skin, its depletion from the formulation, and ultimately from the skin, could lead to crystallization of the test substance, thus affecting dermal absorption.
pH effects
If the pH of the vehicle differs from the pH of the skin, the level of ionization of the compound may be modified. According to the pH-partition hypothesis, non-ionized compounds generally pass more easily through lipid membranes, the predominant route for permeation through the SC, compared to ionized compounds. However, ionized molecules partially contribute to percutaneous absorption via follicular shunt pathways. Some recent studies investigated the effects of pH on absorption (Mutalik et al. Citation2014). The in vitro permeation profile of two non-ionized lipophilic substances, hydrocortisone and testosterone, was not altered by changes to the pH of the solution applied (range 1–11), indicating that the lipoidal route of penetration is not modified below a pH of 11. Conversely, increased penetration has been reported for some compounds at pH 2 or 10, possibly due to intracellular changes at these pH (Sznitowska et al. Citation2001). Whatever the case, unless they correspond to the exposure scenario, extreme pH values must be avoided in vehicles due to their potential corrosive/irritant effects that would lead to destruction of the SC barrier. A pH effect (between pH 3 and 8) on skin permeability was described by Martínez-Pla et al. (Citation2004) for a large proportion of a panel of 12 non-steroidal anti-inflammatory drugs. To measure this effect, the authors used a biopartitioning micellar chromatography retention–permeability model. Variations in skin permeability appear to be linked to ionization of the drug rather than to modification of the skin barrier. However, further studies will be required to confirm this conclusion.
Effects on the viable epidermis
Although the SC is the main barrier to permeation of chemicals, the underlying viable epidermis and dermis may also contribute to strengthening the skin’s barrier function (Andrews et al. Citation2013). As a penetrant moves through the epidermis, it crosses cell membranes and diffuses through cells. In the epidermis, the substance may become separated from vehicle components. However, remaining components can continue to interact and enhance the absorption rate by increasing partitioning into cell membranes or by increasing the fluidity of membranes. Moreover, these components can influence the enzymatic activity with respect to the substance in the epidermis through inhibition or downregulation (Chittenden and Riviere Citation2017). For example, ethanol was shown to inhibit CYP1A1 and CYP2B1/2 in rat skin (Lee et al. Citation2001).
Effects on hair follicles
The presence of sebum in the follicular canal may restrict or enhance the transport of molecules into the follicle, depending on the physicochemical characteristics of the vehicle and the substance. Dissolving the substance of interest in a lipid-solubilizing solvent, such as ethanol or acetone, may solubilize sebum, making it possible for substances to be deposited within the follicle (Meidan et al. Citation2005). An example of this effect was presented by Grice et al. (Citation2010), who noted that ethanol or water/ethanol mixtures promoted the accumulation of minoxidil, a therapeutic agent, in the appendages. In addition, wetting agents decrease the interfacial tension between polar substances and sebum, which may promote mixing in the form of a surfactant/sebum emulsion. Any such mixing will favor partitioning and absorption of the substance (Lauer Citation2005).
Various water-in-oil-emulsion systems have been investigated as follicle-targeting vehicles. In a study with normal and appendage-free rat skins, Bamba and Wepierre (Citation1993) studied the transappendageal pathway for pyridostigmine 5% in various vehicles (315 µL/cm2 applied under occlusion). Their results indicated that this pathway was vehicle-dependent, and that it was maximized with ethanol, DMSO in ethanol, or PG in ethanol. These are all good solvents for sebum, allowing the test substance to mix with it and penetrate the dermis. With ethanol, a difference in flux between the two kinds of skin was observed from 8 h. However, whether this effect would have been observed in more usual deposit conditions – with lower concentrations and smaller volumes, and without occlusion – remains unknown. In terms of transcellular passage, PG alone is a poor enhancer, and consequently the follicular pathway is even more relevant than with the two first vehicles.
The effects these solvents can have on the integrity of the barrier (as mentioned above) depend on the experimental conditions: volume or quantity deposited, dilution of the vehicle in case of solvent, duration of exposure, etc. The experimental conditions reported throughout the literature are sometimes very far removed from those advocated by the guidelines (OECD Citation2004a, Citation2004b, Citation2011).
Vehicles used to mimic occupational exposure
A very large number of vehicles has been used to study the percutaneous absorption of substances – such as drugs or chemicals – encountered in workplaces. From information in the EDETOX database and the literature, Samaras et al. (Citation2012) identified 536 reports on human skin flux. After excluding experiments performed with drugs from this dataset 455 reports remained, using over 180 different vehicle formulations. Among these, water was by far the most frequently used vehicle, and the large majority were classed in just eight formulation categories. In nearly 70% of cases, the vehicle used was water (or saline), acetone, or ethanol (very often mixed with water or saline) ().
Figure 7. Vehicles used in percutaneous absorption experiments after exclusion of experiments with drugs (according to Samaras et al. (Citation2012) data). The exact number of experiments in which each vehicle is used is given.
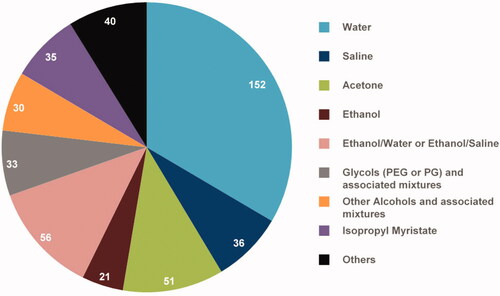
Because water, ethanol, and acetone are the most used, for this narrative review we attempted to take an inventory of studies using these vehicles. In addition, we chose to evaluate the advantages of two more recently developed vehicles, namely artificial sebum and sweat, as they are used to mimic the skin’s natural secretions.
Water
As indicated above, water is widely used as a vehicle for in vitro studies, either in its pure form or as part of formulations with surfactants (Abdallah et al. Citation2015, Citation2016), various PEG, ethanol or other compounds (Hatanaka et al. Citation1993; Abd et al. Citation2019). Here, we will mainly focus on the effects of pure liquid water. Water and aqueous solutions are often considered to be inert with respect to the skin membrane, which is why it is often used as a control vehicle, or even as the only vehicle (particularly for drugs or cosmetics). Indeed, water is a common ingredient in topical and transdermal formulations. However, water is reported to be a potent penetration enhancer (Gwak et al. Citation2004) due to its skin hydrating effect, and is even listed as the most common and safe means to transport drugs and cosmetics into the skin (Haque and Talukder Citation2018). It is important to keep in mind that the degree of hydration of the skin has a major effect on the percutaneous absorption of most substances. Flux was compared for diverse compounds in a range of vehicles, e.g. water vs. diverse water/ethanol formulations for nitroglycerin (Berner et al. Citation1989); water vs. acetone or sebum for the lipophilic molecules bisphenol A and bisphenol S (Champmartin et al. Citation2020); water vs. isopropyl myristate, Tween 80, binary formulations of both vehicles, or ternary formulations including water for several alcohols (Garcia et al. Citation1980). However, in such conditions, it remains difficult to conclude on the enhancement effect of water insofar as there is no consensus on a control or reference vehicle, and in the absence of comparisons involving application of solid test substances in the form of powders.
The literature highlights specific behaviors for some compounds applied in water. In solutions of liquid amphiphilic substances such as butoxyethanol (BE), with a BE weight fraction (wBE) of less than 0.8, water activity is almost 1, and the skin is fully hydrated. In these conditions, the flux of BE is proportional to its thermodynamic activity. With more concentrated solutions – wBE ranging from 0.8 to 1 – the activity of water in the solution drops from 0.9 to 0 with neat BE. As the water activity of hydrated skin exceeds 0.8, the driving force will cause water to diffuse from the skin to the vehicle, decreasing the BE flux as the skin becomes dehydrated. This effect explains the reduced flux observed with more concentrated BE solutions. The impact of the proportion of water applied on skin hydration and on flux, observed and explained for BE (Korinth et al. Citation2007; Traynor et al. Citation2007; Bunge et al. Citation2012) and 2-hydroxypropyl acrylate (Frasch et al. Citation2014), has also been observed with other glycol ethers (Venier et al. Citation2004; Traynor et al. Citation2007), ethanol (Berner et al. Citation1989), N-vinylpyrrolidone (NVP) (Marquet et al. Citation2015), nicotine (Kuswahyuning and Roberts Citation2014), and a number of amphiphilic compounds (Korinth et al. Citation2012). Absorption of these substances through the skin is clearly promoted by the presence of water in the vehicle. In contrast, the proportion of water has a completely different effect on the percutaneous absorption of dimethylformamide, which is itself an enhancer, and is unlikely to have a dehydrating effect on the skin (Wang et al. Citation2009).
Ethanol
Ethanol is a volatile solvent. Its use as a vehicle for percutaneous absorption of drugs or cosmetics is often justified by its solubilization power (in particular for lipophilic compounds). Its chemical enhancer properties are exploited in topical and transdermal pharmaceutical formulations (Berner et al. Citation1989; Abd et al. Citation2019). Whereas its well-established low systemic toxicology and local tolerability explain its frequent use in cosmetic and personal care products. Nevertheless, ethanol-free formulations are recommended for sensitive skin. We will only discuss the effects of neat ethanol (as an applied substance or as a vehicle) and binary ethanol-water systems here.
Thomas and Panchagnula (Citation2003) investigated the effect of ethanol on the percutaneous absorption of the hydrophilic molecule zidovudine (AZT) by varying the proportions of ethanol and water in the binary vehicle. Up to an ethanol volume fraction (Ve) of 0.666, AZT absorption increased; whereas higher proportions of ethanol (100%) decreased its permeation. Similar results have also been reported for other lipophilic molecules applied at an infinite dose: nitroglycerin (Berner et al. Citation1989), N,N-diethyl-m-toluamide (Qiu et al. Citation1998), salicylate (Kurihara-Bergstrom et al. Citation1990), ibuprofen (Watkinson et al. Citation2009). A high Ve leads to SC alteration and dehydration of the outer portion of the skin (Berner et al. Citation1989; Qiu et al. Citation1998; Levang et al. Citation1999; Thomas and Panchagnula Citation2003; Watkinson et al. Citation2009). Consequently, the barrier property increases, blocking ethanol permeation (Ve between 0.7 and 1) (Berner et al. Citation1989). Watkinson et al. (Citation2009) observed skin dehydration and shrinking upon exposure to pure ethanol. In contrast, when ethanol and water are used as cosolvents, the skin was observed to swell (Berner et al. Citation1989).
Several publications highlighted the effect of ethanol on skin lipids due to its lipid extraction properties (Kurihara-Bergstrom et al. Citation1990; Bommannan et al. Citation1991; Levang et al. Citation1999; Suhonen et al. Citation1999; Zhang et al. Citation2017), which could lead to the localized formation of hydrophilic pores/domains within the lipid domain at high ethanol concentrations, potentially resulting in reorganization of lipid polar head regions (Kurihara-Bergstrom et al. Citation1990; Suhonen et al. Citation1999). However, based on small- and wide-angle X-ray scattering analysis, Moghadam et al. (Citation2013) reported no spatial disordering of lipids within bilayers. Lipid fluidization is sometimes mentioned, but appears to be controversial. Indeed, some publications indicate, rather, rigidification of the lipid bilayers (Grubauer et al. Citation1989; Levang et al. Citation1999; Thomas and Panchagnula Citation2003; Lane Citation2013). Still other publications reported an effect of ethanol on skin proteins, extracting them from the SC, thus increasing the skin’s porosity. Protein extraction was associated with secondary conformational alterations to keratinized protein fibrils. The conformational shift from α-helix to β-sheet is associated with swelling of the protein matrix (Kurihara-Bergstrom et al. Citation1990; Suhonen et al. Citation1999). The β-sheet-like conformational change to SC proteins was reported to be reversed by hydration (Suhonen et al. Citation1999). Based on all these observations, Zhang et al. (Citation2017) hypothesized that the enhancement effect of ethanol on the penetration of lipophilic compounds could be explained by an increase in its solubility in the SC lipid domain (transcellular route), whereas penetration of hydrophilic drugs could be enhanced by the increased porosity of the SC as a result of the extraction of lipids or proteins (Trommer and Neubert Citation2006). Interestingly, Bamba and Wepierre (Citation1993) highlighted the effects of ethanol acting as a solvent for sebum, allowing the transport of the hydrophilic compound pyridostigmine bromide via the appendageal pathway. Application of pure ethanol leads to rapid evaporation, which, in the absence of occlusion, has a significant impact both on partitioning of the test substance in the upper SC layers and on its diffusion through the lower skin layers to gain access to the blood, or the receptor fluid in in vitro experiments. Indeed, evaporation leaves the substance in a more thermodynamically active state than when the solvent was present, potentially resulting in supersaturation, which pushes the substance into or across the SC. Consequently, a concentration gradient of solute is established in the skin, and a greater driving force is created for diffusion of the substance. After complete dissipation of the solvent, a certain amount of the substance will be present in the SC, with the remainder precipitated on the skin’s surface, from where it can be slowly, and to a lesser degree, absorbed into the SC (Intarakumhaeng and Li Citation2014). As it rapidly permeates the skin, ethanol modifies the skin’s structure and promotes solubility of the solute. In certain conditions, this scenario creates localized areas containing high concentrations of the test substance (Dias et al. Citation2007). Ethanol can thus pull or drive the substance along with it as it interacts with the skin. Thus, for some substances, flux across the skin was found to correlate in a linear fashion with ethanol flux (Berner et al. Citation1989). Once the ethanol has evaporated and/or passed through the skin, it no longer exerts any effect on transport of the substance (Lane Citation2013).
When ethanol evaporates very rapidly (e.g. 10 µL/cm2 applied), the test substances precipitate at the surface of the skin (Hewitt et al. Citation2020). These experimental conditions may be particularly relevant when assessing exposure to toxicants in solid form, especially since rapid evaporation (within minutes) limits alterations of the skin by the ethanol (Intarakumhaeng and Li Citation2014; Intarakumhaeng et al. Citation2018).
In addition to its effects on skin barrier integrity, ethanol could affect the skin’s viability and the enzymes present, potentially further modifying the percutaneous absorption of some substances, in particular substances that can be metabolized. Lee et al. (Citation2001) tested the effects of ethanol in vivo on rat skin, and showed altered messenger RNA expression levels for some skin cytochrome P450 (CYP) isoforms, with ethanol inhibiting expression of two CYP isoforms. Like other alcohols, ethanol is a substrate for alcohol dehydrogenase (ADH), which is present in the skin of various mammalian species. As such, ethanol will have an enzymatic induction effect (Oesch et al. Citation2007, Citation2018). Liu et al. (Citation1991) directly assessed the effect of ethanol on β-oestradiol metabolism by measuring flux of the parent compound and of its metabolite, estrone, through full-thickness hairless mouse skin. Even at low concentrations (2%), ethanol had an inhibitory effect on the β-estradiol to estrone reaction. Similarly, Oh et al. (Citation2002) studied the metabolism of methyl p-hydroxybenzoate (methylparaben) in skin excised from Yucatan micropigs. In experiments with skin homogenates (without determining which specific enzymes were involved), they reported that, from 1%, ethanol inhibited p-hydroxybenzoic acid hydrolysis, but promoted its transesterification to produce ethyl p-hydroxybenzoate. In the absence of ethanol, only hydrolysis, leading to production of p-hydroxybenzoic acid, was observed. In both human and fuzzy rat skin, Yourick and Bronaugh (Citation2000) reported altered metabolism of 2-Nitro-p-phenylenediamine (2-NPPD) – a chemical used in hair dye formulations –. In rat skin permeation studies, analysis of the receptor fluid showed that more (85%) 2-NPPD was metabolized in the presence of ethanol than with a semi-permanent formulation containing two other dye components (47%).
Acetone
Acetone is often used for its ability to solubilize highly lipophilic substances or to mimic exposure to a pure solid deposit (uniform application of drugs or chemicals) (Feldmann and Maibach Citation1969; Scheuplein and Ross Citation1974; Stinchcomb et al. Citation1999; Akhter and Barry Citation2011; Champmartin et al. Citation2020). Some authors justify the use of acetone as a vehicle by its minimal effect on skin barrier function, with reference to a small number of relatively old publications (Feldmann and Maibach Citation1969, Citation1970; Feldman and Maibach Citation1974; Abrams et al. Citation1993). However, these studies should be considered with care as, for example, Abrams et al. (Citation1993) concluded on an absence of lipid extraction based on short-duration (<12 min) applications of 500 µL/cm2 acetone to human skin in vitro, and Feldmann and Maibach (Citation1969); Feldman and Maibach (Citation1974) assumed the absence of effect on the skin was related to the short contact time (<15 s), with evaporation accelerated by blowing.
Indeed, in most publications mentioning acetone, the reason for its use is mainly as a means to disrupt the skin barrier (through delipidation) in order to mimic damaged skin and thereby increase its permeability (Abrams et al. Citation1993; Denda et al. Citation1996; Barba et al. Citation2016). Acetone treatments involve gently and repeatedly rolling acetone-soaked cotton balls or swabs over the skin (Tsai, Hung, et al. Citation2001; Tsai, Sheu, et al. Citation2001; Rissmann et al. Citation2009). The more severe the acetone treatment (intensity and duration), the greater the alteration to the barrier (Fartasch Citation1997). Few studies have assessed the effects of acetone on the skin’s histological structure. Rissmann et al. (Citation2009) demonstrated that treatment with acetone-soaked cotton swabs on hairless mice led to removal of several corneocytes layers compared to untreated skin. However Tsai Sheu, et al. (Citation2001) reported completely contradictory findings. Similar discrepancies are found when measuring variations in TEWL. Thus, several studies indicated impaired permeability after acetone treatment (Tsai Sheu, et al. Citation2001; Rissmann et al. Citation2009; Barcelos et al. Citation2015), whereas others reported no or very few modifications (Abrams et al. Citation1993); Fartasch (Citation1997); Benfeld and Serup (Citation1999). Gajjar and Kasting (Citation2014) consider acetone to be a mild barrier disruptor, with milder effects on skin than chloroform/methanol (2:1), or hexane/methanol (2:3) (Scheuplein and Ross Citation1970; Abrams et al. Citation1993; Barba et al. Citation2016). In many studies, the effects of acetone on barrier integrity and on skin lipids are assessed simultaneously, as lipids are involved in maintaining barrier function and in controlling intercellular and transcellular diffusion. In these experiments, acetone was found to have no effect or only a mild effect on barrier permeability, and few morphological changes were observed in the skin (Fartasch Citation1997; Benfeld and Serup Citation1999). In corroboration of these findings, the amounts of SC lipids (cholesterol, free fatty acids or ceramides) extracted by acetone were not significant in the experimental conditions used by Abrams et al. (Citation1993). In contrast, in patch tests on human volunteers, extensive disruption of less polar intercellular lamellar lipids was found, even though Fartasch (Citation1997) noticed only slight changes for the more polar LB lipid sheets at the SC/SG interface after 1–3 h treatment with acetone.
Following severe treatment of the isolated porcine SC (by immersion in 10 ml acetone for 2 h), Barba et al. (Citation2016) reported extraction of small amounts of lipids, associated with intercellular disruptions and extensive structural defects of intercellular domains including a decrease in the number of intercellular lamellae, extensive disorganization of intercellular lipid bilayers, and total extraction of lipids in some areas along with dilatation of lacunae. All these effects contributed to the loss of cohesion between the layers reported in several articles (Menon et al. Citation1992; Fartasch Citation1997; Tsai et al. Citation2001). Grubauer et al. (Citation1989) described a relationship between the amount of lipids removed and the degree of barrier perturbation. Thus, acetone led to removal of non-polar lipids from the SC, whereas abundant non-polar lipids persisted in sebaceous glands. When barrier perturbation was profound, most polar lipids were also removed from the SC, but not from the viable epidermis.
The main steps in dermal absorption of a solid dissolved in acetone are the same as reported with ethanol: acetone evaporates rapidly from the skin’s surface, especially when less than 50 µL/cm2 is applied (equivalent to a 40-mg/cm2 dose, acetone absorbed dose ≤0.3%) (Gajjar and Kasting Citation2014). As acetone evaporates, the thermodynamic activity of the solute increases, reaching its maximum at saturation, when the driving force is greatest for partitioning of the solute into the SC. The substance then partitions into the upper layers of the skin, diffusing through the various skin layers down to the receptor fluid. As a result, its flux profile reaches an unsustained maximum, as demonstrated for ibuprofen and flurbiprofen (Akhter and Barry Citation2011), or benzoic acid, desoxycorticosterone and testosterone (Scheuplein and Ross Citation1974). Questions remain relating to the absorption of the thin layer of solid into the SC following complete evaporation of the acetone. For most substances, the dissolution of solid materials is rate-limiting and only allows very slow absorption (Stinchcomb et al. Citation1999; Akhter and Barry Citation2011).
Few or no percutaneous absorption studies have assessed acetone-induced effects compared to the effects of other vehicles, or to the substance applied neat. Larese Filon et al. (Citation1999) found that liquid glycol ethers (GE) applied in the presence of acetone (70%) had a reduced lag time and increased permeation rates compared to neat GE. Skin permeability following acetone pretreatment has also rarely been studied. Tsai Sheu, et al. (Citation2001) showed only slightly modified percutaneous penetration of progesterone – a highly lipophilic substance, (log Kow=3.9) – following skin pretreatment. In this case, the Kp ratio was close to 1 between acetone-treated and non-treated whole skins. In contrast, the permeation coefficient for hydrophilic and amphiphilic substances increased markedly, with acetone treatment reducing the resistance and increasing diffusivity through the intercellular pathway. For these substances, the Kp increased 10- or 20-fold. Once again, as for ethanol, this difference could be explained by the distinct diffusion pathways taken by lipophilic and hydrophilic substances.
Artificial sebum
Sebum is naturally excreted by the sebaceous gland, located next to the hair follicle in each pilosebaceous unit. Sebum content varies with age and gender, and production is greater on the forehead than on other areas of skin, such as the forearms (Man et al. Citation2009). Sebum lubricates and hydrates the skin, and also corresponds to the “first barrier,” preventing percutaneous absorption of unwanted substances. At the outlet of the sebaceous gland, sebum is composed of unsaturated and saturated triglycerides (Stefaniak and Harvey Citation2006), saturated and unsaturated wax mono- and di-esters, and squalene. The sebum collected at the surface of the skin also contains saturated and unsaturated fatty acids (more than 220 substances with 14-, 16- or 18-carbon chains). These compounds are mostly produced by partial hydrolysis of triglycerides, cholesterol, and cholesterol esters from the SC by lipases and esterases. Enzymatic activity can also produce some diglycerides. In most publications, “human sebum” designates lipids from the skin’s surface; the terminology “skin surface lipids” (SSL) is also encountered (Friberg and Osborne Citation1986). SSL should not be confused with “skin surface liquid,” “skin surface film liquids” (SSFL) (Stefaniak and Harvey Citation2006; Luo et al. Citation2020), or “human Residual Skin Surface Component” (RSSC) (Shetage et al. Citation2014), as all of these generally also include sweat.
Differences in the composition of sebum have been reported in the literature. They can be attributed to various factors: inter-individual variations (resulting for example in different degrees of hydrolysis of triglycerides to free fatty acids (Downing et al. Citation1969)), variations between body areas (Tsai et al. Citation2012) (the proportion of epidermally-derived lipids is higher in gland-deficient areas such as the arms and the legs than in gland-rich areas such as the face). Differences in collection (Shetage et al. Citation2014), extraction, or analysis methods can also contribute to discrepancies between studies. Despite all these potential sources of differences, based on the compositions reported in the literature, an average composition (% w/w) can be proposed, as follows: 15% squalene, 24% wax esters, 34% triglycerides, 22% free fatty acids, 2.1% cholesterol, 2.4% cholesterol esters, 0.6% diglycerides (Downing et al. Citation1969; Greene et al. Citation1970; Stefaniak and Harvey Citation2006; Valiveti and Lu Citation2007; Lu et al. Citation2009; Tsai et al. Citation2012). Note that only the two first references mention the presence of diglycerides.
No standardized formulation of artificial sebum has been established; authors use either simple formulations (Rush et al. Citation2019; Champmartin et al. Citation2020) or elaborate mixtures (some supplied by the cosmetics industry), with varying degrees of complexity, in an attempt to get as close as possible to human sebum (Stefaniak and Harvey Citation2006; Valiveti and Lu Citation2007; Schneider et al. Citation2016). For the purposes of dissolution studies, Stefaniak and Harvey (Citation2006) critically reviewed artificial sebum formulations presented in articles published between 1940 and 2005; they completed their analysis in 2010 (Stefaniak et al. Citation2010) with information from articles published from 2005 to 2010. According to this analysis, representative artificial sebum must contain at least one constituent among the six first classes of substances mentioned above, at levels close to those reported for human sebum. Based on the data analyzed, Stefaniak et al. developed their own artificial sebum formulation with proportions for each class of lipids close to those mentioned above. Several years later, some authors still refer to Stefaniak’s work when preparing sebum formulations (Schneider et al. Citation2016; Pawar et al. Citation2017; Champmartin et al. Citation2020; Luo et al. Citation2020).
Although there is no standardized formulation for artificial sebum, beyond the composition, it may be necessary to properly characterize the artificial sebum (Valiveti et al. Citation2008; Lu et al. Citation2009) to ensure that its parameters remain stable over time and across studies (Wertz Citation2009; Stefaniak et al. Citation2010). At first glance, using artificial sebum as a vehicle may be a tempting idea, since sebum is naturally present on the skin. However, the amount of sebum experimentally applied to the skin (several mg/cm2) generally far exceeds natural levels (few µg/cm2 up to a maximum of 200 µg/cm2) (Tsai et al. Citation2012; Schneider et al. Citation2016; Rush et al. Citation2019; Champmartin et al. Citation2020), as explained and emphasized by Schneider et al. (Citation2016). In addition, the protocols implemented vary widely. Lack of consensus on formulation and protocols probably explain why few percutaneous absorption experiments have been conducted with this vehicle. To overcome these issues, OECD guidelines relating to skin absorption studies could include a standard formulation of artificial sebum, as suggested by Hopf et al. (Citation2020), along with a protocol for its use. Currently, artificial sebum is used in two ways: the skin is either pretreated with sebum before application of the substance of interest (Tsai et al. Citation2012; Schneider et al. Citation2016; Rush et al. Citation2019), or the sebum is used as vehicle to dissolve the test substance before application (Champmartin et al. Citation2020). In the first case, the substance must be transferred from the vehicle to the sebum (vehicle/sebum partition coefficient) and then diffuse from the sebum into the skin. In the second case, partition occurs directly between the sebum and the SC. Tsai et al. (Citation2012) and Rush et al. (Citation2019) compared skin permeability for substances applied in aqueous vehicles with or without sebum pretreatment, and reported that a combination of artificial sebum with aqueous vehicle increases skin permeability for weakly lipophilic zinc pyrithione and cimetidine (log Kow between 0 and 1). Rush et al. (Citation2019) demonstrated that this combination had an effect on skin integrity in vitro (increase in tritiated water flux), whereas Tsai et al. (Citation2012) reported that exposure of in vivo skins to sebum alone increased skin hydration and slightly decreased TEWL. Based on attenuated total reflectance infrared spectroscopy, the increase in skin permeability was attributed to altered SC barrier function due to disordering of structures in the intercellular lipid bilayers. This conclusion appears to be supported by results for bisphenol S (log Kow = 1.2) applied directly in water or in an artificial sebum, with Champmartin et al. (Citation2020) reporting a ten-fold increase in percutaneous flux with sebum compared to water. In apparent contradiction, however, Schneider et al. (Citation2016) observed no difference in permeability between sebum pretreated skin or control skin upon exposure to liquid solvent, whether hydrophilic like ethanol (log Kow = −0.31) or lipophilic like toluene (log Kow = 2.73). Similar results were reported by Tsai et al. (Citation2012) for cyanophenol (log Kow = 1.6).
Based on these apparently contradictory results and the limited data available, it is difficult to draw conclusions on the effects of sebum on the skin and percutaneous absorption of substances. If the enhancement effect of sebum is confirmed, it could be attributed to its occlusive effect (leading to increased skin hydration), particularly when several mg/cm2 are applied (Schneider et al. Citation2016; Rush et al. Citation2019; Champmartin et al. Citation2020). An ingredient present in sebum formulation could also act as an enhancer. For example, long chain monounsaturated fatty acids with a cis configuration, like 9-octadecaenoic acid (or oleic acid, OA) and 11-octadecaenoic acid, have known chemical enhancer effects (Suhonen et al. Citation1999; Williams and Barry Citation2012; Lane Citation2013; Haque and Talukder Citation2018). They interact with SC lipids, disrupting their ordered orientation and increasing their fluidity (Golden et al. Citation1987; Moghadam et al. Citation2013; Moghimipour et al. Citation2013). However, Naik et al. (Citation1995) suggested these effects to be temporary, reversible, and sustained only by the surface and uppermost layers. Some studies have also pointed out that OA exists in a separate phase (fluid state) within the intercellular lipid bilayers (solid state) (Ongpipattanakul et al. Citation1991; Naik et al. Citation1995). Skin penetration of lipophilic and hydrophilic substances, like caffeine, naproxene, salicylic acid, or thymoquinone, can effectively be increased by low percentages of OA (typically less than 10%) (Golden et al. Citation1987; Haq and Michniak-Kohn Citation2018; Abd et al. Citation2019). It should be noted that for some studies (Golden et al. Citation1987; Ongpipattanakul et al. Citation1991; Naik et al. Citation1995) OA was added as an ethanol solution, and an impact of ethanol cannot be ruled out.
Artificial sweat
Artificial sweat can also be used to analyze percutaneous absorption of chemicals in contact with the other natural human skin surface liquid: sweat. Sweat or perspiration is naturally excreted by three types of glands: eccrine, apocrine, and apoeccrine. Eccrine glands play a major role in overall sweat production due to their number, the fact that they cover the entire surface of the body and that they excrete the highest volume of sweat. The literature mainly contains information on eccrine sweat formation and composition (Stefaniak and Harvey Citation2006; Baker Citation2019). Eccrine sweat is a complex aqueous mixture mostly composed of water (99–99.5%) and sodium chloride (NaCl). However, a multitude of other solutes are also present in varying concentrations: micronutrients (electrolytes, minerals) and metabolic products, proteins, amino acids, and toxicants. Two comprehensive reviews (Stefaniak and Harvey Citation2006; Baker Citation2019) indicated the concentration ranges for each component in sweat, and Baker (Citation2019) highlighted some methodological issues. Both reviews agreed on the concentrations of the most concentrated micronutrients: sodium (Na) and chloride (Cl) (10–90 mmol/L), and also potassium (K) (2–8 mmol/L). For the fifty remaining substances, they only provided orders of magnitude for the concentrations. Furthermore, like for human sebum, the concentrations of many constituents of human sweat and its pH vary widely due to various factors such as diet, season, timing of sweat sampling, degree of acclimation, gender, and body region (Stefaniak and Harvey Citation2006; Harvey et al. Citation2010; Baker Citation2019). Thus, using sweat for percutaneous experiments entails a certain number of inherent difficulties. With the exception of Stauber et al. (Citation1994), who directly collected human sweat, and Dalton et al. (Citation2018) who purchased artificial sweat formulations, most authors prepared their own artificial sweat (Stauber et al. Citation1994; Larese Filon et al. Citation2004; Williams et al. Citation2005; Van Lierde et al. Citation2006; Larese et al. Citation2007; Larese Filon et al. Citation2008; Harvey et al. Citation2010; Bianco et al. Citation2014; Franken et al. Citation2015; Midander et al. Citation2016; Jansen Van Rensburg et al. Citation2017). Compositions are not always described, and are generally barely justified. Like for artificial sebum, the formulation can range from a simple one, based on the formulation given in the European reference method EN 1811 (Test Method for Release of Nickel, (European Committee for Standardization (CEN) Citation2011)) – composed of NaCl, lactic acid and urea in MilliQ or deionized water with an adjusted pH (Larese Filon et al. Citation2004; Larese et al. Citation2007; Larese Filon et al. Citation2008; Franken et al. Citation2015; Midander et al. Citation2016; Jansen Van Rensburg et al. Citation2017) – to a very complex formulation in an attempt to get as close as possible to a “median” human perspiration (Stefaniak and Harvey Citation2006; Harvey et al. Citation2010; Midander et al. Citation2016; Pawar et al. Citation2017; Luo et al. Citation2020). To our knowledge, no comparative data is available on how different artificial sweat formulations affect the skin barrier. Apart from few exceptions as in Midander et al. (Citation2020), sweat formulations are rarely used in percutaneous absorption experiments. Due to the numerous ingredients, their preparation is very time consuming, and it is legitimate to raise the question of how each of these ingredients affects the dissolution of test substances and their percutaneous absorption. Stefaniak and Harvey (Citation2006) reviewed the effect of some sweat ingredients on the solvation of substances (particularly metals); and more recently, reported very few differences in terms of levels of metal released during solvation of metallic reference materials exposed to simple and more comprehensive sweat. The penetration of metal, often in particle form, has frequently been assessed using artificial sweat (pure or diluted in water) as a vehicle (Larese Filon et al. Citation2004; Van Lierde et al. Citation2006; Larese et al. Citation2007; Larese Filon et al. Citation2008; Bianco et al. Citation2014; Franken et al. Citation2015). In addition to the granulometry of the metallic particles, their permeation depends on their oxidation to soluble ions, made possible by the presence of weak organic acid (lactic acid) in the artificial sweat and its pH (Larese Filon et al. Citation2004; Larese et al. Citation2007; Larese Filon et al. Citation2008). The volume of artificial sweat applied is sometimes calculated based on in vivo sweating rates (Dalton et al. Citation2018). When the metal is not oxidized by the sweat, no flux is observed, as for example for chromium (Larese et al. Citation2007). Stefaniak and Harvey (Citation2006) also indicated that amino acids may be required to help dissolve some metals, and indeed, methionine was shown to reduce chromium VI to chromium III (CrIII) (Van Lierde et al. Citation2006). However, the same publication reported the formation of CrIII complexes in the presence of lactic acid, without significant effect on passage through the skin (Van Lierde et al. Citation2006). These few publications show that several ingredients in the composition of artificial sweat, as well as its pH, could influence the absorption of inorganic substances such as metals. Nevertheless, comparative results of percutaneous absorption of substances with and without sweat remain rare. Most studies only tested a single artificial sweat formulation without comparison to another vehicle (sometimes several pH values were compared (Jansen Van Rensburg et al. Citation2017)). Occasionally, comparative experiments were performed with an aqueous solution of the test compounds, for example for chrome (Van Lierde et al. Citation2006) and cobalt (Larese Filon et al. Citation2004). In these cases, sweat had a demonstrated effect on metal speciation. Nevertheless, given the paucity of data, it is difficult to determine whether or not sweat could promote sufficient ionization of metal particles to allow absorption.
The few publications dealing with percutaneous absorption of organic substances and sweat do not use it as vehicle but as a skin pretreatment: applying the sweat first, followed by the substance (Williams et al. Citation2005; Dalton et al. Citation2018). Williams et al. (Citation2005) aimed to determine the influence of a moisture source (in their case, sweat) on percutaneous absorption of a pesticide – chlorpyrifos – contained in nylon carpet fibers. The objective of Dalton et al. (Citation2018) was to assess exposure to the nerve agent VX in various occupational situations, in particular to determine whether the presence of perspiration affects percutaneous absorption of VX. Although neither study reported an effect of sweat treatment on percutaneous absorption compared to untreated skin (dry skin), it would be very unwise to attempt to draw definitive conclusions based on only two studies.
Choosing a vehicle
Elements to take into account
In a context of risk assessment, a vehicle mimicking the occupational exposure scenario should, naturally, be given priority. However, there is often no obvious or possible option, and for these cases we will discuss the criteria to consider when selecting a vehicle. To our knowledge, these criteria have not been extensively discussed in the context of percutaneous absorption studies aiming to assess occupational exposure. In contrast, several authors have broached the subject for topical or transdermal application of cosmetics or pharmaceutics. They all came to the conclusion that there is “no universal vehicle,” and therefore no recommendations or guidelines can be formulated. Thus, for each drug, concentration and therapeutic application, an optimal vehicle must be determined (Idson Citation1983; Smith et al. Citation2005). For cosmetic applications, Lane et al. (Citation2012) provide recommendations for defining the ideal vehicle in a given situation. They suggest choosing the formulation that will deliver the active in an optimal way. In the same year, Williams and Barry (Citation2012) published a list of the properties required for penetration enhancers included in drug formulations. Although the objectives differ when studying percutaneous absorption of pharmaceuticals/cosmetics or of toxicants for risk assessment purposes, we have identified common characteristics to guide the choice of liquid vehicle in this context. From a practical point of view, the vehicle must be easy to apply (and to remove if necessary), which is generally the case for liquid vehicles. It should also be chemically inert: stable over time and unreactive with respect to the substance being investigated. Finally, the mixture corresponding to the vehicle (e.g. sebum or sweat) and the test solution (substance in vehicle) or suspension must be homogeneous and allow even dispersion over the skin. Differences between “pharmaceutical/cosmetic” and occupational approaches can be found in the vehicle-skin interactions. For occupational approaches, a vehicle without deleterious effects on the skin is mandatory – i.e. it should have no action on the structure of the skin and its content, in particular the lipids; nor should it be toxic, irritating, allergenic, or sensitizing. Pharmaceutical or cosmetic dermatological applications may involve a vehicle that softens and relaxes biological tissues. Moreover, the delivery vehicle generally contains one or more enhancers (or possibly retardants) that interact in many ways with skin constituents, reversibly modifying the skin’s structure and increasing (or decreasing) percutaneous absorption of the active substance.
As already mentioned, in vitro experiments designed to assess occupational exposure via the dermal route do not seek to enhance percutaneous absorption of the tested substance. They must, as far as possible, reflect the real exposure conditions, as recommended by the OECD (Citation2004b), and other guidelines and reports on dermal absorption or exposure assessment (Hoang Citation1992; Howes et al. Citation1996; Kielhorn et al. Citation2006; SCCS Citation2010; Dumont et al. Citation2015). For example, for substances marketed as water formulations, such as BE in water-based paints, cleaning agents, or cutting oils, it seems particularly relevant to use water as a vehicle when assessing percutaneous absorption (Korinth et al. Citation2007). Similarly, since ethanol is widely used in insect repellent formulations, it is relevant to use it when testing the percutaneous absorption of insecticides with a view to risk assessment (Qiu et al. Citation1998). For scenarios involving exposure to neat solid substances, the application is not technically feasible and/or controllable, even if some recent proposals such as the use of a transfer device (Aggarwal et al. Citation2019) or direct exposure to aerosols (Neilson et al. Citation2015; Lee et al. Citation2021) may help. The EFSA (Citation2012, Citation2017), based on guidelines published by the OECD (Citation2004a), recommended that “solid materials should be moistened with a minimal volume of vehicle (e.g. water or physiological saline) to make a paste,” to simulate ambient humidity and sweat on the skin. The authors of these guidelines oppose the use of organic solvents. Ideally, the mixture (substance + vehicle) should be the same as the source of exposure in an industrial setting, or should represent a realistic surrogate (when exposure conditions cannot be reproduced); in all cases, the choice of the vehicle and its volume must be justified (OECD Citation2004b).
Checks to be performed
Before launching series of in vitro percutaneous absorption experiments with a selected vehicle, its effects on the parameters listed in this section should be checked. When performing these studies, the vehicle must be applied without the test substance, but in the same in vitro experimental conditions (in particular volume and experiment duration) as those to be used with the test substance.
Skin barrier integrity
Skin barrier integrity is usually assessed before in vitro percutaneous absorption experiments to check if a skin sample is diseased or damaged, whether the skin has been freshly collected or stored. If it is recommended to check it after the experiment, it may also be important and relevant to check the effect of the vehicle (without the test substance) on skin integrity (USEPA Citation2004; OECD Citation2011). Whatever the method used, measurements should be taken on clean dry skin, because the presence of a substance at the surface of the skin could influence the result. Therefore, the vehicle must be removed at the end of the experiment before checking skin integrity.
As an alternative to measuring skin integrity, corneoxenometry assesses alterations to the SC resulting from the action of a substance. Experiments are performed on cyanoacrylate skin surface stripping samples collected from skin sections. This technique could help to select potentially appropriate vehicles, and particularly to avoid harmful solutions. The colorimetric technique cheaply and rapidly reveals the removal and disorganization of lipids from the SC, as well as the denaturation of proteins in the SC (Goffin et al. Citation2000).
Skin hydration level
As previously indicated, the skin’s hydration state will affect the flux of a test substance. For this reason, it is important to measure the humidity (ambient hygrometry) and the skin hydration. If the vehicle is appropriate for the exposure scenario, any moisturizing effect on the skin is not problematic. In the other cases, the vehicle should barely modify the skin during in vitro percutaneous absorption experiments compared to a control skin without vehicle. Ideally, the state of hydration should be close to that found in vivo and remain stable throughout the experiment. Consequently, occlusion is not recommended (except to simulate the effect of wearing gloves, for example).
The standard methods used to measure skin hydration are based on the electrical properties of water, either capacitance or conductance, which changes based on the skin’s water content. A Corneometer® is commonly used in vivo but also in vitro to test the effects of cosmetics on skin hydration (Girard et al. Citation2000; Heinrich et al. Citation2003; Osseiran et al. Citation2018). Corneometry is a simple, rapid, effective, nondestructive, and relatively inexpensive method; however, it also has some inherent drawbacks. For example, the system must be calibrated to establish the relationship between the Corneometer® measurement (expressed in arbitrary units) and the skin’s water content. In addition, the measurement is limited to a surface area of 1 cm2 at a small depth (10 − 20 µm of the SC). This limitation makes it impossible to distinguish between the different skin layers, or to differentiate between intra- and extra-cellular hydration (Barel and Clarys Citation1997; Girard et al. Citation2000; Osseiran et al. Citation2018). A major drawback when seeking to test the effect of a vehicle is that Corneometer® measurements appear to be influenced by the composition of any products applied to the skin. Indeed, some substances can form an insulating film on the surface of the skin, which may interfere with measurements (e.g. polar substances or electrically conducting solutions like sweat) (Girard et al. Citation2000).
Alternatively, and very simply, skin water content can also be measured by gravimetric determination (Bouwstra et al. Citation1991, Citation1992, Citation2003) or thermogravimetric analysis (Thomas and Panchagnula Citation2003; Shah et al. Citation2008). These methods require a skin dehydration step (destructive step) which is not a problem as this test must be performed before the percutaneous absorption experiments. Some authors recently used infrared (IR) or near IR (NIR) technologies to measure skin hydration (Zhang et al. Citation2005; Shah et al. Citation2008; Lee et al. Citation2018). The relative water content was calculated from the water-to-protein ratio, by comparing CH and OH bands in SC tape-stripping samples analyzed by Fourier Transform IR spectroscopy (Lee et al. Citation2018). A multispectral imaging method developed by Zhang et al. (Citation2005) measures the absorption of NIR light by water in living tissue based on its reflectance spectrum (band at 1450 nm). This technique is rapid, non-contact and non-invasive, and has the additional advantage of revealing the degree of hydration for different locations. It can also be used to quantify overall skin hydration. However, it provides no information on skin hydration as a function of depth. Various optical microscope-based techniques are available for that purpose, most notably the destructive sampling technique cryo-scanning electron microscopy (Bouwstra et al. Citation2003). Raman spectroscopy combined with confocal scanning laser microscopy (Caspers et al. Citation2001, Citation2003), coherent Raman scattering imaging (Osseiran et al. Citation2018), and optical coherence tomography (Sand et al. Citation2006) also offer alternative possibilities for non-invasive and non-destructive skin research and skin characterization at a high spatial resolution. These techniques can be used to calculate the water content at various depths within the skin. Nuclear Magnetic Resonance Spectroscopy (NMR-S), which is also a non-destructive method, provides extremely precise and direct quantification of cutaneous hydration throughout the epidermis and the superficial dermis. However, it provides an overall moisture content, and unlike the previously-mentioned methods, cannot be used to study the water concentration profile. Alternative methods based on the thermal properties of water also exist. For example, transient heat transfer can be non-invasively measured by the Hydrascan®, developed by Laboratoire Dermscan (Villeurbanne, France), to determine hydration measurements from the surface down to three epidermal depths (Girard et al. Citation2000). Finally, Jachowicz et al. (Citation2005) developed the fast dew point hygrometer with an integrated semiconductor detector specifically to measure skin moisture content.
Further details on all the existing methods can be found in Verdier-Sévrain and Bonté (Citation2007). Some authors have compared the results obtained with two or three of these methods used in parallel during experimental studies (Barel and Clarys Citation1997; Girard et al. Citation2000; Zhang et al. Citation2005; Shah et al. Citation2008; Osseiran et al. Citation2018). By assessing skin hydration in different ways at different depths, they provide complementary information (Girard et al. Citation2000). On principle, in the context of a panel of tests to retain or exclude a vehicle, it is not necessarily useful to deploy sophisticated techniques and expensive equipment; an overall measurement by corneometry, which is easy to implement, could be sufficient. Up to now, no guideline advocates measuring skin hydration. However, it would be desirable to include a paragraph on skin hydration and methodological issues in the new version of GN156, which is currently being revised. By establishing a consensus on one of the methods, practices could be harmonized across in vitro percutaneous absorption experiments.
Histology: alterations to the skin/skin appendages
Histological examination can be performed as part of the preliminary tests carried out to ensure that the vehicle selected is relevant. In most publications, classical histology is applied; the material is immersed and fixed, solidified by embedding in a substrate or freezing, sliced at a few µm thick and stained for observation under a microscope. This method can be used to verify the preservation of the structure and morphology of the skin and its appendages (Dick and Scott Citation1992; Lauer et al. Citation1997; Rajadhyaksha et al. Citation1999; Knapik et al. Citation2013; Cleland et al. Citation2014), in particular the thickness of the different layers (Dick and Scott Citation1992; Khiao In et al. Citation2019; Marquet et al. Citation2019), the number of cell layers (Rissmann et al. Citation2009; Khiao In et al. Citation2019), the follicle depths (Dick and Scott Citation1992), the number and size of sebaceous glands (Lauer et al. Citation1997). It is important to ensure that the SC has not been modified, destroyed, disrupted or partially removed (Bond and Barry Citation1988; Messager et al. Citation2003; Rissmann et al. Citation2009; Zhou and Banga Citation2011; Dabboue et al. Citation2015; Praça et al. Citation2018; Alalaiwe et al. Citation2020), and that the different layers have not become separated (Bond and Barry Citation1988; Sterne et al. Citation2000; Turhan-Haktanir et al. Citation2011). Histology can also be used to detect abnormalities at the cellular level (Rajadhyaksha et al. Citation1999; Sterne et al. Citation2000; Turhan-Haktanir et al. Citation2011), and could provide information on skin viability by determining levels of apoptosis or other forms of cell death, thanks to immunohistochemistry techniques (Knapik et al. Citation2013; Boekema et al. Citation2015). Histology thus seems adapted and useful in the framework of preliminary tests, but not as a routine test during experiments as it entails destruction of the skin sample. In addition, histology requires a lot of practice and know-how to refine observational skills. The protocol must be adapted to the objectives of the study. For example, to observe an effect on SC lipids, the classic histological preparation, which involves embedding samples in paraffin, must be avoided as it would dissolve the lipids; cryostat sections should be used instead. Other artifacts could also lead to disruption of the SC and corneocytes and lead to erroneous conclusions (Rajadhyaksha et al. Citation1999).
Effects on viability/metabolism
Even though it may affect the percutaneous absorption of a substance, the effect of vehicles on viability and/or metabolism of the skin is incompletely documented in the literature. Indeed, only a few publications report the effects of ethanol on these parameters. Reports on the effects of other vehicles are even more rare, and use a number of distinct methodologies, making the results difficult to interpret. Consequently, these subjects will need to be further investigated. As viability and metabolism are linked, several researchers used measures of specific enzymatic activity in the skin to conclude on its viability. Skin is acknowledged to be a metabolic site, and the metabolic capacity of skin explants has been more extensively investigated over the last decade (Eilstein et al. Citation2014; Manevski et al. Citation2015; Oesch et al. Citation2018). Corrosive effects and loss of viability also correlate, and it is recommended to assess the corrosive effect of a substance on skin based on cellular viability measurements (OECD Citation2019). Some methods to assess skin viability are briefly discussed in Hopf et al. (Citation2020). When assessing the cutaneous metabolism of a test substance, if the metabolic pathways are known, the effect of the vehicle on these pathways should be investigated. Otherwise, for the moment, we can particularly recommend that ethanol be avoided because of its effects on various enzymes.
Conclusion
This narrative review is primarily intended for researchers aiming to experimentally assess occupational dermal exposure to solid toxicants using in vitro experiments. The experimental setup requires a certain number of parameters to be tuned and several choices to be made, including selection of the vehicle. There is no ideal vehicle, but in a context of risk assessment, a vehicle mimicking the occupational exposure scenario should be given priority. Very often, there is no obvious, or even possible, option. In that case, the researcher must identify the vehicle that has least influence on the skin barrier and on flux to obtain reliable percutaneous absorption results for the test substance. Thus, in the absence of sufficient data in the literature, we recommend performing preliminary experiments with the selected vehicle alone to test how it affects the skin’s integrity and hydration. If the researchers have the requisite histology skills, it is a good idea to check effects on the structure of the skin.
Except when seeking to mimic exposure to aqueous solutions of toxicants, water should be used with caution as it is a well-known enhancer, interacting with both lipids and corneocytes in the SC. Considering the various effects of ethanol, it is not recommended for use neat, and it should particularly be avoided when studying skin metabolism. There is little advantage to using it in combination with water. The use of a volatile solvent may be an alternative means to directly deposit solid substances. In this case, a small deposit volume should be used to allow rapid evaporation and limit the effect on the skin barrier. Acetone is preferable to ethanol. Although more realistic, the use of artificial sebum requires first to decide on its composition, and then to develop a technique to evenly apply a small volume to the skin, to accurately mimic its secretion in vivo. After all these preliminary steps, it will be necessary to assess the effects of the artificial sebum on the skin. For artificial sweat, it is currently difficult to conclude, given the paucity of data and the lack of consensus composition. However, we recommend that the solution be isotonic, including artificial sweat or NaCl 0.9% in water, rather than ultrapure water in its composition.
The tests to be carried out with the vehicles and the methodology must be discussed between researchers pursuing similar objectives, and guidelines should address the corresponding protocols, as well as providing information on vehicles (volume, deposition time). Harmonization of laboratory practices is an issue to be considered when seeking to obtain more comparable and reproducible results.
Acknowledgements
The manuscript was sent to The Scientific Board Executive of INRS (Institut National de Recherche et de Sécurité) for review, and no objections were received. The authors would like to acknowledge Dr Pierre Campo, head of Toxicology and Biomonitoring division, for his excellent support and advice and for his critical review of the document. They are also pleased to acknowledge the anonymous reviewers the Editor selected.
Declaration of interest
The authors declare that they have no competing interests to disclose related to the submission of this manuscript. At the time of writing all authors are employed by the INRS, a non-profit organization under the egis of the French Social Insurance system. Fabrice Marquet is a member of the expert group working on the OECD N°156 Guidance notes on dermal absorption (Draft Second Edition). The other authors have not participated in and do not anticipate participation in any legal, regulatory, or advocacy proceedings related to the contents of the paper. This article has not received external funding support.
References
- Abd E, Benson HAE, Mohammed YH, Roberts MS, Grice JE. 2019. Permeation mechanism of caffeine and naproxen through in vitro human epidermis: effect of vehicles and penetration enhancers. Skin Pharmacol Physiol. 32(3):132–141.
- Abdallah MAE, Pawar G, Harrad S. 2015. Evaluation of 3D-human skin equivalents for assessment of human dermal absorption of some brominated flame retardants. Environ Int. 84:64–70.
- Abdallah MAE, Pawar G, Harrad S. 2016. Human dermal absorption of chlorinated organophosphate flame retardants; implications for human exposure. Toxicol Appl Pharmacol. 291:28–37.
- Abrams K, Harvell JD, Shriner D, Wertz P, Maibach H, Maibach HI, Rehfeld SJ. 1993. Effect of organic solvents on in vitro human skin water barrier function. J Invest Dermatol. 101(4):609–613.
- Aggarwal M, Fisher P, Kluxen FM, Maas W, Morgan N, Parr-Dobrzanski R, Strupp C, Wiemann C. 2019. Assessing in vitro dermal absorption of dry residues of agrochemical sprays using human skin within OECD TG 428. Regul Toxicol Pharmacol. 106:55–67.
- Akhter AS, Barry BW. 2011. Absorption though human skin of ibuprofen and flurbiprofen; effect of doses variation, deposited drug films, occlusion and the penetration enhancer n-methyl-2-pyrrolidone. J Pharm Pharmacol. 37(1):27–37.
- Alalaiwe A, Lin YK, Lin CH, Wang PW, Lin JY, Fang JY. 2020. The absorption of polycyclic aromatic hydrocarbons into the skin to elicit cutaneous inflammation: the establishment of structure-permeation and in silico-in vitro-in vivo relationships. Chemosphere. 255:126955.
- Ananthapadmanabhan KP, Moore DJ, Subramanyan K, Misra M, Meyer F. 2004. Cleansing without compromise: the impact of cleansers on the skin barrier and the technology of mild cleansing. Dermatol Ther. 17(s1):16–25.
- Anderson SE, Meade BJ. 2014. Potential health effects associated with dermal exposure to occupational chemicals. Environ Health Insights. 8(Suppl 1):51–62.
- Andrews SN, Jeong E, Prausnitz MR. 2013. Transdermal delivery of molecules is limited by full epidermis, not just stratum corneum. Pharm Res. 30(4):1099–1109.
- Atobe T, Mori M, Yamashita F, Hashida M, Kouzuki H. 2015. Artificial neural network analysis for predicting human percutaneous absorption taking account of vehicle properties. J Toxicol Sci. 40(2):277–294.
- Azzi CG, Zhang J, Purdon CH, Smith EW, Surber C, Maibach HI. 2005. Chapter 58, Topical dermatological vehicles: Engineering the delivery system. In Bronaugh RL, Maibach HI, editors. Percutaneous absorption_ drugs, cosmetics, mechanisms, methodology. Boca Raton (FL): Taylor and Francis Group. p. 801–809.
- Baker LB. 2019. Physiology of sweat gland function: the roles of sweating and sweat composition in human health. Temperature. 6(3):211–259.
- Bamba FL, Wepierre J. 1993. Role of the appendageal pathway in the percutaneous absorption of pyridostigmine bromide in various vehicles. Eur J Drug Metab Pharmacokinet. 18(4):339–348.
- Barba C, Alonso C, Marti M, Manich A, Coderch L. 2016. Skin barrier modification with organic solvents. Biochim Biophys Acta. 1858(8):1935–1943.
- Barba C, Martí M, Semenzato A, Baratto G, Manich AM, Coderch L. 2015. Effect of lipid modification on stratum corneum permeability. J Therm Anal Calorim. 120(1):297–305.
- Barcelos RC, de Mello-Sampayo C, Antoniazzi CT, Segat HJ, Silva H, Veit JC, Piccolo J, Emanuelli T, Burger ME, Silva-Lima B, et al. 2015. Oral supplementation with fish oil reduces dryness and pruritus in the acetone-induced dry skin rat model. J Dermatol Sci. 79(3):298–304.
- Barel AO, Clarys P. 1997. In vitro calibration of the capacitance method (Corneometer CM 825) and conductance method (Skicon-200) for the evaluation of the hydration state of the skin. Skin Res Technol. 3(2):107–113.
- Barry BW. 1987. Mode of action of penetration enhancers in human skin. J Control Release. 6(1):85–97.
- Bashir SJ, Maibach HI. 2005. Cutaneous metabolism of xenobiotics. In: Bronaugh RL, Maibach HI, editors. Percutaneous absorption_ drugs, cosmetics, mechanisms, methodology. fourth edition ed. Boca Raton (FL): Taylor and Francis Group. p. 51–64.
- Benfeld E, Serup J. 1999. Effect of barrier perturbation on cutaneous penetration of salicylic acid in hairless rats: in vivo pharmaco kinetics using microdialysis and non-invasive quantification of barrier function. Arch Dermatol Res. 291(9):517–526.
- Berner B, Juang RH, Mazzenga GC. 1989. Ethanol and water sorption into stratum corneum and model systems. J Pharm Sci. 78(6):472–476.
- Berner B, Mazzenga GC, Otte JH, Steffens RJ, Juang RH, Ebert CD. 1989. Ethanol: water mutually enhanced transdermal therapeutic system II: Skin permeation of ethanol and nitroglycerin. J Pharm Sci. 78(5):402–407.
- Bianco C, Adami G, Crosera M, Larese F, Casarin S, Castagnoli C, Stella M, Maina G. 2014. Silver percutaneous absorption after exposure to silver nanoparticles: a comparison study of three human skin graft samples used for clinical applications. Burns. 40(7):1390–1396.
- Boekema BK, Boekestijn B, Breederveld RS. 2015. Evaluation of saline, RPMI and DMEM/F12 for storage of split-thickness skin grafts. Burns. 41(4):848–852.
- Bolzinger M-A, Briançon S, Pelletier J, Chevalier Y. 2012. Penetration of drugs through skin, a complex rate-controlling membrane. Curr Opin Colloid Interface Sci. 17(3):156–165.
- Bommannan D, Potts RO, Guy RH. 1991. Examination of the effect of ethanol on human stratum corneum in vivo using infrared spectroscopy. J Control Release. 16(3):299–304.
- Bond JR, Barry BW. 1988. Limitations of hairless mouse skin as a model for in vitro permeation studies through human skin: hydration damage. J Invest Dermatol. 90(4):486–489.
- Bourgart E. 2019. Métabolisme cutané et biomarqueurs d'exposition aux mélanges complexes d'hydrocarbures aromatiques polycycliques [Cutaneous metabolism and biomarkers of exposure to complex mixtures of polycyclic aromatic hydrocarbons]. [thesis]. Grenoble: Université Grenoble Alpes. French.
- Bouwstra JA, de Graaff A, Gooris GS, Nijsse J, Wiechers JW, van Aelst AC. 2003. Water distribution and related morphology in human stratum corneum at different hydration levels. J Invest Dermatol. 120(5):750–758.
- Bouwstra JA, Gooris GS. 2010. The lipid organisation in human stratum corneum and model systems. TODJ. 4(1):10–13.
- Bouwstra JA, Gooris GS, Salomons-de Vries MA, van der Spek JA, Bras W. 1992. Structure of human stratum corneum as a function of temperature and hydration: a wide-angle X-ray diffraction study. Int J Pharm. 84(3):205–216.
- Bouwstra JA, Gooris GS, Van der Spek JA, Bras W. 1991. Structural investigations of human stratum corneum by small-angle X-ray scattering. J Invest Dermatol. 97(6):1005–1012.
- Brown TN, Armitage JM, Egeghy P, Kircanski I, Arnot JA. 2016. Dermal permeation data and models for the prioritization and screening-level exposure assessment of organic chemicals. Environ Int. 94:424–435.
- Bunge AL, Persichetti JM, Payan JP. 2012. Explaining skin permeation of 2-butoxyethanol from neat and aqueous solutions. Int J Pharm. 435(1):50–62.
- Caspers PJ, Lucassen GW, Carter EA, Bruining HA, Puppels GJ. 2001. In vivo confocal Raman microspectroscopy of the skin: noninvasive determination of molecular concentration profiles. J Invest Dermatol. 116(3):434–442.
- Caspers PJ, Lucassen GW, Puppels GJ. 2003. Combined in vivo confocal Raman spectroscopy and confocal microscopy of human skin. Biophys J. 85(1):572–580.
- Champmartin C, Marquet F, Chedik L, Décret MJ, Aubertin M, Ferrari E, Grandclaude MC, Cosnier F. 2020. Human in vitro percutaneous absorption of bisphenol S and bisphenol A: a comparative study. Chemosphere. 252:126525.
- Chittenden JT, Riviere JE. 2017. Chapter 6, The effects of vehicle mixtures on transdermal absorption: thermodynamics, mechanisms, assessment, and prediction. In: Dragicevic N, Maibach HI, editors. Percutaneous penetration enhancers drug penetration into/through the skin. Berlin Heidelberg: Springer Verlag; p. 95–118.
- Cleland H, Wasiak J, Dobson H, Paul M, Pratt G, Paul E, Herson M, Akbarzadeh S. 2014. Clinical application and viability of cryopreserved cadaveric skin allografts in severe burn: a retrospective analysis. Burns. 40(1):61–66.
- Coderch L, López O, de la Maza A, Parra JL. 2003. Ceramides and skin function. Am J Clin Dermatol. 4(2):107–129.
- Crawford M, Dagnino L. 2017. Scaffolding proteins in the development and maintenance of the epidermal permeability barrier. Tissue Barriers. 5(4):e1341969.
- Dabboue H, Builles N, Frouin E, Scott D, Ramos J, Marti-Mestres G. 2015. Assessing the impact of mechanical damage on full-thickness porcine and human skin using an in vitro approach. Biomed Res Int. 2015:1–10.
- Dalton C, Graham S, Jenner J. 2018. Effect of aqueous dilution on the absorption of the nerve agent VX through skin in vitro. Toxicol in Vitro. 53:121–125.
- Dancik Y, Jepps OG, R MS. 2008. Chapter 12, Beyond stratum corneum. In Roberts MS, Walters KA, editors. Dermal absorption and toxicity assessment second edition ed. New York: Taylor and Francis Group; p. 209–250.
- Denda M, Wood LC, Emami S, Calhoun C, Brown BE, Elias PM, Feingold KR. 1996. The epidermal hyperplasia associated with repeated barrier disruption by acetone treatment or tape stripping cannot be attributed to increased water loss. Arch Dermatol Res. 288(5–6):230–238.
- Dias M, Hadgraft J, Lane ME. 2007. Influence of membrane-solvent-solute interactions on solute permeation in skin. Int J Pharm. 340(1–2):65–70.
- Dick IP, Scott RC. 1992. The influence of different strains and age on in vitro rat skin permeability to water and mannitol. Pharm Res. 9(7):884–887.
- Downing DT, Abraham W, Wegner BK, Willman KW, Marshall JL. 1993. Partition of sodium dodecyl sulfate into stratum corneum lipid liposomes. Arch Dermatol Res. 285(3):151–157.
- Downing DT, Strauss JS, Pochi PE. 1969. Variability in the chemical composition of human skin surface lipids. J Invest Dermatol. 53(5):322–327.
- Dumont C, Prieto P, Asturiol D, Worth A. 2015. Review of the availability of in vitro and in silico methods for assessing dermal bioavailability. Appl in Vitro Toxicol. 1(2):147–164.
- EFSA 2012. Guidance on dermal absorption. EFSA J. 10:4.
- EFSA 2017. Guidance on dermal absorption. EFSA J. 15:6.
- Eilstein J, Lereaux G, Budimir N, Hussler G, Wilkinson S, Duche D. 2014. Comparison of xenobiotic metabolizing enzyme activities in ex vivo human skin and reconstructed human skin models from skinethic. Arch Toxicol. 88(9):1681–1694.
- El Maghraby GM. 2017. Chapter 2, Occlusive versus nonocclusive application in transdermal drug delivery. In: Dragicevic N, Maibach HI, editors. Percutaneous penetration enhancers drug penetration into/through the skin. Berlin Heidelberg: Springer; p. 27–34.
- Elias PM. 1983. Epidermal lipids, barrier function, and desquamation. J Invest Dermatol. 80(1 Suppl):44s–49s.
- Esposto Biondo N, Fretes Argenta D, Schneider Rauber G, Caon T. 2021. How to define the experimental conditions of skin permeation assays for drugs presenting biopharmaceutical limitations? Int J Pharm. 607:120987.
- European Committee for Standardization (CEN), 2011. Reference Test Method for Release of Nickel from All Post Assemblies Wich Are Inserted into Pierced Parts of the Human Body and Articles Intended to Come into Direct and Prolonged Contact with the skin. Vol. EN1811.
- Fabian E, Oesch F, Ott K, Landsiedel R, van Ravenzwaay B. 2017. A protocol to determine dermal absorption of xenobiotica through human skin in vitro. Arch Toxicol. 91(3):1497–1511.
- Fartasch M. 1997. Ultrastructure of the epidermal barrier after irritation. Microsc Res Tech. 37(3):193–199.
- Feldman RJ, Maibach H. 1974. Percutaneous penetration of some pesticides in man. Toxicol Appl Pharmacol. 28(1):126–132.
- Feldmann RJ, Maibach HI. 1969. Percutaneous penetration of steroids in man. J Invest Dermatol. 52(1):89–94.
- Feldmann RJ, Maibach HI. 1970. Absorption of some organic compounds through the skin in man. J Invest Dermatol. 54(5):399–404.
- Franken A, Eloff FC, du Plessis J, Badenhorst CJ, Du Plessis JL. 2015. In vitro permeation of platinum through African and Caucasian skin. Toxicol Lett. 232(3):566–572.
- Franz TJ. 1975. Percutaneous absorption on the relevance of in vitro data. J Invest Dermatol. 64(3):190–195.
- Frasch HF, Barbero AM, Dotson GS, Bunge AL. 2014. Dermal permeation of 2-hydroxypropyl acrylate, a model water-miscible compound: effects of concentration, thermodynamic activity and skin hydration. Int J Pharm. 460(1–2):240–247.
- Friberg SE, Osborne DW. 1986. Interaction of a model skin surface lipid with a modified triglyceride. J Am Oil Chem Soc. 63(1):123–126.
- Froebe C, Simion F, Rhein L, Cagan R, Kligman A. 1990. Stratum corneum lipid removal by surfactants: Relation to in vivo irritation. Dermatologica. 181(4):277–283.
- Gajjar RM, Kasting GB. 2014. Absorption of ethanol, acetone, benzene and 1,2-dichloroethane through human skin in vitro: a test of diffusion model predictions. Toxicol Appl Pharmacol. 281(1):109–117.
- Garcia B, M JP, Wepierre J. 1980. Etude des facteurs conditionnant l'absorption percutanée des alcools incorporés dans des mélanges eau-monooléate de sorbitanne polyoxyethylene-mytistate d'isopropyle. Int J Pharm. 4(3):205–217.
- Geerligs M. 2009. Skin layer mechanics [Thesis]. Eindhoven, The Netherlands Universiteitsdrukkerij TU Eindhoven.
- Georgel A. 2008. Pénétration transcutanée des substances actives. Application en dermocosmétologie. [Percutaneous penetration of active substances. Application in dermocosmetology.] [Thesis]. Nancy: Université Nancy Poincaré. French.
- Girard P, Beraud A, Sirvent A. 2000. Study of three complementary techniques for measuring cutaneous hydration in vivo in human subjects: NMR spectroscopy, transient thermal transfer and corneometry – application to xerotic skin and cosmetics. Skin Res Technol. 6(4):205–213.
- Goffin V, Henry F, Piérard-Franchimont C, Piérard GE. 2000. Penetration enhancers assessed by corneoxenometry. Skin Pharmacol Appl Skin Physiol. 13(5):280–284.
- Golden GM, McKie JE, Potts RO. 1987. Role of stratum corneum lipid fluidity in transdermal drug flux. J Pharm Sci. 76(1):25–28.
- Greene RS, Downing DT, Pochi PE, Strauss JS. 1970. Anatomical variation in the amount and composition of human skin surface lipid. J Invest Dermatol. 54(3):240–247.
- Grice JE, Ciotti S, Weiner N, Lockwood P, Cross SE, Roberts MS. 2010. Relative uptake of minoxidil into appendages and stratum corneum and permeation through human skin in vitro. J Pharm Sci. 99(2):712–718.
- Grubauer G, Elias PM, R FK. 1989. Transepidermal water loss: the signal for recovery of barrier structure and function. J Lipid Res. 30(3):323–333.
- Grubauer G, Feingold KR, Harris RM, Elias PM. 1989. Lipid content and lipid type as determinants of the epidermal permeability barrier. J Lipid Res. 30(1):89–96.
- Guth K, Schafer-Korting M, Fabian E, Landsiedel R, van Ravenzwaay B. 2015. Suitability of skin integrity tests for dermal absorption studies in vitro. Toxicol in Vitro. 29(1):113–123.
- Gwak HS, Oh IS, Chun IK. 2004. Transdermal delivery of ondansetron hydrochloride: effects of vehicles and penetration enhancers. Drug Dev Ind Pharm. 30(2):187–194.
- Haq A, Michniak-Kohn B. 2018. Effects of solvents and penetration enhancers on transdermal delivery of thymoquinone: permeability and skin deposition study. Drug Deliv. 25(1):1943–1949.
- Haque T, Talukder MMU. 2018. Chemical enhancer: a simplistic way to modulate barrier function of the stratum corneum. Adv Pharm Bull. 8(2):169–179.
- Harvey CJ, LeBouf RF, Stefaniak AB. 2010. Formulation and stability of a novel artificial human sweat under conditions of storage and use. Toxicol in Vitro. 24(6):1790–1796.
- Hatanaka T, Shimoyama M, Sugibayashi K, Morimoto Y. 1993. Effect of vehicle on the skin permeability of drugs: polyethylene glycol 400-water and ethanol-water binary solvents. J Control Release. 23(3):247–260.
- Heinrich U, Koop U, Leneveu-Duchemin M-C, Osterrieder K, Bielfeldt S, Chkarnat C, Degwert J, Häntschel D, Jaspers S, Nissen H-P, et al. 2003. Multicentre comparison of skin hydration in terms of physical-, physiological- and product-dependent parameters by the capacitive method (Corneometer CM 825). Int J Cosmet Sci. 25(1-2):45–53.
- Hewitt NJ, Gregoire S, Cubberley R, Duplan H, Eilstein J, Ellison C, Lester C, Fabian E, Fernandez J, Genies C, et al. 2020. Measurement of the penetration of 56 cosmetic relevant chemicals into and through human skin using a standardized protocol. J Appl Toxicol. 40(3):403–415.
- Hoang KT. 1992. Dermal exposure assessment: principles and applications. Washington (USA): U.S. Environmental Protection Agency, Office of Health and Environmental Assessment.
- Hopf N, Champmartin C, Schenk L, Berthet A, Chedik L, Du Plessis J, Franken A, Frasch F, Gaskin S, Johanson G, et al. 2020. Reflections on the OECD guidelines for in vitro skin absorption studies. Regul Toxicol Pharmacol. 117:104752.
- Howes D, Guy R, Hadgraft J, Heylings J, Hoeck U, Kemper F, Maibach HI, Marty JP, Merk H, Parra J, et al. 1996. Methods for assessing percutaneous absorption: the report and recommendations of ECVAM workshop 13. Altern Lab Anim. 24(1):81–106.
- Hui XY, Wester RC, Zhai H, Maibach HI. 2005. Chapter 21, Chemical partitioning into powdered human stratum corneum: a useful in vitro model for studying interaction of chemicals and human skin. In: Bronaugh RL, Maibach HI, editors. Percutaneous absorption_ drugs, cosmetics, mechanisms, methodology. Boca Raton (FL): Taylor and Francis Group p. 291–302.
- Idson B. 1983. Vehicle effects in percutaneous absorption. Drug Metab Rev. 14(2):207–222.
- Igawa S, Kishibe M, Murakami M, Honma M, Takahashi H, Iizuka H, Ishida-Yamamoto A. 2011. Tight junctions in the stratum corneum explain spatial differences in corneodesmosome degradation. Exp Dermatol. 20(1):53–57.
- Intarakumhaeng R, Li SK. 2014. Effects of solvent on percutaneous absorption of nonvolatile lipophilic solute. Int J Pharm. 476(1–2):266–276.
- Intarakumhaeng R, Wanasathop A, Li SK. 2018. Effects of solvents on skin absorption of nonvolatile lipophilic and polar solutes under finite dose conditions. Int J Pharm. 536(1):405–413.
- Iwai I, Kunizawa N, Yagi E, Hirao T, Hatta I. 2013. Stratum corneum drying drives vertical compression and lipid organization and improves barrier function in vitro. Acta Derm Venereol. 93(2):138–143.
- Jachowicz R, Weremczuk J, Tarapata G. 2005. Transepidermal water loss sensor based on fast dew point hygrometer. Sens Actuators A Phys. 123–124:7–11.
- Jansen Van Rensburg S, Franken A, Du Plessis J, Du Plessis JL. 2017. The influence of pH on the in vitro permeation of rhodium through human skin. Toxicol Ind Health. 33(6):487–494.
- Kadir R, Stempler D, Liron Z, Cohen S. 1987. Delivery of theophylline into excised human skin from alkanoic acid solutions: a "push-pull" mechanism. J Pharm Sci. 76(10):774–779.
- Kasting GB, Miller MA, Talreja PS. 2005. Chapter 13, Percutaneous absorption_ drugs, cosmetics, mechanisms, methodology. In: Bronaugh RL, Maibach HI, editors. Percutaneous absorption_ drugs, cosmetics, mechanisms, methodology. fourth edition ed. Boca Raton (FL): Taylor and Francis Group. 193–212.
- Kaushik D, Michniak-Kohn B. 2010. Percutaneous penetration modifiers and formulation effects: Thermal and spectral analyses. AAPS PharmSciTech. 11(3):1068–1083.
- Kendall AC, Kiezel-Tsugunova M, Brownbridge LC, Harwood JL, Nicolaou A. 2017. Lipid functions in skin: Differential effects of n-3 polyunsaturated fatty acids on cutaneous ceramides, in a human skin organ culture model. Biochim Biophys Acta Biomembr. 1859(9 Pt B):1679–1689.
- Khiao In M, Richardson KC, Loewa A, Hedtrich S, Kaessmeyer S, Plendl J. 2019. Histological and functional comparisons of four anatomical regions of porcine skin with human abdominal skin. Anat Histol Embryol. 48(3):207–217.
- Kielhorn J, Melching-Kollmuß S, Mangelsdorf I. 2006. Environmental health criteria 235 dermal absorption. Geneva: World Health Organization.
- Knapik A, Kornmann K, Kerl K, Calcagni M, Contaldo C, Vollmar B, Giovanoli P, Lindenblatt N. 2013. Practice of split-thickness skin graft storage and histological assessment of tissue quality. J Plast Reconstr Aesthet Surg. 66(6):827–834.
- Korinth G, Jakasa I, Wellner T, Kezic S, Kruse J, Schaller KH. 2007. Percutaneous absorption and metabolism of 2-butoxyethanol in human volunteers: a microdialysis study. Toxicol Lett. 170(2):97–103.
- Korinth G, Wellner T, Schaller KH, Drexler H. 2012. Potential of the octanol-water partition coefficient (logP) to predict the dermal penetration behaviour of amphiphilic compounds in aqueous solutions. Toxicol Lett. 215(1):49–53.
- Kretsos K, Miller MA, Zamora-Estrada G, Kasting GB. 2008. Partitioning, diffusivity and clearance of skin permeants in mammalian dermis. Int J Pharm. 346(1–2):64–79.
- Kurihara-Bergstrom T, Knutson K, DeNoble LJ, Goates CY. 1990. Percutaneous absorption enhancement of an ionic molecule by ethanol-water systems in human skin. Pharm Res. 7(7):762–766.
- Kuswahyuning R, Roberts MS. 2014. Concentration dependency in nicotine skin penetration flux from aqueous solutions reflects vehicle induced changes in nicotine stratum corneum retention. Pharm Res. 31(6):1501–1511.
- Kwak S, Brief E, Langlais D, Kitson N, Lafleur M, Thewalt J. 2012. Ethanol perturbs lipid organization in models of stratum corneum membranes: an investigation combining differential scanning calorimetry, infrared and 2H NMR spectroscopy. Biochim Biophys Acta Biomembr. 1818(5):1410–1419.
- Lafforgue C. 2008. Lipides épidermiques et stratum corneum. Réalités Thérapeutiques en Dermato-Vénérologie. 179(2):6–8.
- Lane ME. 2013. Skin penetration enhancers. Int J Pharm. 447(1–2):12–21.
- Lane ME, Hadgraft J, Oliveira G, Vieira R, Mohammed D, Hirata K. 2012. Rational formulation design. Int J Cosmet Sci. 34(6):496–501.
- Larese F, Gianpietro A, Venier M, Maina G, Renzi N. 2007. In vitro percutaneous absorption of metal compounds. Toxicol Lett. 170(1):49–56.
- Larese Filon F, D’Agostin F, Crosera M, Adami G, Bovenzi M, Maina G. 2008. In vitro percutaneous absorption of chromium powder and the effect of skin cleanser. Toxicol in Vitro. 22(6):1562–1567.
- Larese Filon F, Fiorito A, Adami G, Barbieri P, Coceani N, Bussani R, Reisenhofer E. 1999. Skin absorption in vitro of glycol ethers. Int Arch Occup Environ Health. 72(7):480–484.
- Larese Filon F, Maina G, Adami G, Venier M, Coceani N, Bussani R, Massiccio M, Barbieri P, Spinelli P. 2004. In vitro percutaneous absorption of cobalt. Int Arch Occup Environ Health. 77(2):85–89.
- Lauer AC. 2005. Chapter 29, Percutaneous drug delivery to the hair follicle. In Bronaugh RL, Maibach HI, editors. Percutaneous absorption_ drugs, cosmetics, mechanisms, methodology. fourth edition ed. Boca Raton (FL): Taylor and Francis Group. p. 411–428.
- Lauer AC, Elder JT, Weiner ND. 1997. Evaluation of the hairless rat as a model for in vivo percutaneous absorption. J Pharm Sci. 86(1):13–18.
- Lee N, Jang DY, Lee DH, Jeong H, Nam KT, Choi DW, Lim KM. 2021. Local toxicity of biocides after direct and aerosol exposure on the human skin epidermis and airway tissue models. Toxics. 9(2):29.
- Lee SH, Jun SH, Yeom J, Park SG, Lee CK, Kang NG. 2018. Optical clearing agent reduces scattering of light by the stratum corneum and modulates the physical properties of corneocytes via hydration. Skin Res Technol. 24(3):371–378.
- Lee AY, Lee KH, Ko D-S, C WY. 2001. Constitutive expression and changes of cytochrome P450 isozymes mRNAs by vehicles (petrolatum, DMSO, ethanol) in rat skin using semi-quantitative RT-PCR. Korean J Physiol Pharmacol. 5(5):407–412.
- Levang AK, Zhao K, Singh J. 1999. Effect of ethanol/propylene glycol on the in vitro percutaneous absorption of aspirin, biophysical changes and macroscopic barrier properties of the skin. Int J Pharm. 181(2):255–263.
- Liu P, Higuchi WI, S WQ, Kurihara–Bergstrom T, Good WR. 1991. Quantitative evaluation of ethanol effects on diffusion and metabolism of beta-estradiol in hairless mouse skin. Pharm Res. 8(7):865–872.
- Lockley DJ, Howes D, Williams FM. 2004. Percutaneous penetration and metabolism of 2-butoxyethanol. Arch Toxicol. 78(11):617–628.
- Lu GW, Valiveti S, Spence J, Zhuang C, Robosky L, Wade K, Love A, Hu LY, Pole D, Mollan M. 2009. Comparison of artificial sebum with human and hamster sebum samples. Int J Pharm. 367(1-2):37–43.
- Luo K, Zeng D, Kang Y, Lin X, Sun N, Li C, Zhu M, Chen Z, Man YB, Li H. 2020. Dermal bioaccessibility and absorption of polycyclic aromatic hydrocarbons (PAHs) in indoor dust and its implication in risk assessment. Environ Pollut. 264:114829.
- Mak VW, Potts RO, Guy R. 1991. Does hydration affect intercellular lipid organization in the stratum corneum? Pharm Res. 8(8):1064–1065.
- Man MQ, Xin SJ, Song SP, Cho SY, Zhang XJ, Tu CX, Feingold KR, Elias PM. 2009. Variation of skin surface pH, sebum content and stratum corneum hydration with age and gender in a large Chinese population. Skin Pharmacol Physiol. 22(4):190–199.
- Manevski N, Swart P, Balavenkatraman KK, Bertschi B, Camenisch G, Kretz O, Schiller H, Walles M, Ling B, Wettstein R, et al. 2015. Phase II metabolism in human skin: skin explants show full coverage for glucuronidation, sulfation, N-acetylation, catechol methylation, and glutathione conjugation. Drug Metab Dispos. 43(1):126–139.
- Marquet F, Grandclaude MC, Ferrari E, Champmartin C. 2019. Capacity of an in vitro rat skin model to predict human dermal absorption: influences of aging and anatomical site. Toxicol in Vitro. 61:104623.
- Marquet F, Payan JP, Beydon D, Wathier L, Ferrari E, Grandclaude MC. 2015. Influence of water dilution on percutaneous absorption of N-vinyl-2-pyrrolidone in vivo and ex vivo in rats and ex vivo in humans. Arch Toxicol. 89(11):2007–2014.
- Martínez-Pla JJ, Martín-Biosca Y, Sagrado S, Villanueva-Camañas RM, Medina-Hernández MJ. 2004. Evaluation of the pH effect of formulations on the skin permeability of drugs by biopartitioning micellar chromatography. J Chromatogr A. 1047(2):255–262.
- Meidan VM, Bonner MC, Michniak BB. 2005. Transfollicular drug delivery-is it a reality? Int J Pharm. 306(1–2):1–14.
- Menon GK, Cleary GW, Lane ME. 2012. The structure and function of the stratum corneum. Int J Pharm. 435(1):3–9.
- Menon GK, Elias PM, Lee SH, Feingold KR. 1992. Localization of calcium in murine epidermis following disruption and repair of the permeability barrier. Cell Tissue Res. 270(3):503–512.
- Messager S, Hann AC, Goddard PA, Dettmar PW, Maillard JM. 2003. Assessment of skin viability: is it necessary to use different methodologies? Skin Res Technol. 9(4):321–330.
- Midander K, Julander A, Kettelarij J, Liden C. 2016. Testing in artificial sweat – is less more? Comparison of metal release in two different artificial sweat solutions. Regul Toxicol Pharmacol. 81:381–386.
- Midander K, Schenk L, Julander A. 2020. A novel approach to monitor skin permeation of metals in vitro. Regul Toxicol Pharmacol. 115:104693.
- Moghadam SH, Saliaj E, Wettig SD, Dong C, Ivanova MV, Huzil JT, Foldvari M. 2013. Effect of chemical permeation enhancers on stratum corneum barrier lipid organizational structure and interferon alpha permeability. Mol Pharm. 10(6):2248–2260.
- Moghimipour E, Salimi A, Zadeh BSM. 2013. Effect of the various solvents on the in vitro permeability of vitamin B12 through excised rat skin. Trop J Pharm Res. 12(5):671–677.
- Mohammed D, Matts PJ, Hadgraft J, Lane ME. 2014. In vitro-in vivo correlation in skin permeation. Pharm Res. 31(2):394–400.
- Morris SAV, Ananthapadmanabhan KP, Kasting GB. 2019. Anionic surfactant-induced changes in skin permeability. J Pharm Sci. 108(11):3640–3648.
- Mutalik S, Shetty PK, Kumar A, Kalra R, Parekh HS. 2014. Enhancement in deposition and permeation of 5-fluorouracil through human epidermis assisted by peptide dendrimers. Drug Deliv. 21(1):44–54.
- Naik A, Pechtold LARM, Potts RO, Guy RH. 1995. Mechanism of oleic acid-induced skin penetration enhancement in vivo in humans. J Control Release. 37(3):299–306.
- Neilson L, Mankus C, Thorne D, Jackson G, DeBay J, Meredith C. 2015. Development of an in vitro cytotoxicity model for aerosol exposure using 3D reconstructed human airway tissue; application for assessment of e-cigarette aerosol. Toxicol in Vitro. 29(7):1952–1962.
- OECD 2004a. Test no. 28: Guidance document for the conduct of skin absorption studies. Series on testing and assessment.
- OECD 2004b. Test no. 428: Skin absorption: in vitro method.
- OECD 2011. Test no. 156: Guidance notes on dermal absorption. Series on testing and assessment
- OECD 2019. Test no. 431: In vitro skin corrosion: reconstructed human epidermis (RHE) test method.
- Oesch F, Fabian E, Landsiedel R. 2018. Xenobiotica-metabolizing enzymes in the skin of rat, mouse, pig, guinea pig, man, and in human skin models. Arch Toxicol. 92(8):2411–2456.
- Oesch F, Fabian E, Oesch-Bartlomowicz B, Werner C, Landsiedel R. 2007. Drug-metabolizing enzymes in the skin of man, rat, and pig. Drug Metab Rev. 39(4):659–698.
- Oh SY, Fujii M, Takeda Y, Yoda K, Utoguchi N, Matsumoto M, Watanabe Y. 2002. The effect of ethanol on the simultaneous transport and metabolism of methyl p-hydroxybenzoate in excised skin of Yucatan micropig. Int J Pharm. 236(1-2):35–42.
- Ongpipattanakul B, Burnette RR, Potts RO, Francoeur ML. 1991. Evidence that oleic acid exists in a separate phase within stratum corneum lipids. Pharm Res. 8(3):350–354.
- Osman-Ponchet H, Gaborit A, Linget J-M, Wilson CE. 2017. Expression of drug transporters in the human skin: comparison in different species and models and its implication for drug development. Admet Dmpk. 5(2):75–84.
- Osseiran S, Cruz JD, Jeong S, Wang H, Fthenakis C, Evans CL. 2018. Characterizing stratum corneum structure, barrier function, and chemical content of human skin with coherent Raman scattering imaging. Biomed Opt Express. 9(12):6425–6443.
- Pannatier P, Jenner P, Testa B, Etter JC. 1978. The skin as a drug-metabolizing organ. Drug Metab Rev. 8(2):319–343.
- Patzelt A, Richter H, Buettemeyer R, Huber HJ, Blume-Peytavi U, Sterry W, Lademann J. 2008. Differential stripping demonstrates a significant reduction of the hair follicle reservoir in vitro compared to in vivo. Eur J Pharm Biopharm. 70(1):234–238.
- Pawar G, Abdallah MA, de Sáa EV, Harrad S. 2017. Dermal bioaccessibility of flame retardants from indoor dust and the influence of topically applied cosmetics. J Expo Sci Environ Epidemiol. 27(1):100–105.
- Praça FSG, Medina WSG, Eloy JO, Petrilli R, Campos PM, Ascenso A, Bentley MVLB. 2018. Evaluation of critical parameters for in vitro skin permeation and penetration studies using animal skin models. Eur J Pharm Sci. 111:121–132.
- Prost-Squarcioni C. 2006. Histologie de la peau et des follicules pileux [Histology of skin and hair follicles]. Med Sci. 22(2):131–137.
- Qiu H, McCall JW, Won Jun H. 1998. Formulation of topical insect repellent N,N-diethyl-m-toluamide (DEET): vehicle effects on DEET in vitro skin permeation. Int J Pharm. 163(1–2):167–176.
- Rajadhyaksha M, Gonzalez S, Zavislan JM, Anderson RR, Webb RH. 1999. In vivo confocal scanning laser microscopy of human skin II: advances in instrumentation and comparison with histology. J Invest Dermatol. 113(3):293–303.
- Rawlings A, Watkinson A, Rogers J, Mayo H, Scott I. 1994. Abnormalities in stratum corneum structure, lipid composition, and desmosome degradation in soap-induced winter xerosis. J Soc Cosmet Chem. 45:203–220.
- Ribaud C, Garson JC, Doucet J, Lévêque JL. 1994. Organization of stratum corneum lipids in relation to permeability: Influence of sodium lauryl sulfate and preheating. Pharm Res. 11(10):1414–1418.
- Rissmann R, Oudshoorn MH, Hennink WE, Ponec M, Bouwstra JA. 2009. Skin barrier disruption by acetone: observations in a hairless mouse skin model. Arch Dermatol Res. 301(8):609–613.
- Riviere JE. 2005. Chapter 10, Percutaneous absorption of chemical mixtures. In Bronaugh RL, Maibach HI, editors. Percutaneous absorption_ drugs, cosmetics, mechanisms, methodology. Boca Raton (FL): Taylor and Francis Group p. 155–164.
- Roberts MS, Cross SE, Pellett MA. 2002. Chapter 4, Skin transport. In Walters KA, editor. Dermatological and transdermal formulations. Walters KA ed ed. New York. Basel: Marcel Dekker.
- Rode B, Ivens U, Serup J. 2000. Degreasing method for the seborrheic areas with respect to regaining sebum excretion rate to casual level. Skin Res Technol. 6(2):92–97.
- Rosado C, Cross SE, Pugh WJ, Roberts MS, Hadgraft J. 2003. Effect of vehicle pretreatment on the flux, retention, and diffusion of topically applied penetrants in vitro. Pharm Res. 20(9):1502–1507.
- Rothe H, Obringer C, Manwaring J, Avci C, Wargniez W, Eilstein J, Hewitt N, Cubberley R, Duplan H, Lange D, et al. 2017. Comparison of protocols measuring diffusion and partition coefficients in the stratum corneum. J Appl Toxicol. 37(7):806–816.
- Rush AK, Nash JF, Smith Iii ED, Kasting GB. 2019. Formulation and artificial sebum effects on the percutaneous absorption of zinc pyrithione through excised human skin. Skin Pharmacol Physiol. 32(4):224–234.
- Salimi A, Moghimipour E, Rahman F. 2015. Effects of the various solvents on the in vitro permeability of indomethacin through whole abdominal rat skin. ARRB. 5(4):335–346.
- Samaras EG, Riviere JE, Ghafourian T. 2012. The effect of formulations and experimental conditions on in vitro human skin permeation—data from updated EDETOX database. Int J Pharm. 434(1–2):280–291.
- Sand M, Gambichler T, Moussa G, Bechara FG, Sand D, Altmeyer P, Hoffmann K. 2006. Evaluation of the epidermal refractive index measured by optical coherence tomography. Skin Res Technol. 12(2):114–118.
- SCCS 2010. Basic criteria for the in vitro assessment of dermal absorption of cosmetic ingredients. Vol. SCCS/1358/10.
- Scheuplein R, Ross L. 1970. Effects of surfactants and solvents on the permeability of epidermis. J Soc Cosmet Chem. 21:853–873.
- Scheuplein RJ, Ross LW. 1974. Mechanism of percutaneous absorption. V. Percutaneous absorption of solvent deposited solids. J Invest Dermatol. 62(4):353–360.
- Schneider D, Dennerlein K, Goen T, Schaller KH, Drexler H, Korinth G. 2016. Influence of artificial sebum on the dermal absorption of chemicals in excised human skin: a proof-of-concept study. Toxicol in Vitro. 33:23–28.
- Schurer NY, Elias PM. 1991. The biochemistry and function of stratum corneum lipids. In Elias PM, editor. Advances in lipid research. San Diego (CA): Academic Press Inc.; p. 27–56.
- Shah DK, Khandavilli S, Panchagnula R. 2008. Alteration of skin hydration and its barrier function by vehicle and permeation enhancers: a study using TGA, FTIR, TEWL and drug permeation as markers. Methods Find Exp Clin Pharmacol. 30(7):499–512.
- Shetage SS, Traynor MJ, Brown MB, Chilcott RP. 2014. Chapter 4, Collection and characterisation of residual skin surface components obtained from sebum, sweat and epidermal lipids. In: Chilcott R, Brain KR, editors. Advances in dermatological sciences. Cambridge: The royal society of chemistry; p. 26–36.
- Smith EW, Surber C, Maibach HI. 2005. Chapter 49, Topical dermatological vehicles: a holistic approach. In Bronaugh RL, Maibach HI, editors. Percutaneous absorption_ drugs, cosmetics, mechanisms, methodology. Fourth Edition ed. Boca Raton (FL): Taylor and Francis Group p. 655–662.
- Stauber JL, Florence TM, Gulson BL, Dale LS. 1994. Percutaneous absorption of inorganic lead compounds. Sci Total Environ. 145(1–2):55–70.
- Stefaniak AB, Harvey CJ. 2006. Dissolution of materials in artificial skin surface film liquids. Toxicol in Vitro. 20(8):1265–1283.
- Stefaniak AB, Harvey CJ, Wertz PW. 2010. Formulation and stability of a novel artificial sebum under conditions of storage and use. Int J Cosmet Sci. 32(5):347–355.
- Sterne GD, Titley OG, Christie JL. 2000. A qualitative histological assessment of various storage conditions on short term preservation of human split skin grafts. Br J Plast Surg. 53(4):331–336.
- Stinchcomb AL, Pirot F, Touraille GD, Bunge AL, Guy RH. 1999. Chemical uptake into human stratum corneum in vivo from volatile and non-volatile solvents. Pharm Res. 16(8):1288–1293.
- Suhonen TM, Bouwstra JA, Urtti A. 1999. Chemical enhancement of percutaneous absorption in relation to stratum corneum structural alterations. J Control Release. 59(2):149–161.
- Sullivan KM, Aggarwal M, Akins JM, Fabian E, Heylings JR, Raabe H, Shah PPV, Wiemann C, Peffer R. 2017. Dermal absorption for pesticide health risk assessment: harmonization of study design and data reporting for North American Regulatory Submissions. Regul Toxicol Pharmacol. 90:197–205.
- Sznitowska M, Janicki S, Baczek A. 2001. Studies on the effect of pH on the lipoidal route of penetration across stratum corneum. J Control Release. 76(3):327–335.
- Thomas NS, Panchagnula R. 2003. Transdermal delivery of zidovudine: effect of vehicles on permeation across rat skin and their mechanism of action. Eur J Pharm Sci. 18(1):71–79.
- Traynor MJ, Wilkinson SC, Williams FM. 2007. The influence of water mixtures on the dermal absorption of glycol ethers. Toxicol Appl Pharmacol. 218(2):128–134.
- Trommer H, Neubert RH. 2006. Overcoming the stratum corneum: the modulation of skin penetration. A review. Skin Pharmacol Physiol. 19(2):106–121.
- Tsai JC, Hung PL, Sheu HM. 2001. Molecular weight dependence of polyethylene glycol penetration across acetone-disrupted permeability barrier. Arch Dermatol Res. 293(6):302–307.
- Tsai JC, Lu CC, Lin MK, Guo JW, Sheu HM. 2012. Effects of sebum on drug transport across the human stratum corneum in vivo. Skin Pharmacol Physiol. 25(3):124–132.
- Tsai JC, Sheu HM, Hung PL, Cheng CL. 2001. Effect of barrier disruption by acetone treatment on the permeability of compounds with various lipophilicities: implications for the permeability of compromised skin. J Pharm Sci. 90(9):1242–1254.
- Turhan-Haktanir N, Dilek FH, Koken G, Demir Y, Yilmaz G. 2011. Evaluation of amniotic fluid as a skin graft storage media compared with RPMI and saline. Burns. 37(4):652–655.
- USEPA. 2004. In vitro dermal absorption rate testing of certain chemical of interest to the occupational safety and health administration; final rule. Fed Regist. 69(80):22402–22441.
- Valiveti S, Lu GW. 2007. Diffusion properties of model compounds in artificial sebum. Int J Pharm. 345(1–2):88–94.
- Valiveti S, Wesley J, Lu GW. 2008. Investigation of drug partition property in artificial sebum. Int J Pharm. 346(1–2):10–16.
- Van Hal DA, Jeremiasse E, Junginger HE, Spies F, Bouwstra JA. 1996. Structure of fully hydrated human stratum corneum: a freeze-fracture electron microscopy study. J Invest Dermatol. 106(1):89–95.
- Van Hemmen JJ. 2004. Dermal exposure to chemicals. Ann Occup Hyg. 48(3):183–185.
- Van Lierde V, Chery CC, Roche N, Monstrey S, Moens L, Vanhaecke F. 2006. In vitro permeation of chromium species through porcine and human skin as determined by capillary electrophoresis-inductively coupled plasma-sector field mass spectrometry. Anal Bioanal Chem. 384(2):378–384.
- Venier M, Adami G, Larese F, Maina G, Renzi N. 2004. Percutaneous absorption of 5 glycol ethers through human skin in vitro. Toxicol in Vitro. 18(5):665–671.
- Verdier-Sévrain S, Bonté F. 2007. Skin hydration: a review on its molecular mechanisms. J Cosmet Dermatol. 6(2):75–82.
- Wang SM, Chang HY, Tsai JC, Lin WC, Shih TS, Tsai PJ. 2009. Skin penetrating abilities and reservoir effects of neat DMF and DMF/water mixtures. Sci Total Environ. 407(19):5229–5234.
- Watkinson AC, Herkenne C, Guy RH, Hadgraft J, Oliveira G, Lane ME. 2009. Influence of ethanol on the solubility, ionization and permeation characteristics of ibuprofen in silicone and human skin. Skin Pharmacol Physiol. 22(1):15–21.
- Wertz PW. 2004. Stratum corneum lipids and water. Exog Dermatol. 3(2):53–56.
- Wertz PW. 2009. Human synthetic sebum formulation and stability under conditions of use and storage. Int J Cosmet Sci. 31(1):21–25.
- Williams AC, Barry BW. 2012. Penetration enhancers. Adv Drug Deliv Rev. 64:128–137.
- Williams RL, Reifenrath WG, Krieger RI. 2005. Artificial sweat enhances dermal transfer of chlorpyrifos from treated nylon carpet fibers. J Environ Sci Health B. 40(4):535–543.
- Yourick JJ, Bronaugh RL. 2000. Percutaneous penetration and metabolism of 2-nitro-p-phenylenediamine in human and fuzzy rat skin. Toxicol Appl Pharmacol. 166(1):13–23.
- Zhang A, Jung EC, Zhu H, Zou Y, Hui X, Maibach H. 2017. Vehicle effects on human stratum corneum absorption and skin penetration. Toxicol Ind Health. 33(5):416–425.
- Zhang Q, Li P, Roberts MS. 2011. Maximum transepidermal flux for similar size phenolic compounds is enhanced by solvent uptake into the skin. J Control Release. 154(1):50–57.
- Zhang SL, Meyers CL, Subramanyan K, Hancewicz TM. 2005. Near infrared imaging for measuring and visualizing skin hydration. A comparison with visual assessment and electrical methods. J Biomed Opt. 10(3):031107.
- Zhou Y, Banga AK. 2011. Enhanced delivery of cosmeceuticals by microdermabrasion. J Cosmet Dermatol. 10(3):179–184.