Abstract
Long-term inhalation exposure to manganese (Mn) metal or its inorganic compounds can result in manganism or subclinical neurofunctional deficits. Studies have described affected workers in Mn dioxide mining, Mn-containing ore crushing and milling facilities, manufacturing of dry-cell batteries, Mn steel and alloy production plants, and in welders. The objective of this study was to critically review existing evidence on the reliability of potential biomarkers of Mn exposure, specifically the relationship between inhalation exposure to Mn particulates in different occupational settings and Mn concentrations in blood and other biological fluids and tissues, with a particular focus on whole blood as a potentially useful medium for measuring internal tissue dose. We also examined available evidence on the relationship between Mn levels in blood and adverse clinical and subclinical neurotoxic outcomes. Three bibliographic databases were searched for relevant studies and identified references were screened by two independent reviewers. Of the 6338 unique references identified, 76 articles were retained for data abstraction. Findings indicate that the relationships between Mn in blood and both external Mn exposure indices and neurofunctional impairments are limited and inconsistent. Different sources of exposure to Mn compounds, heterogeneity in the methodological approaches, and inadequate reporting of essential information limited direct comparison of the reported findings. Among the Mn-exposure biomarkers considered in this review – including biomarkers in blood, plasma, serum, erythrocytes, urine, bone, toenails, fingernails, hair, saliva – biomarkers in whole blood may provide to be most useful in Mn biomonitoring and risk assessment.
1. Introduction
Manganese (Mn) is an essential element in the human body, where its deficiency or excess can lead to adverse health effects. Mn absorption, retention, and excretion are controlled by homeostatic mechanisms and are highly interrelated. Regulation of Mn in blood, different organs and tissues is affected by Mn levels from dietary exposure (the main source of Mn supply in humans) and by individual variability in homeostatic regulations [(Freeland-Graves et al. Citation1988); see Andersen et al. (Citation1999), for a detailed discussion of Mn toxicokinetics)]. Despite the fact that Mn uptake following inhalation exposure represents less than 1% of the total daily intake, in occupational settings, inhalation exposure represents the most efficient route of transport of Mn to the brain. Inhaled Mn bypasses the protective role of the liver to enter the systemic circulation, it might enter the brain via the olfactory system, bypassing the protective role of the blood-brain barrier. In addition, occupational aerosol may contain substantial levels of non-respirable Mn particles (size from 10 to 100 µm) and Mn uptake into blood can occurs via the gastrointestinal tract (due to a mucociliary clearance from the tracheobronchial airways) (Long et al. Citation2014).
Long-term occupational inhalation exposure to high levels of insoluble Mn oxide dust or fume particulates may lead to an excessive Mn accumulation in the basal ganglia of the brain and the development of a clinical condition called manganism (ATSDR Citation2012). Classical fully developed manganism is characterized by tremor, dysdiadochokinesia, ataxic gait, dystonia, and cogwheel phenomenon (postural tremor) (ATSDR Citation2012). “Cock walk” is generally considered as a clinical sign that is specific to Mn neurotoxicity (Calne et al. Citation1994; Jankovic Citation2005). It has been suggested that neurological symptoms may improve in some cases if a patient is removed from Mn exposure at an early stage (e.g. cognitive, (Ky et al. Citation1992)); however, clinical progression of motor functions impairment tends to persist and may even worsen after cessation of exposure (Huang et al. Citation1998). Clinical signs and symptoms of manganism are similar to Parkinson’s Disease (PD) with some clinical, pharmacological, and imaging differences (see Calne et al. (Citation1994) for a detailed discussion of the differences).
The precise mechanism of Mn neurotoxicity is not known. However, available data suggest that the basic mechanism is multifactorial, and involves oxidative stress induced by iron (Fe) and the direct interaction of Mn with the mitochondria in the terminal part of the dopaminergic nerves, leading to selective mitochondrial dysfunction (Verity Citation1999). The effects of Mn excess on the central nervous system (CNS) are dependent upon the Mn dose and duration of Mn exposure, the age at which exposure occurs, and the nutritional status of the individual, particularly with respect to Fe (Aschner et al. Citation2009). Severity of clinical effects also depends on physical and chemical characteristics, composition, and bioavailability of Mn compounds. Despite extensive research on occupational exposure to insoluble and soluble Mn compounds in different settings, the bioavailability of Mn in airborne particulates in workplaces has not been adequately investigated (Hammer et al. Citation2021). The overall pathways and severity of adverse health outcomes following long-term inhalation exposure to Mn is described by Mattison et al. (Citation2017).
Shilnikova et al. (Citation2022) reviewed biomarkers of environmental exposure to Mn, within the context of the risk characterization framework on the role of exposure biomarkers in environmental biomonitoring. The framework and presented in the current paper, adapted from McClellan (Citation2020), describe the exposure to dose to effects continuum, and provide a basis for situating the role of biomarkers of Mn exposure and effects within an expanded risk assessment paradigm depicted in .
Figure 1. An expanded risk characterization paradigm illustrating the role of biomarkers of exposure, effect, and susceptibility (adapted from McClellan (Citation2020)).
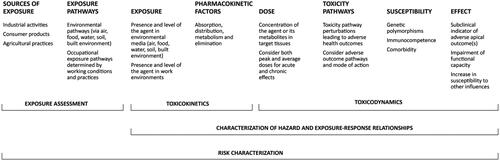
Exposure biomarkers typically reflects the dose of the agent of interest in critical target tissues, allowing for absorption, distribution, metabolism, and elimination of the agent, with a good exposure biomarker being correlated with external sources of exposure to the agent. Effect biomarkers typically reflect early biological changes that are correlated with an adverse, clinically detectable, apical outcome (see Farrell et al. (Citation2022), for further discussion of homeostatic, adaptive, and toxic responses to essential elements, such as Mn, which can lead to toxicity either through excess or deficiency). Although outside the scope of the present paper, biomarkers of susceptibility are biological properties of the host, such as genetic polymorphisms, that may render the host more susceptible to the developing an adverse outcome following exposure to the agent of interest (see Zeise et al. (Citation2013) for a detailed discussion of host susceptibility factors).
The present review focuses on potential biomarkers of Mn occupational exposure and adverse effects on CNS. Hoet and Roels (Citation2015) cite the following three desirable properties of an occupational exposure biomarker for Mn:
the biomarker should be able to distinguish exposed and non-exposed workers;
the biomarker demonstrates a dose-related association with external exposure; and
the biomarker should demonstrate a dose-related association with adverse effect(s) on the CNS.
The second of these three properties is a requirement of any good exposure marker within the context of the risk characterization paradigm in . The first and third properties are additional desirable properties that would enhance the use of a biomarker of occupational Mn exposure in risk assessment applications: being able to distinguish exposed and non-exposed workers (Property 1) would be helpful both in epidemiological risk assessment and in occupational health monitoring; a dose-related association with an adverse CNS effect(s) would further enhance the relevance of the exposure biomarker for both clinical diagnosis and risk assessment purposes.
Because whole blood Mn (Mn-B) is traditionally used in occupational clinics for identification of workers with excessive exposure and those at risk of developing of clinical Mn-related neurofunctional impairments (IRSST Citation2005; Myers et al. Citation2009), a primary focus of the current review is on Mn-B. This review also focuses on evidence of the associations between Mn-B with airborne Mn (Mn-A) and with clinical or subclinical neurofunctional outcomes. We also briefly summarize primary research on Mn in other biological fluids and tissues, including urine (Mn-U), blood components (plasma (Mn-P), serum (Mn-Se), erythrocytes (Mn-E), bone (Mn-Bn), nails (toenails (Mn-Tn), fingernails (Mn-Fn), hair (Mn-H), and saliva (Mn-Sa).
2. Methods
Three electronic literature databases, Medline, Embase, and Toxline, were searched to identify potentially relevant studies from inception to January 19, 2022. Database-specific search strategies were developed using keywords and MeSH terms related to “manganese” and “biomarkers”. The search was designed to exclude animal or in vitro studies. Detailed search strategies are presented in Supplemental Material 1 (Tables S1.1–S1.3). References captured by the search were imported into EndNote and duplicates were removed. Grey literature sources were also searched for additional publications that may be relevant.
Table 1. Summary of evidence from occupational exposure studies on Mn in whole blood as a useful biomarker of airborne Mn exposure.
Identified references were screened using a two-stage process where titles and abstracts were screened (Level 1) followed by full-text screening (Level 2) of all references advanced from the first stage. Screening was performed independently by two reviewers (NK, NS) based on predefined eligibility criteria (Supplemental Material 1, Table S1.4). Discrepancies in screening between the reviewers were resolved by consensus. The screening process was performed using DistillerSR (Evidence Partners, Ottawa, Canada) systematic review software.
Peer-reviewed original human studies (cohort, case-control, or clinical case reports), meta-analyses, and systematic reviews with or without meta-analysis published in English were eligible for inclusion. News articles, literature reviews, conference abstracts, and editorials were excluded. Included studies had to have assessed Mn-B among workers exposed through inhalation to inorganic Mn compounds or Mn-containing welding fumes under defined exposure scenarios (e.g. miners, dry-cell batteries and Mn oxides and salts production workers, Fe-Mn and Si-Mn alloys production and smelters workers, and welders). Studies assessing Mn dietary or dermal exposures, inhalation exposure from organic Mn compounds or Mn-containing nanoparticles, modeled exposure studies, exposure from parenteral administration of Mn substances, oral intake of Mn-containing pharmaceuticals or nutritional supplements, and other irrelevant exposure scenarios (e.g. environmental exposure from drinking water or from incinerator facilities) were excluded. Only neurotoxic adverse health endpoints were eligible for inclusion into review, while other health endpoints were excluded. Original studies examining a correlation between Mn-B and Mn content in brain using MRI or other imaging techniques were not included as they were reviewed in an accompanying paper. No restriction on year of publication was applied.
Eligible studies had to meet the following additional criteria: parallel investigation of a non-exposed control group or background Mn levels in the same group of workers, the study had to include an analysis of the association between Mn-B and Mn-A exposure or neurofunctional outcomes, available data on Mn-A concentration in the occupational air, as well as control of major confounders. For published studies on subjects involved in a litigation process, only data on Mn-B exposure but not on the effects were included, due to a risk of reporting (response) bias.
For qualitative assessment of the quality of evidence in this critical review, the following general criteria were considered: the proper selection and characterization of the exposed and control groups; adequate characterization of exposure; valid method for observing an effect; proper consideration of confounding factors; a reasonable statistical reliability to justify the conclusion; and key limitations of the research (reported by the authors and identified by reviewers) which may have biased the findings of the included studies in the manuscript. In this critical review, the risk of bias was not assessed in a standardized manner. Existing systematic reviews and critical reviews published by experts in the areas of Mn epidemiology and toxicology were incorporated to consolidate all available research findings and to emphasize how the present research contributes to knowledge in the area of potential biomarkers of Mn exposure.
Custom data abstraction forms were developed to capture key details of each primary study (see Supplemental Material 2–6). Abstracted information was summarized in evidence tables to include main findings on potential biomarkers of exposure in workers exposed to Mn particulates and Mn welding fumes in different exposure settings. Summary tables focus on studies of whole blood, with studies on biomarkers of exposure in biological fluids and tissues other than whole blood presented in separate tables. Data abstraction was conducted by one reviewer (NK).
3. Findings
3.1. Search results
A total of 8892 references were identified from the electronic database searches. Following the removal of duplicates, 6338 references were screened by title and abstract (Level 1) for relevance, where 594 references were retained for full-text (Level 2) screening. Seventy-six studies met the eligibility criteria and underwent data abstraction ().
Figure 2. Study flow diagram of records identified and screened [Adapted from Moher et al. (Citation2009)].
![Figure 2. Study flow diagram of records identified and screened [Adapted from Moher et al. (Citation2009)].](/cms/asset/7406e1f3-1b27-4b18-85b0-4a6ee262cf82/itxc_a_2128718_f0002_c.jpg)
3.2. Results
Reviewed studies examined workers exposed to Mn in several occupational settings and different exposure scenarios including exposure to insoluble Mn-containing dust in mines and Mn ore milling facilities, in dry-cell batteries production plants, Mn oxides and soluble salts production plants, at ferromanganese (Fe-Mn) and silico-manganese (Si-Mn) alloy production manufactures and smelters, and to Mn-containing fumes during welding, mainly in industrial, manufacturing, and construction settings.
Initially, manganism was reported in miners exposed to high concentration of Mn dust mainly as insoluble Mn oxides particulates (Rodier Citation1955). More recently, a significant decrease in Mn exposure levels was observed at occupational settings due to the significant improvements in the technological processes and/or ventilation systems. For example, In mines, the highest Mn exposure levels decreased from 1955 to 2002 by 2–3 times (from 250–450 to 62–114 mg/m3) (Rodier Citation1955; Boojar et al. Citation2002); in dry cell production industry, exposure to Mn decreased by 53 times (from 6.8–42.2 to 0.137–0.794 mg/m3) in 2001 compared to 1971 (Emara et al. Citation1971; Dietz et al. Citation2001); in Fe-Mn alloy production plants by 8.87 times (from 0.12–13.3 to 0.005–1.5 mg/m3) in 1999 compared to 1973 (Smyth et al. Citation1973; Lucchini et al. Citation1999) in Fe-Mn alloy smelters, Mn exposure decreased by 43.45 times (from 0.66–1.26 to 0.002–0.029 mg/m3) from 2007 to 2016 (Jiang et al. Citation2007; Hassani et al. Citation2016). From 1981 to 1995, an average (geometric mean [GM]) Mn concentration in total dust in the Italian Fe-Mn/Si-Mn alloy production plant at the furnace area decreased by 9.65 times (from 1597.03 to 165.47 µg/m3), at the casting area by 2.26 times (from 151.53 to 67.05 µg/m3) and in other open spaces of the plant by 47.17 times (from 570 to 12.09 µg/m3) (Lucchini et al. Citation1999). However, only small decrease in Mn-containing fume exposure levels occurred in welding industry (from 0.44–2.6 to 0.007–2.32 mg/m3) from 1981 to 2006, respectively (Chandra et al. Citation1981; Ellingsen et al. Citation2006). Currently, welders are still exposed to Mn concentrations in Mn-containing fumes often exceeded a level of 0.02 mg/m3 (see Supplemental Material 7) (Mehrifar et al. Citation2020). The reporting of a wide range of measured values (as total Mn, respirable or inhalable fractions, Cumulative Exposure Index (Mn-CEI)), which have not always been taken into account in previous analyses, or as a range of detected Mn concentrations), different types of sampling (personal, stationary), different timing and duration of sampling (before shift or after shift, in the beginning, end or in the middle of working week; full shift, half-shift, few hours), different methodologies for cumulative exposure calculation, lack of details on sampling technique (e.g. type of sampler heads used during air sampling) limited a direct comparison of reported values.
3.2.1. Mn-B as an internal marker of external exposure and an indicator of neurofunctional impairment
Several studies included in this review examined concentrations of Mn in whole blood as a marker of external exposure or neurofunctional impairments in various settings among populations from United States, Germany, Belgium, China, Norway, Poland, Finland, Northern Thailand, Canada, and Russia. These included 3 studies in Mn mines and Mn ore milling factories, 3 studies in dry-cell batteries production plants, 3 studies in Mn oxide and Mn salts production plants, 20 studies on workers in active and/or historic Fe-Mn and Si-Mn alloy production manufactures, and 24 studies among welders in different industrial and manufacturing settings.
These most informative studies that assessed the relationship between Mn-B with measures of Mn-A dust or fume or with adverse neurofunctional outcomes are described below. Details regarding these studies could be found in Supplemental Materials 2–6.
3.2.1.1. Mn-B in workers at Mn mines and Mn ore milling facilities
At the Mn ore milling factory, workers are exposed to insoluble Mn oxides dust particles during feeding of Mn ore into the ball mill and especially bagging of the milled Mn dust in a very dusty environment. Miners are exposed primarily to insoluble Mn oxides and to Mn silicates during tunnel digging, drilling, mixing, and transporting of the raw materials in the mine (Boojar et al. Citation2002). Three studies were conducted on workers exposed to insoluble Mn dust particles at mine and Mn ore milling factories (Gan et al. Citation1988; Chia et al. Citation1993; Boojar et al. Citation2002). More details regarding these studies could be found in Supplemental Material 2, Table S2.1.
In Singapore, Chia et al. (Citation1993) compared 17 workers exposed to Mn oxide dust (mean level 1.59 mg Mn/m3, as total dust) for an average (geometric mean) of 7.4 years (range: 1–14 years) at Mn ore milling factories to 17 unexposed (control) subjects. Mn-B concentrations in Mn-exposed workers (mean 25.3 (range 15–92.5) μg/L) were significantly higher than those values in control subjects (mean 23.3 (range 17.3–30.1) μg/L). No significant relationships were reported between Mn-B and Mn-A. However, the exposed group had a significantly higher prevalence of subjective symptoms such as insomnia and profuse sweating and a higher prevalence of decreased scores in several neurobehavioral tests including finger tapping, digit symbol, and pursuit aiming. No significant correlation was observed between the neurobehavior test scores and the Mn-B in each worker. The strongest correlation was between Mn-B and the digit symbol test. Chia et al. (Citation1993) suggested that because workers were exposed mostly to non-respirable dust particulates, total dust should be used to control exposure levels. One of the limitations of this study is a small sample size. In a more recent study in Singapore, Gan et al. (Citation1988) conducted a study on 22 workers from Mn ore milling plants and 58 control subjects to determine the usefulness of Mn-B estimations as biological indicators of exposure. Workers were exposed to Mn oxide dust (as total dust) from 3 months to more than 3 years. Mn-B concentrations in Mn-exposed workers from the milling plants were significantly higher (p < 0.001) than those values in control subjects. Mn-A concentrations correlated significantly with Mn-B concentrations (r = 0.69). At the TLV of 5 mg/m3, the corresponding Mn-B concentration was about 30 µg/L. Mn-B concentrations were not related to age, duration of exposure and type of work, ethnic group, or smoking status. In Iran, Boojar et al. (Citation2002) examined lung function and respiratory symptoms in 145 Mn mine workers and 65 unexposed subjects. The workers were categorized into three groups by mean Mn concentrations at workplaces (as Mn-A total or Mn-A respirable fraction) and by duration of exposure: at the beginning of work, in the following 4.3 years, and at 7.5 years of exposure. It was found that workers exposed to the highest levels of Mn-A for 4.3-7.5 years had higher Mn-B concentrations (mean 137.2 and 167.2 µg/L, respectively) compared to the new workers (mean 17.3 µg/L). Groups with the highest Mn-A exposure had significantly higher Mn-B compared to control subjects. It was also found that Mn-B levels increased with duration of exposure. The association between Mn-B and Mn-A exposure was not studied. The authors conducted air sampling during half of the work shift only, time lag between Mn-A sampling and Mn-B sampling, response rate at stage 2 and 3, and information on use of personal protection equipment were not reported.
Summary
The limited inconsistent evidence and studies limitations do not allow a conclusion regarding the use of Mn-B as a biomarker of external exposure in Mn oxide-exposed miners or Mn ore milling plants workers. Reported results highlighted importance of sufficient sample size for statistical analysis and consideration of potential effects on the lungs in clinical practice and in research studies on insoluble Mn oxide dust-exposed miners. Mn mining workers have a high potential for pulmonary problems because of the higher accumulation of Mn in the lungs that affects ventilatory capacity and lung functions. Findings from epidemiological studies in humans exposed to high concentrations of insoluble Mn oxide particulates indicate adverse effects on the respiratory system at levels below 1 mg/m3, whereas studies of effects on the CNS below this level can be equivocal or negative (WHO Citation1981; US EPA Citation1984).
3.2.1.2. Mn-B in workers employed at dry cell battery production facilities
The main operations in the manufacture of dry-cell acid or alkaline batteries consist of mixing of the dry ingredients (e.g. Mn dioxide, potassium hydroxide, and zinc, as the most common types of filling substances) and compressing the mixture into the “cake”. This “cake” is placed in zinc cans and carbon rods, then sealed and capped. The mixers are primarily exposed to Mn oxide dry powder and workers in the compressing section are exposed to moist Mn oxide mixture. This suggests that levels of exposure to Mn might be different and significantly higher in mixers.
Two studies were conducted on workers employed in a dry-cell battery manufacturing plant in Germany (Bader et al. Citation1999; Dietz et al. Citation2001) and one study on workers employed in a dry alkaline battery plant in Belgium (Roels et al. Citation1992). More details regarding these studies could be found in Supplemental Material 3 (Table S3.1).
In Belgium, Roels et al. (Citation1992) compared 92 male workers in a dry alkaline battery plant and 101 age- and area-matched controls for their performance in a battery of neurobehavioral tests. Mn workers were exposed to 215 μg Mn/m3 (respirable dust) and 948 μg Mn/m3 (total dust). Mn concentrations in total and respirable dusts were measured with personal sampling in the breathing zones, exceeded 4–5 h in 80% of the measurements and was carried out during worker’s usual job tasks. Blood sampling was conducted on the day of the clinical examination. Mn-B concentrations were significantly higher in the exposed group (8.1 µg/L, the geometric mean) than in the control group (6.8 µg/L, the geometric mean) (p < 0.001). At the individual level, no correlation was found between Mn-B and duration of exposure or Mn-A (total dust), Mn-A (respirable fraction) or Mn-CEI (total dust) and Mn-CEI (respirable fraction). On a group basis, no relationship was reported between blood Mn and measures of simple reaction time, eye-hand coordination, and hand steadiness, despite it was a significantly poorer performance in Mn-exposed workers than in the comparison group. However, an association was observed between external cumulative (integrated) Mn exposure and neurofunctional impairment. Using logistic regression analysis, it was shown that an increased risk of peripheral tremor exists when the lifetime integrated exposure to Mn dust exceeds 3.575 or 730 μg Mn/m3 × year for total and respirable dust, respectively.
Bader et al. (Citation1999) examined 100 workers from three dry cell battery plants and 17 control subjects. The workers were divided into three subgroups based on their tasks in the plant: the black area (n = 39), the grey area (n = 22), and the white area (n = 39). The mean air concentrations of Mn in the three areas were 4 μg/m3, 40 μg/m3, and 400 μg/m3, respectively. The mean Mn concentrations in the blood were 12.2 (SD: 4.8) μg/L among workers and 7.5 (SD: 2.7) μg/L among the controls. There were significant differences in the levels of Mn in the blood between the highly exposed workers and both the controls and the low-exposure group, as well as between the total number of Mn-exposed workers and the controls (p < 0.05, U-test in all cases). A significant correlation was determined between Mn-B and Mn-A (inhalable particles) in group-based calculations but not with individual levels. Dietz et al. (Citation2001) examined a group of 11 workers with a long-term exposure (mean exposure duration >10 years) to high levels of MnO2 dust and 11 age-matched workers from the same factory with similar socioeconomic status (reference group). Mean Mn-A concentrations were 387 μg/m3 and 10 μg/m3 in the exposed and in the reference group, respectively. Levels of Mn-B of the exposed workers (mean 14.8, range 9.1–19.6 μg/L) were significantly higher than those in control subjects (mean 11.0, range 3.9–22.5 µg/L). No significant relationships were found between Mn-B and Mn-A. Only limited details on study design and methods used for occupational air and biological monitoring were provided by Dietz et al. (Citation2001); however, the authors cited Bader et al. (Citation1999) study for more information. No significant differences in neurofunctional test scores between exposed and control groups were found and association between Mn-B and test results was not studied.
Summary
Three studies analyzed the association between Mn-B and Mn-A or neurofunctional performance, all of which reported a statistically significant group difference between exposed workers compared to controls (Roels et al. Citation1992; Bader et al. Citation1999; Dietz et al. Citation2001). No association was reported between Mn-B and Mn-A levels in one study (Dietz et al. Citation2001), no relationships between Mn-B levels and abnormal neurological performance were reported by Roels et al. (Citation1992). Overall, reported data on Mn-B as a biomarker of exposure or as a risk factor for neurofunctional impairment are limited and inconsistent.
3.2.1.3. Mn-B in workers of Mn oxides and Mn salts production plant
The Mn workers from plants produces Mn oxides (Mn3O4, MnO2) and Mn salts (sulfate, carbonate, nitrate) using concentrated ores. Two studies have examined the suitability of Mn as a potential biomarker of occupational Mn exposure and adverse health effects in Mn-exposed workers at these plants (Roels, Lauwerys, Buchet, et al. Citation1987; Roels, Lauwerys, Genet, et al. Citation1987). A brief description of these studies provided below, and more details of these studies can be found in Supplemental Material 4 (Table S4.1).
In a cross-sectional study, Roels, Lauwerys, Genet, et al. (Citation1987) examined 141 workers in a Mn oxide and salt producing plant and 104 matched control subjects recruited from a chemical plant located in the same area. The total Mn-A concentration was determined in the breathing zone of workers using personal air samplers during the full shift. The difference in Mn-B levels between the exposed and control group was statistically significant and on average two-fold between groups. Levels of Mn-B collected before and after the work shift from Mn-exposed workers were not significantly different; Mn-A was measured on the same day, but no correlation was seen between Mn-B and Mn-A in these workers. Analysis of individual data of all 141 exposed workers found no correlation between Mn-B in and Mn-A level or duration of Mn exposure. Analysis of data grouped by workplace found no correlation between current Mn-A and Mn-B. Mn-B was not correlated with exposure duration in four groups based on duration of exposure (<5, 5–9, 10–14, ≥15 years). However, Mn-B was significantly correlated with integrated past exposure subjectively estimated by the chief foreman and graded into six categories from 1+ (low exposure) to 6+ (heavy exposure).
In another cross-sectional study, Roels, Lauwerys, Buchet, et al. (Citation1987) examined 141 male workers in a Mn oxide and salt producing plant, and 104 matched control subjects. Blood samples were collected on the day of the clinical examination which included a standard neurological examination and psychomotor tests: simple reaction time, short-term memory, and hand tremor. For dose-response analyses, the Mn-exposed workers were classified into three groups according to blood Mn (<1, 1–1.5, >1.5 µg/100 mL) or duration of exposure (<3, 3–9, and >9 years) and the control workers were included as a separate group. The prevalence of abnormal values for simple reaction time and short-term memory were not related to blood Mn. The prevalence of abnormal values for eye-hand coordination, hand steadiness (hand tremor) increased with increasing blood Mn concentrations (chi-square test; p < 0.02). Results of the eye-hand coordination test in the exposed workers suggest the existence of a Mn-B threshold level at about 10 µg Mn/L; no Mn-B threshold was evident for hand steadiness. No statistically significant relationships were found between neuropsychological functioning and duration of Mn exposure. No statistically significant relationship was found between the prevalence of CNS symptoms and abnormal CNS test results and the subjective estimation of integrated exposure to Mn by the chief foreman. The authors suggested that workers exposed to airborne Mn (total dust) of about 1 mg/m3 for less than 20 years may develop preclinical signs of intoxication and that psychomotor tests are more sensitive for the early detection of adverse effects of Mn on the CNS than the standardized neurological examination. The article does not describe how air sampling was performed.
Summary
Overall, reported data on Mn-B as a biomarker of exposure or as a risk factor of neurofunctional impairments are limited and inconsistent.
3.2.1.4. Mn-B in workers of Fe-Mn and Si-Mn production facilities
At Fe- and Si-Mn alloy production factories, workers could be exposed to insoluble Mn oxides dust. After mineral ores are crushes and sintered, fusion takes place in electric furnaces. The input mixture into electric furnaces includes about 80% pyrolusite ore with a Mn content of about 70%. Almost all the Mn (95%) is in the form of insoluble Mn oxides (MnO2, MnO3, MnO4) dust. The respirable fraction of Mn-A particles is about 50–60% (Lucchini et al. Citation1997). The respirable fraction of Mn particles is about 50–60% (Lucchini et al. Citation1997). Several studies were conducted in Italy on workers employed at Fe-Mn and Si-Mn alloy production plants (Fe-Mn/Si-Mn). More details regarding these studies could be found in Supplemental Material 5 (Table S5.1).
Lucchini et al. (Citation1995) compared 58 workers during a period of forced cessation of work (1–42 days) at Fe-Mn alloy factory exposed to Mn (95% in the form of oxides and 50–60% was in respirable fraction of total Mn-A) to 19 control subjects. The Mn-CEI was calculated for each subject, multiplying the average annual Mn-A concentration (respirable dust characteristic) of each job by the number of years in which this activity was perform. Workers were exposed to Mn (in the form of Mn-CEI (total dust) from 0.199 ± 0.260 (median) (range 7.9–9.5 μg/m3) to 0.668 ± 0.590 (median) (range 0.015–2.130) µg/m3 and control subjects were exposed to 0.177 ± 0.213 (median) (range: 0.001–0.943) µg/m3. Blood Mn in Mn exposed workers ranged from 7.9 to 18 µg/L and from 4 to 7.4 µg/L in the non-exposed subjects. After the transformation of the Mn-B level and the Mn-CEI in the logarithmic form, a good correlation was observed at an individual basis between log Mn-B and log Mn-CEI (r2 = 0.36, p = 0.0001) and between Mn-B and the following neurobehavioral tests: finger tapping (rs = 0.15, p = 0.004), symbol digit (rs = 0.22, p = 0.004), digit span (rs = 0.12, p = 0.01), and additions (rs = 0.26, p = 0.0001). The correlation coefficients increased in subjects tested more than 13 days after cessation of exposure. Personal and stationary air sampling were conducted before blood sampling, however, duration of air sampling and details on measurement techniques were not reported. Blood samples were collected after cessation of exposure but immediately before the neurobehavioral testing. Reported findings are in contrast with data reported by Roels et al. (Citation1992) who did not find a correlation between Mn-B and Mn-A exposure at the individual levels which might be related to the timing of the current study where the Mn-B as a potential biological indicator of exposure was assessed after a period without of exposure and at a different occupational (dry alkaline battery) plant. Lucchini et al. (Citation1995) study was conducted during a cessation of work at the plant which eliminated the influence of night shifts on behavioral functions and recent exposure; reported findings can reflect only body burden during past exposure.
Two years later, Lucchini et al. (Citation1997) conducted study on the currently exposed 35 furnace and casting workers from an Italian Fe-Mn/Si-Mn alloy factory; control subjects were 37 electric company workers with no known chemical exposures. Most of the Mn-A was in the form of oxides with Mn concentrations averaging 93 µg/m3 (total dust). Air Mn sampling was determined by personal and stationary sampling and performed approximately 1 month before the testing period. The Mn-CEI was calculated by multiplying the average annual airborne Mn concentration characteristic of each job performed by the subject during his work history by the corresponding estimated average pulmonary ventilation and by a number of exposure years. Blood samples were collected in the morning before the work shift and immediately before the neurofunctional testing procedure. Neurofunctional examination was conducted after two workdays off before work shift. The average (GM) Mn-B in exposed and referent workers was 9.84 (range 4.6–23.4) µg/L and 6.78 (range 4.8–10.9) µg/L, respectively. A strong correlation was found between log Mn-CEI and log Mn-B (r = 0.52, r2 = 0.27, p = 0.002); the correlation was higher when the Mn-A respirable fraction was used instead of inhalable dust. Higher Mn-B concentrations were correlated with poorer Aiming Pursuit II test results (n = 30, r = 0.37; r2 = 0.13, p = 0.04), no statistically significant differences were found for motor function performance between exposed and control subjects. No relationship was observed between olfactory functions and Mn-B in this study. Exposure levels were significantly reduced at the plant, and it could be suggested that the degree and a scope of neurofunctional impairment was induced by the cumulative past exposure to the higher Mn levels.
Lucchini et al. (Citation1999) compared 61 workers from an Italian Fe-Mn/Si-Mn alloy production plant to an age-matched group of 87 workers from a civil hospital with no known exposure to neurotoxins. Most of the Mn-A was in the form of oxides (total dust) and around 40–60% was in respirable fraction. Blood samples were collected in the morning before the work shift and immediately before testing. Mn concentrations were measured using personal and stationary sampling (duration of sampling not reported). The overall Mn-A concentrations measured in various workplace areas in total and respirable dust ranged from 5–1490 µg/m3 (GM 54.25) and 1–670 µg/m3 (GM 17.18), respectively. Mn-CEI was calculated for each subject by multiplying the average annual Mn-A concentration (total dust) by the number of years of work. The average (GM) Mn-B in exposed and control subjects was 9.18 µg/L (4–19) and 5.74 µg/L (2–9.5), respectively. A significant positive correlation between Mn-A (total dust) and Mn-B was observed (r = 0.36, r2 = 0.13, p = 0.0068), however, no association was found between Mn-CEI and Mn-B. The exposure subgroups were divided based on log CEI values by low (<0.50 mg/m3 × years), medium (0.50–1.80 mg/m3 × years), and high log CEI (>1.80 mg/m3 × years) and revealed significant dose-response relationships in the finger tapping (dominant hand and non-dominant hand) (both, r = 0.32, r2=0.10, p = 0.01), Digit Span (r = 0.44, r2 = 0.19, p = 0.004), and symbol digit tests (r = 0.33, r2=0.11, p = 0.01). No association was found between tests results and Mn-B. No difference for contrast sensitivity was observed between Mn-exposed and control subjects. Lucchini et al. (Citation1999) suggested that Luria motor subtests could be used to discriminate between Mn-exposed and control subjects. The authors proposed the average annual exposure level lower than 100 µg/m3 as a protective level for workers for the entire working life. Despite that the study by Lucchini et al. (Citation1999) remains one of the most reliable studies, it is unclear if the same relationship will be observed at the current levels of exposure and selected neurofunctional tests at Fe-Mn and Si-Mn alloy production plants.
Apostoli et al. (Citation2000) assessed the relationship between the airborne Mn levels and blood Mn-B in 94 workers in the Italian Fe-Mn/Si-Mn alloy production plant exposed to Mn oxides (MnO2 and Mn3O4) and in 87 non-exposed subjects. Exposure levels of airborne dust and fume were detected using personal sampling during half the work shift and ranged between 5 and 740 μg/m3. Blood samples were collected at the end of the shift. On a group basis, a correlation was observed between Mn-A (total dust) and Mn-B (r = 0.34, r2 = 0.112, p = 0.001). No association was observed between Mn CEI and Mn-B. Apostoli et al. (Citation2000) concluded that Mn-B can differentiate groups of Mn exposed workers from groups of non-exposed subjects or can be used as an indicator of the intensity of external exposure, but it is not suitable at individual basis due to high individual variability.
Smargiassi et al. (Citation1995) compared 11 workers at a Fe-Mn and Fe-Cr alloy plant and 15 controls (blood donors) in a study focused on markers of catecholamine metabolism. Levels of Mn-B of exposed workers (geometric mean 11.6 (range, 6.7–23.4 µg/dL) were significantly higher than those in control subjects (geometric mean 6.2 (range, 4.8–8.4 µg/dL). Within the exposed group, Mn-B was positively related to both respirable and total Mn-CEI (both, r2 > 0.59, p < 0.01). The authors acknowledge that the results require confirmation due to limited sample size “with careful analysis of potential confounders”.
Smith et al. (Citation2007) compared 123 workers of a Fe-Mn and Fe-Cr alloy plant and 24 blood donors (as a control group). Blood was collected at the end of the work shift in parallel with air samples. In Fe-Mn alloy workers, Mn-B was significantly associated with Mn-A (total dust) concentrations in subjects currently exposed to low (median = 0.42 µg/m3) and moderate (median = 4.2 µg/m3) Mn-A concentrations, but not in workers exposed to the highest Mn-A concentrations (median = 292 µg/m3). Smith et al. (Citation2007) suggest that a complex and limited relationship exists between exposure and blood Mn levels that may depend upon the magnitude, duration, frequency, variability, and latency of exposure pattern and the timing of blood sampling relative to exposure and a careful consideration of use blood Mn for constructing exposure metrics and determination of exposure-effect relationship is needed.
Several studies were conducted in Canada. Mergler et al. (Citation1994) compared 115 male workers in a Fe-Mn/Si-Mn alloy plant with matching working subjects (controls) without a history of exposure to neurotoxicants in Southwest Quebec, Canada. Mn-A was determined using stationary sampling [means: 0.89 mg Mn/m3 (total dust) and 0.04 mg Mn/m3 (respirable dust)]. Blood samples were taken prior to exposure on the last day of the worker’s shift. The mean Mn-B was higher in Mn-exposed workers (geometric mean: 10.3 µg/L versus 6.8 µg/L in controls), but these values did not exceed the upper value of normal limit at 12 µg Mn/L of blood (US EPA Citation1984). Mn-B levels were not associated with cumulative Mn-CEI (total dust). The authors found impaired motor functions and lower olfactory perception threshold in Mn-exposed group compared to controls. In general, Mn-A levels of exposure were below the recommended levels, Mn-B did not exceed the normal level, and none of the participants reached a clinical level of neurological disorders (e.g. Luria Nebraska Neuropsychological Test Battery). Potential relationships between Mn-B and neurological impairments were not studied. Reported findings indicate that in the presence of normal Mn-B values in the Mn-exposed workers, with apparent sub-clinical deficits, Mn-B could not be considered as a suitable biomarker of exposure.
Bouchard et al. (Citation2003) examined a possible relationship between Mn-B and mood states in Mn-exposed workers following alcohol consumption and using a data set reported by (Mergler et al. Citation1994). Mn-A (respirable fraction) exposure in the plant averaged 0.23 mg/m3 and was correlated with Mn-B but not Mn CEI (respirable fraction). Workers were grouped according to their Mn-B concentration (<10 and ≥10 µg/L) and alcohol consumption (<400 and ≥400 g per week). There was no difference in the Profile of Mood States (POMS) scale scores between Mn-B subgroups.
Beuter et al. (Citation2004) compared ten workers 12 months after cessation of exposure at Fe-Mn and Si-Mn alloy plant in Quebec, Canada to 11 control subjects (a sub-sample from Mergler et al. (Citation1994). Differences between these groups were examined based on measuring motor performance (postural tremor with visual feedback). Mn-B was measured at the end of exposure for the Mn group only and 12 months later for both groups. At the time of plant closure, the mean (SD) value for Mn-B levels of the workers was 1.06 (0.42) μg/100 ml and showed significant correlation (Spearman’s rank coefficient) with both harmonic index (rs = 0.70, p = 0.03) and first maximum of the autocorrelation function (rs = 0.89, p = 0.001). However, Mn-B levels were similar in both groups 12 months after cessation of exposure and had only insignificant correlation with tremor variables. Reported findings suggested that neurofunctional alterations still be detected at the normal Mn-B levels in the Mn-exposed workers. The small number of subjects in the groups, lack of reported Mn-A levels, and a complex calculation of variables in the analysis limited the generalization of the results.
Bouchard, Mergler, Baldwin, Panisset, Roels (Citation2007) examined neuropsychiatric symptoms among 77 Mn-exposed workers from a Fe-Mn and Si-Mn plant, 14 years after the plant was closed and 81 matched-pair referents in South-West Quebec, Canada. Mn-A exposure at the plant was measured in 1991. The Mn-A sampling (total dust, respirable fraction, and Mn content) was conducted by personal monitoring. The 8 h time-weighted average environmental measures of Mn-A (total dust) levels ranged from 0.01 to 11.48 mg/m3 (GM: 0.23 mg/m3) while the Mn-A (respirable fraction) ranged from 12–35% of total Mn (reported in Baldwin et al. (Citation1993)). The exposure history of each worker was re-constructed, and Mn cumulative exposure indices (Mn-CEI) were calculated for each of employment. T-scores on scales of Depression and Anxiety, used for evaluation of neuropsychiatric symptoms, were significantly higher among former Mn-workers than referents. Study groups were stratified based on Mn-CEI levels [mean ± SD, median] [highest: 58.2 ± 21.7, 55.0 mg/m3; middle: 19.5 ± 5.0, 19.0 mg/m3; lowest: 6.0 ± 4.3, 6.2 mg/m3]. Former workers in the two highest tertiles of Mn-CEI showed a higher risk for hostility (OR 7.5; 95% CI, 1.5–38.9), depression (OR 2.6; 95% CI, 1.1–8.4) and anxiety (OR 3.0; 95% CI, 1.1–8.4). Bouchard, Mergler, Baldwin, Panisset, Bowler, et al. (Citation2007) also examined neurobehavior functions in the same set of workers. The mean total score on the Luria Motor Scale was significantly higher in Mn-workers than in referents (p < 0.01). Higher levels of exposure were significantly associated with poorer scores on the Luria Motor Scale (p < 0.01). Significant relationships were observed for scores on the Hand Steadiness Test (time of contact), and color-word trial of the Stroop Color-Word Test (p < 0.05). Increased level of cumulated exposure was positively associated with scores on the Confusion-Bewilderment POMS scale (p < 0.01). There was also a trend for higher scores on anger-hostility (p = 0.06) and fatigue-inertia (p = 0.07) scales. However, in the lowest Mn-CEI tertile, the Luria Motor Scale performance scores in the former workers were significantly lower from the controls (p < 0.05), which indicate an improvement in performance. The possible bias of healthy workers effect and uncertainty in occurrence of mortality among both groups can influence results for both initial and follow-up studies. Re-constructed exposure can also induce some exposure miscalculations for both initial and follow-up studies. Re-constructed exposure can also induce some exposure miscalculations for both initial and follow-up studies.
Park, Bouchard, et al. (Citation2014) examines in detail the exposure-response relationships between airborne Mn and neurobehavioral outcomes in Mn-exposed workers and referents using data from the earlier study of Mergler et al. (Citation1994) and the follow-up study of Bouchard, Mergler, Baldwin, Panisset, Bowler, et al. (Citation2007). Origin of exposure and type of exposure particles (e.g. large respirable particulate (Mn-LRP, dust), as well as small respirable particulate (Mn-SRP, fume), were used together with detailed work histories to construct exposure metrics. Eight neurobehavioral outcomes were modeled in relation to past Mn exposure. The authors concluded that observed deficit in neurobehavioral performance was mainly dependent on furnace-area emissions rather than mechanical origin of dust particulates and associated with duration of exposure for Mn-A exposures less than 0.2 mg/m3 (respirable fraction). Park, Bouchard, et al. (Citation2014) mentioned that “the challenge for risk assessment is to identify the upper bound of internal Mn tissue levels under normal metabolic regulation and to establish an exposure response in this range on external exposures”. In a consequent study, Park, Baldwin, et al. (Citation2014) modeled Mn-B levels in relation to prior Mn exposure using data from the earlier study of Mergler et al. (Citation1994) and the follow-up study of Bouchard, Mergler, Baldwin, Panisset, Bowler, et al. (Citation2007). Despite that exposure levels estimated for individual jobs varied, duration of employment (exposure) was itself a strong predictor of Mn-B levels and neurobehavior effects. As it mentioned above, re-constructed exposure may induce some exposure miscalculations for both initial and follow-up studies.
Two studies were conducted in Norway (Ellingsen, Hetland, et al. Citation2003; Bast-Pettersen et al. Citation2004). Bast-Pettersen et al. (Citation2004) conducted a cross-sectional study to investigate neurobehavioral outcomes in 100 Fe-Mn and Si-Mn alloys producing plants workers and 100 age-matched controls (workers) from silicon metal and microsilica plant and from a titanium oxide slag and pig iron plant. Personal air samples were collected on three days for each subject closely before the neurobehavioral assessment and blood samples. Arithmetic means for Mn-A (inhalable dust) and Mn-B in the Mn-exposed workers were 0.75 mg/m3 (range 0.01–0.0115 mg/m3), and 189 nmol (10.44 μg/L) in blood (range 84–426 nmol/L, equal to 4.64–23.53 μg/L (using equation 18.1 nmol Mn/L = 1 µg Mn/L), respectively. Mean levels of Mn-B in control workers (166 nmol Mn/L, equal to 9.17 μg/L) were significantly lower than levels in exposed workers. Postural tremor, as measured in the hand steadiness test, was significantly increased in the Mn-exposed group compared to the controls and showed an exposure-response relationship with different durations of employment. However, results from an alternative test of tremor (“Tremor”) did not distinguish between the Mn-exposed group and the control group. The authors reported that a previous Mn exposure in the plants was not available for review, but some evidence suggest that previous levels of exposure might be 2–3 times higher than current levels.
In the cross-sectional study, conducted in Northern Taiwan, Hua and Huang (Citation1991) assessed the effects of chronic exposure to Mn on the neurobehavioral function of the exposed workers at a Fe-Mn alloy plant. The study included 17 exposed workers (Group 1) and 19 control subjects (Group 4). Levels of Mn-B in the exposed workers (range 0.60–137.70 ppb) were considerably higher compared to normal values (range 7.0–12.0 ppb values). There was no evidence of neurobehavioral deficits in workers with chronic exposure to Mn based on the tests of orientation, intelligence, learning and memory, language and communication, visuospatial and visual perception, visual attention, manual dexterity, and information processing speed in comparison with controls, with the exception of a mild slowdown in response speed which can be also attributed to the small sample size of both groups. The authors concluded that chronic Mn exposure did not affect workers’ neurobehavioral function compared to the established control group.
In the study conducted in Iran, Hassani et al. (Citation2016) assessed the relationship between Mn-B and neuropsychological effects in 31 Fe-Mn smelters (Mn-exposed groups) and 30 office workers (as unexposed controls). The mean concentrations of air Mn for ferroalloy smelter groups were 0.008 ± 0.005 mg/m3. Mn concentrations in blood samples of exposed workers ranged between 1.80 and 32.60 μg/L and were significantly higher than Mn concentrations in the controls. Statistically significant weak correlation was found between blood Mn in the workers and air Mn. Significant inverse relationships between neurocognitive outcome measures and Mn-B levels were also found. Hassani et al. (Citation2016) suggest that blood Mn could be used to distinguish Mn-exposed workers from unexposed population at the group level. However, similar to many other studies, the major limitation of this cross-sectional study was small sample size.
In a cross-sectional study in South Africa, Myers, Thompson, Naik, et al. (Citation2003) evaluated neurobehavioral end points in a group of 509 workers at a Mn smelter and compared results with a group of unexposed workers from an electrical fittings assembly plant (remote from the Mn smelter). Mn exposure levels were assessed using personal air samples and blood samples. Mn-CEI indices were calculated for each exposed worker based on Mn concentrations in “inhalable” dust fraction from personal air samples and job histories. No association was found between Mn-B with neurobehavioral test results. Associations were observed between Mn-B and Mn-A expressed as Mn-CEI or exposure intensity. A receiver operating characteristic (ROC) analysis showed that a Mn-B cut-off of 10 µg/L (the 95th percentile in the unexposed) could be used as a screening tool to discriminate between individual exposures at the ACGIH threshold limit value (TLV) of 0.2 mg/m3. Young et al. (Citation2005) re-analysed results reported by Myers, Thompson, Naik, et al. (Citation2003) by estimation of exposures to Mn in “respirable” dust as opposed to “inhalable” dusts in the earlier analyses. Results were similar to the those reported by Myers, Thompson, Naik, et al. (Citation2003). The authors concluded that exposure estimates based on respirable dust do not provide a more sensitive method to detect Mn neurobehavioral effects.
Blond and Netterstrom (Citation2007) conducted a longitudinal study to evaluate neuromotor function in a Danish cohort of active (n = 60) and former steel workers (n = 32) exposed to Mn-containing vapours and Mn metal dust (from 1989 up to 2002). After 2002, the primary exposure was from dust and red-hot steel. A control group (workers, employed as office workers, smiths, and electricians) was examined in 1996 (n = 19) and in 2003 (n = 14). The steel workers showed a decline in the ability to perform fast precise hand pronation/supination and finger tapping from 1995 to 2005 compared to control group. Correlation analysis did not find associations between test results for fast hand coordination and blood Mn as independent variables; a trend was found between Mn-B in 1995 and fast right-hand pronation/supination (r2 = 0.26, p = 0.06). Blond and Netterstrom (Citation2007) hypothesized that the observed findings might be also the result of combined exposures to Mn and Pb, as well as to other occupational hazardous compounds. According to Blond and Netterstrom (Citation2007), this study limitations include: the occurrence of significant results by chance could not be excluded, examiner bias can be possible, the observed higher level of Mn-B among the former steel workers led to their unwillingness to participate in the study which could represent a possible selection bias, age and lifestyle factors, such as alcohol and tobacco use were potential confounders. The control group was non-matched in term of alcohol consumption. Overall, diverse characteristics of the studied participants and exposure patterns in this study limited reliability of reported findings.
In China, Jiang et al. (Citation2007) conducted a cross-sectional study to investigate a possible correlation between Mn-B and neurotoxic outcomes in Mn smelters. Thirteen smelter workers had high Mn-A exposure (mean 1.26 mg/m3), and five subjects were selected from power distribution department from a ferro-alloy factory with low level of Mn-A exposure (mean 0.66 mg/m3, a range 0.23–0.77 mg/m3). Nine office or cafeteria workers (all males) were selected as a control group from a non-smelting factory (Mn exposure, mean 0.01 mg/m3, range 0–0.03 mg/m3). None of workers had clinical symptoms and/or signs of manganism.
Rolle-McFarland et al. (Citation2018) conducted a cross-sectional study of 60 Chinese workers to determine a possible association between Mn-A exposure and Mn-B. Thirty workers were recruited from an equipment manufacturing and installation factory with limited use of Mn, and 30 participants worked at Mn Fe-Mn factory. Self-described occupational history reported by workers was used to compute two Mn-CEI for each participant, one for the previous 16 years of exposure (Mn-CEI-16) and the second one for total work history (Mn-CEI (total)). Mean Mn-CEI (total) and Mn-CEI-16 were 37.5 and 25.0 mg/m3, respectively. Median Mn-B was 14.1 (4.0) μg/L, respectively. Mn-CEI-16 and Mn-B were significantly higher in the exposed workers compared to the reference group (p = 0.02 and p < 0.01, respectively). The study limitations include: lack of air sampling data, Mn-CEI was constructed based on self-reported occupational history and exposure misclassification could not be ruled out; alcohol consumption and PPE use by workers was not reported; time of blood sampling in relation to air sampling was not available; clinical significance of reported findings not discussed; rationale/methods for selection of participants not reported, Mn-CEI for lifetime work history was higher in the selected control group compared to the Fe-Mn alloy workers; potential confounding was not appropriately adjusted for the final analysis. Based on the reported limitations, a generalizability of the reported findings is limited. In a follow up study, Rolle-McFarland et al. (Citation2019) investigated a potential cross-sectional association between Mn-B with cognitive and olfactory functions among Mn-exposed workers. BMn was not associated with cognitive function in Mn-exposed workers or with olfactory function. Limitations of this study include small sample size and a possibility of cross-contamination of biological samples; in addition, this study does not include a Mn non-exposed control group.
In the study on refinery workers, Brown et al. (Citation1991) reported differences between 19 exposed workers exposed to high levels of Mn dust (mean 10.58 mg/m3) (>TLV at 5 mg/m3) and 20 control subjects with significantly lower exposure (mean 0.81 mg/m3). No association was found between blood Mn levels and neuropsychological outcomes. Despite that “soft” neurological signs were observed in 31.4% of the cases (particular group is not provided), they were all within normal limits. Significant correlations were found between level of exposure to Mn-A dust and the visuoconstructional dyspraxia (neuropsychological domain associated with an ability to complete the construction tasks, such as drawing or assembling the various parts of an object into a complete structure). Lack of reported details on timing and methods of Mn detection in blood limited the reliability of reported findings.
Summary
Among reviewed studies on Fe-Mn- and Si-Mn alloy production plants workers, 2 studies were conducted in Norway (Ellingsen, Haug, et al. Citation2003; Bast-Pettersen et al. Citation2004), 3 studies in Canada (Mergler et al. Citation1994; Bouchard et al. Citation2003; Beuter et al. Citation2004), five studies in Italy (Lucchini et al. Citation1995; Smargiassi et al. Citation1995; Lucchini et al. Citation1999; Apostoli et al. Citation2000; Smith et al. Citation2007), 3 studies in China (Jiang et al. Citation2007; Rolle-McFarland et al. Citation2018, Citation2019), 3 studies in South Africa (Brown et al. Citation1991; Myers, Thompson, Naik, et al. Citation2003; Young et al. Citation2005), one study – each in Denmark, Iran and Northern Taiwan (Hua and Huang Citation1991; Blond and Netterstrom Citation2007; Hassani et al. Citation2016), respectively). Ten studies reported positive associations between blood Mn and different types of measured air Mn (Mergler et al. Citation1994; Lucchini et al. Citation1995; Smargiassi et al. Citation1995; Lucchini et al. Citation1999; Apostoli et al. Citation2000; Bouchard et al. Citation2003; Myers, Thompson, Naik, et al. Citation2003; Young et al. Citation2005; Smith et al. Citation2007; Hassani et al. Citation2016). Among 11 researchers who studied an association between Mn-B and CNS functions impairment, 7 reported an association between Mn-B and neurological outcomes (Mergler et al. Citation1994; Lucchini et al. Citation1995; Bouchard et al. Citation2003; Ellingsen, Hetland, et al. Citation2003; Bast-Pettersen et al. Citation2004; Beuter et al. Citation2004; Blond et al. Citation2007). However, the same researchers did not find an association between blood Mn and other neurological outcomes (Ellingsen, Hetland, et al. Citation2003; Bast-Pettersen et al. Citation2004). No association between Mn-B and studied neurological outcomes was reported in several other studies (Brown et al. Citation1991; Hua and Huang Citation1991; Lucchini et al. Citation1999; Jiang et al. Citation2007; Hassani et al. Citation2016).
The reviewed studies suggest that, in occupational settings, Mn-B does not always reflect airborne Mn exposure. Lucchini et al. (Citation1995) observed associations between Mn-B and Mn-CEI across all jobs in workers during a laid-off period. It was postulated that the correlation between Mn-CEI and Mn-B appeared as Mn-B levels were related to the Mn released from the body deposits and were not influenced by current exposure (Mergler and Baldwin Citation1997). In their later work on currently exposed workers, Lucchini et al. (Citation1999) observed associations between Mn-B and Mn-A (total dust) but not with cumulative exposure. Baker et al. (Citation2014) reported a positive association between Mn-A and Mn-B and hypothesize that below the exposure to the Mn-A concentration at about 10 μg/m3, Mn in the body is dominated by dietary Mn and any additional inhaled Mn causes only negligible changes in Mn levels due to the homeostatic regulations (unless the workers are exposed to high dose). Excess of Mn in blood above 10 µg/L may be useful in clinical practice for identification of Mn-exposed persons, who is in exceedance of safety regulatory values such as the current ACGIH TLV of 0.2 mg/m3. However, the practical usefulness of the suggested threshold diminished due to high interindividual variability. Several researchers concluded that there is no good relationship between Mn in blood and external exposure metrics (Mn-CEI) (Lucchini et al. Citation1999; Apostoli et al. Citation2000; Bouchard et al. Citation2003; Myers, Thompson, Ramushu, et al. Citation2003) or Mn-A (total dust), respirable or inhalable fractions (Ellingsen, Hetland, et al. Citation2003). Several reviewed studies demonstrated that there was no apparent association of neurofunctional impairments with internal Mn-B exposure (Lucchini et al. Citation1999; Mergler et al. Citation1994; Bouchard et al. Citation2003; Blond and Netterstrom Citation2007). It is not surprising, as with decreasing Mn exposure levels, clinical effects become more subtle and if there had been convincing adverse effects it would be possible to future explore the utility of Mn-B as a screening tool and a risk factor. Reviewed studies indicate that, on an individual basis, levels of Mn-B are not reliable predictors of Mn-A exposure and neurofunctional impairment.
3.2.1.5. Mn in blood of welders
Welding is a well-recognized source of Mn exposure. Exposure to Mn varies considerably depending on the welding process and the amount of Mn in the welding wire, rods, flux and base metal (Smargiassi et al. Citation1995). Physicochemical properties, such as particulates size, composition, and solubility of welding fumes are critical for the bioaccessibility of Mn and the Mn neurotoxic effects. In contrast to exposure to Mn-A dust particulate, welders are exposed to more than 90% of airborne particles in the respirable fraction in the welding fumes which lead to a deep penetration of Mn in the lungs and increase of Mn bioavailability and access to the target tissue. In confined space welding, the exposure to Mn-containing fumes may exceed 1 mg Mn/m3 (Ellingsen et al. Citation2006). Refer to Supplemental Material 8 for a summary of types of welding and corresponding metals involved.
Numerous studies have examined the suitability of Mn-B as a potential biomarker of occupational Mn exposure and a risk factor of neurofunctional impairments (Jarvisalo et al. Citation1992; Sjogren et al. Citation1996; Barrington et al. Citation1998; Hałatek et al. Citation2005; Kim et al. Citation2005; Ellingsen et al. Citation2006; Yuan et al. Citation2006; Bowler, Nakagawa, et al. Citation2007; Bowler, Roels, et al. Citation2007; Ellingsen et al. Citation2007; Kim et al. Citation2007; Smith et al. Citation2007; Ellingsen et al. Citation2008; Cavallari Citation2010; Laohaudomchok et al. Citation2011; Pesch et al. Citation2012; Ellingsen et al. Citation2013; Ellingsen, Chashchin, et al. Citation2014; Ellingsen, Kusraeva, et al. Citation2014; Baker et al. Citation2016; Bonberg et al. Citation2017; Casjens et al. Citation2017; Casjens et al. Citation2018; Ellingsen et al. Citation2019; Mehrifar et al. Citation2020; Stanislawska et al. Citation2020). Six of 26 reviewed articles reported that Mn-B was significantly higher among exposed workers compared to controls. Five studies reported a lack of statistically significant difference between exposed workers and controls. Nine studies reported a positive association between Mn-B and Mn-A, and four studies found no association between Mn-B levels and Mn-A exposures. Four studies reported an association between Mn-B and neurological outcomes. Characteristics and findings from these studies are provided in Supplemental Material 6 (Table S6.1).
Several studies were conducted in the United States. Using findings from short-term and cumulative 30- and 90-day exposures to Mn in welder trainees, Baker et al. (Citation2016) found that following 30 days of exposure, a 1 mg/m3 – days increase in Mn-A exposure was correlated with a 0.57 ng/mL increase in Mn-B (95% CI: −0.04, 1.19) and after a 90-day (95% CI: −0.04, 1.19) and a cumulative exposure window, a 1 mg/m3-days increase in Mn-A was correlated with a 0.26 ng/mL increase in Mn-B (95% CI: 0.005, 0.51) and 0.09 ng/mL (95% CI: 0.006, 0.17, respectively). The authors suggested that Mn-B may serve as a potentially useful biomarker of Mn exposure in welders only over longer time periods or high levels of exposure when the homeostatic control of Mn in the blood is perturbated.
Barrington et al. (Citation1998) studied the cognitive ability and emotional behavior in eight subjects with occupational Mn exposure at Mn alloy welding (railway track frogs) and machinists. No significant relationships between Mn in blood and length of exposure or neurobehavioral tests were found. This study did not have a control group and the sample size of the exposed subjects was small. Bowler, Roels, et al. (Citation2007) conducted a study to identify the dose–effect relationship between adverse health effects and Mn-A or whole blood in welders working in a confined space during construction. Forty-three welders performed welding on the bridge for 16.5 months (mean) with little or no personal protection equipment. The mean time weighted average of Mn-air ranged from 0.11–0.46 mg/m3. Blood Mn levels > 0.10 mg/L were found in 43% of the workers. Significant inverse dose–effect relationships with Mn-CEI and/or Mn-B were found for IQ (p ≤ 0.05), executive function (p ≤ 0.03), sustaining concentration and sequencing (p ≤ 0.04), verbal learning (p ≤ 0.01), working (p ≤ 0.04), and immediate memory (p ≤ 0.02) after adjusting for demographics and years of welding. However, because this evidence derived from litigation study, this study provides detailed information on likely Mn effects in a welder population but does not permit quantitative estimates of excess risk.
Cavallari (Citation2010; results published in Laohaudomchok et al. (Citation2011)) examined 28 welders in the welding school from a boilermaker union. Median airborne Mn concentrations measured over a work shift ranged from 2.02 to 137.41 µg/m3 (median 12.89 mg/m3). Mn-CEIs were calculated for the following exposure windows: 1–6 months, 7–9 months, 10–12 months, 7–12 months, and 1–12 months. Blood Mn did not correlate with Mn-CEI for any time window. There was no significant correlation between air Mn over the work shift and post-shift blood Mn, and changes in blood Mn over the work shift. The sample size for blood sampling before and after shift was small (n = 27 and n = 24, respectively). Further, the range of exposure scenarios was more limited in the welding school than in many other occupational settings, which decrease the accuracy of Mn-CEI. In addition, potential errors in Mn-CEI based on retrospective work histories might reduce the correlations between bioindicators of Mn exposure and CEI.
Smith et al. (Citation2007) studied associations between Mn exposure in occupational settings and Mn levels in blood of bridge welders. Mn-B was significantly associated with calculated individual cumulative respiratory exposure index (Mn-CEI). The authors suggest that the relationship between Mn exposure and blood Mn levels may depend upon the exposure pattern and the latency of blood sampling relative to exposure.
Casjens et al. (Citation2017) studied active and occasional welders: 26 active welders with a median (interquartile range) Mn of 1121 (465; 1766) µg/m3 years (inhalable fraction), 215 occasional welders with a median Mn of 74.2 (27.2; 185) μg/m3 years (inhalable fraction), and additional 113 men worked in other occupations with anticipated Mn exposure (median Mn 25.1 (8.8; 78.8) μg/m3 years (inhalable fraction) to estimate effects of Mn on olfaction as a sign of potential neurotoxic effects. Mn-B concentrations at baseline (median; interquartile fraction) were 8.7 (7.2; 9.6) μg/L, 8.2 (6.9–10.3), and 8.3 (6.9–10.4), respectively. Mn-B concentration > 15 μg/L had only 2 active welders, 7 (18.9%) occasional welders and 1 worker (2.7%) from other occupations groups. There was no apparent association between Mn-B >15 mg/L and higher concentrations of inhalable Mn (>185 mg/m3 years) and olfactory perception.
Pesch et al. (Citation2012) investigated Mn in welding fumes and Mn in blood, potential predictors of exposure levels in German welders. The data came from a nationwide cross-sectional study that included 241 welders in German industries. The welders were equipped with two personal samplers, one to collect respirable particles and the other to collect inhalable particles in the breathing zone inside the helmets. The association between respirable Mn in welding fume and Mn-B was non-linear: there was no correlation between respirable Mn and Mn-B at respirable Mn concentrations below 50–100 µg/m3 (Spearman rs = 0.44, 95% CI: 0.33–0.54); above that threshold, Mn-B increased with increasing respirable Mn concentration. These findings indicate that homeostatic mechanisms regulate Mn in biological systems up to a certain exposure level.
Jarvisalo et al. (Citation1992) reported that the levels of Mn-B monitored throughout a full working week were significantly higher in manual metal arc (MMA) welders of mild steel (MS) in a shipyard in Finland than in an occupationally nonexposed population (reference group) and suggested that the measurement of Mn-B may be used for monitoring Mn exposure in MMA/MS welders only at the group level.
Several studies were conducted on Russian welders. Ellingsen and colleaguesFootnote1 (Ellingsen et al. Citation2008; Ellingsen et al. Citation2013; Ellingsen, Chashchin, et al. Citation2014; Ellingsen, Kusraeva, et al. Citation2014) measured Mn in whole blood of current welders, former welders diagnosed with welding-related manganism, and referents with no occupational exposure to Mn. In these studies, personal full-shift air sampling on two consecutive days preceding the biological sample collection was performed only in the current welders. No air sampling was performed for the referents, and no reliable data on air Mn were available for the former welders. When the 95th percentile of blood Mn concentrations among the referents was used as a cut-point, only 70% of the most highly exposed welders exceeded these levels with respect to blood Mn. Based on this finding, the authors concluded that, despite good correlations with exposure to soluble Mn in welding fumes, welders cannot be reliably distinguished from referents based on Mn in blood (Ellingsen et al. Citation2013). Levels of Mn in whole blood of the current welders were significantly higher compared to those in the referents in other studies (Ellingsen et al. Citation2008; Ellingsen et al. Citation2013; Ellingsen, Chashchin, et al. Citation2014; Ellingsen, Kusraeva, et al. Citation2014) and in the former welders diagnosed with manganism (Ellingsen et al. Citation2013; Ellingsen et al. Citation2019). In the former welders diagnosed with manganism, blood Mn concentrations were significantly higher compared to the referents (Ellingsen et al. Citation2013; Ellingsen, Chashchin, et al. Citation2014; Ellingsen et al. Citation2019). In the current welders, blood Mn was significantly positively correlated with Mn-Se (r = 0.69) and with Mn in urine (r = 0.64). No correlations among the biomarkers were seen in the former welders or in the referents (Ellingsen et al. Citation2013). In multiple regression analysis that included the current welders and referents, blood Mn was associated with being exposed to as a welder (Ellingsen, Chashchin, et al. Citation2014). In multiple regression analysis that included only the current welders, blood Mn was significantly positively associated with years of welding, as well as with biologically accessible Mn – the fraction of the total Mn soluble in artificial lung lining fluid (Hatch’s solution) – in air collected two days before the biological sample (Ellingsen et al. Citation2013; Ellingsen, Chashchin, et al. Citation2014). When the current welders were stratified into three equally sized groups according to air Mn, the welders had significantly higher blood Mn levels than the referents even in the lowest exposure strata (<6 µg/m3 of biologically accessible Mn in air collected two days before blood collection) (Ellingsen et al. Citation2013). The authors suggest that the long-term internal deposits may exist for insoluble Mn oxide particulates after cessation of exposure (e.g. the lungs) and Mn levels leaching from internal deposits may confound the results from current exposure. It suggests that Mn-B may be a less reliable biomarker of current exposure. Despite that correlations were observed between exposure to soluble fraction of Mn (Hatchsol Mn) in welding fumes and the concentration of Mn in blood, welders cannot reliably be discriminated from the referents based on Mn-B in blood due to the population background levels of Mn.
In a neurobehavioral study of current and former welders exposed to Mn, performance in the Digit Symbol Test of 32 current welders with highest blood Mn (mean 12.6 µg/L) was poorer (p < 0.01) compared to 32 matched referents (adjusted for education and age), whose mean blood Mn was 7.5 µg/L. The performance in this test was also significantly negatively associated with Mn-A. (Ellingsen et al. Citation2008).
Baker et al. (Citation2014) observed a positive association between Mn-A (mean) and Mn-B (mean) among studies reporting Mn-A concentrations above 10 μg/m3, based on the results of the segmented regression analysis of data extracted from 63 exposure groups in 24 published papers; however, using a log/log scale, a positive association between Mn-A and Mn-B was observed among studies reporting Mn-A concentrations above about 10 μg/m3. The authors suggested that interpretation of the results is limited by largely cross-sectional data, study design variability, and differences in exposure monitoring methods (Baker et al. Citation2014).
Two studies were conducted in Poland. Hałatek et al. (Citation2005) compared 59 shipyard welders and 23 controls (mechanics and electricians). The welders were exposed to significantly higher concentrations of Mn measured in personal air samples (mean (SD) 0.59 ± 0.85 mg/m3) compared to controls (mean (SD) 0.002 ± 0.002 mg/m3). Levels of Mn-B were higher (but not significantly) in welders (mean (SD) 11.42 ± 8.37 µg/dL) compared to controls (mean (SD) 6.07 ± 2.3 µg/dL). Mn-B levels significantly correlated with Mn-A and Mn-CEI levels (r2 = 0.72 and r2 = 0.66, respectively). Levels of Mn-B were higher in individuals who reported subjective neurological symptoms and in those with abnormal results of neurophysiological testes (EEG and VEP/visual evoked potentials) suggested by the authors for use in the detection of early effect of exposure to low Mn concentration. Reporting of this study is unclear and the confidence in the study results is low.
Stanislawska et al. (Citation2020) compared 67 chromium-nickel steel welders exposed to Mn-containing welding fumes to 52 control subjects without occupational exposure to metals. Metal inert gas (MIG) welding with a consumable welding electrode in the argon (Ar) shield was used. Mn in welding fumes were continuously collected in the workers' breathing zone during a shift. The workers did not wear powered air purifying respirator welding helmets. A strong correlation between the Mn-A concentrations (the inhalable and respirable fractions) and the Mn-B was observed (r = 0.58 and r = 0.46, respectively, both p < 0.0001, Spearman correlation coefficient), although Mn-B concentrations in welders was not significantly higher compared with the control group (median, 6.50 µg/L versus 5.70 µg/dL). Mn-B concentrations of the exposed welders were within the reference range for the general population 15 μg Mn/L (ATSDR Citation2012). Strong correlations were also observed between Cr (VI), Cr (III), and Ni in the air and urinary metal concentrations which contribute to the controversy regarding the role of inhaled Mn in the causation of neurofunctional impairments.
In South Korea, Kim et al. (Citation2005) analyzed the relationships between Mn-B and the integrated level of neurobehavioral performance in 111 males (welders, smelters and welding rod manufacturers) with occupational exposure to Mn using a structured regression model and cross-sectional data from a nationwide survey on workers exposed to manganese (Kim et al. Citation1999). A relationship between the Mn-A concentration and duration of exposure (as a surrogate of exposure) (correlation coefficient matrix = 0.334, p < 0.01) was found. Lack of information regarding past exposure to Mn-A which might impact the reported outcomes is a limitation of this study.
In the Iranian study, Mehrifar et al. (Citation2020) compared 38 welders exposed to welding (total) fume (at a mean level of 9.08 mg/m3) during shielded metal arc welding to 27 employees from the administrative department at the same metal construct manufacturer company. Sampling of total fume and analysis of Mn in fume were conducted in the breathing zone of welders; blood samples were obtained at the end of working shift. Average concentration of Mn in total fume was 0.81 mg/m3. Mn-B concentrations and frequency of neurobehavioral symptoms were significantly higher in welders compared to control group (18.33 ± 5.84 vs 10.24 ± 3.22 µg/L and 6.32 ± 2.83 and 1.55 ± 0.61, p < 0.01 and p < 0.02, respectively). A correlation was observed between Mn-A concentrations and Mn-B levels (rs = 0.352, Spearman correlation coefficient). A significant correlation was detected between the frequency of neurobehavioral symptoms (Q16 questionnaire) and Mn-A in the breathing zone (r = 0.504) and Mn-B levels (r = 0.643). A correlation was found between the Mn-A and Mn-B and neurocognitive indices (assessed by Stroop and Continuous Performance (CPT) tests) (r = 0.433–0.690, Pearson correlation coefficients). The authors concluded that that exposure to Mn-containing fumes induced neurobehavioral and neurocognitive impairments in welders. At the same time, the authors provided a slightly contradicting conclusion “because welders are exposed to numerous processes, ergonomic and environmental problems, gases, fumes, and multiple neurochemicals during welding (Finley and Santamaria Citation2005), the observed cognitive impairments cannot be solely attributed to Mn exposure.” Information was not obtained on the efficiency of the ventilation system at workplaces and the use of personal protective devices; however, it was mentioned that during air sampling “ventilation and personal respiratory protective equipment were also taken into consideration”.
Summary
The number of studies that reported blood Mn was higher among exposed workers compared to controls was similar to those that reported no differences between the Mn-exposed welders and non-exposed groups. However, a larger number of studies suggest that there is an association between Mn-B and Mn-A exposure compared to those that found no association.
It is not possible to directly compare findings from studies on welders to the studies on workers exposed mainly to Mn-containing dust particulates as the exposure to the different Mn forms and substances in different exposure scenario may have a considerable impact on uptake, absorption, and excretion of Mn in workers and the relationship between Mn-A and blood Mn. Based on the available data, it could be suggested that risk of neurofunctional impairments is relatively low at Mn-B below 10 µg/L. The question is whether workers exposed to Mn dust particulates uptake or absorb Mn in the brain to the same extent as welders.
3.2.2. Evidence of dose–response relationship between Mn-B and Mn-A and thresholds of health effects
Following the definition of a useful biomarker of exposure from Hoet and Roels (Citation2015), the biomarker should demonstrate a dose–response relationship with the occurrence of adverse health effect(s) or biomarkers of effect, providing information for health-based screening and research purposes. Results of several studies suggest that there is some evidence of the existence of a few dose-response relationships between Mn-B and Mn-A or neurofunctional impairments.
Inhalation exposure is a primary route and the first point of contact following inhalation exposure to Mn at occupational settings. “Manganese pneumonia” was a characteristic respiratory outcome in workers and animals exposed to high level of insoluble Mn dust (Suzuki et al. Citation1978; WHO Citation1981). WHO (Citation1981) experts concluded that “signs of adverse effects on the CNS may occur at Mn concentrations in air ranging from 2 to 5 mg/m3 (total dust).” A threshold at 1 mg/m3 (total dust) for less severe manganism was suggested by WHO in 1981. Findings from epidemiological studies in Mn-exposed workers indicate the presence of effects of Mn-containing particulates on the respiratory system at Mn-A levels even below 1 mg/m3, whereas studies of effects on the CNS below this level are equivocal or negative (US EPA Citation1984). It should be noted that these effects might be characteristic of insoluble particulate matter in general and not directly related to the Mn content of the particles.
In Mn oxides and Mn salts production plant, Roels, Lauwerys, Genet, et al. (Citation1987) suggest that a time-weighted average exposure to airborne Mn oxide (total dust) of about 1 mg/m3 for less than 20 years may lead to the occurrence of lung damage and CNS functions impairments (particularly, a disturbance in the eye-hand coordination and hand steadiness (peripheral tremor). The authors stated that the dose-response relationship between Mn-B and the eye-hand coordination test indicates the existence of a Mn-B threshold at about 10 µg Mn/L. However, the prevalence of reported respiratory symptoms and the lung function parameters did not differ between the referents and dry alkaline battery production workers exposed to Mn oxide (Roels et al. Citation1992).
In dry alkaline battery workers exposed to poorly soluble Mn oxide, Roels et al. (Citation1992) reported that the geometric means of Mn-B was significantly higher in the Mn-exposed workers than that in the referents (Mn-B 8.1 versus 6.8 µg/L). On an individual basis, no relationship was found between Mn-B and external exposure parameters (duration of exposure, current exposure, or lifetime integrated exposure to airborne Mn) or abnormal neurofunctional performance (simple reaction time, eye-hand coordination, or hand steadiness). On a group basis, no statistically significant association was found between Mn-B and current Mn-A concentrations. In this exposure-specific scenario, findings may be explained by a long-term co-exposure of workers to potassium hydroxide (“caustic potash”) powder, a typical base electrolyte used in alkaline batteries production and potential nephrotoxic effects. A 5% probability of increased hand tremor for a time-integrated Mn-A exposure threshold of 3.575 mg/m3 × year (total dust) and 0.730 mg/m3 × year (respirable dust) was reported in the same study, which indicates the importance of Mn particles size for interaction with biological systems and its target toxicity.
Dietz et al. (Citation2001) also proposed a threshold for Mn-B at 10 µg/L (based on the 95th percentile in the unexposed) which can be used to identify currently employed workers in dry cell battery production factory with exposure intensity in the current job exceeded the ACGIH TLV level of 0.2 mg/m3. Baldwin et al. (Citation1999) suggest that it may be a plateau level at 10 μg/m3 related to the homeostatic ability to control Mn-B at the individual levels of exposure which can be exceeded at the high levels of exposure. It could be suggested that lack of the observed effects on CNS functions, negative results on the association between Mn-B and neurological impairments in some studies could be explained by the fact that internal exposure did not meet a threshold for effect.
Because most (90%) of the airborne Mn particles in welding fume are in the respirable fraction, Mn bioavailability is likely to be higher than for coarse airborne Mn particulates as found in mining, milling of Mn ore, dry-battery assembly, and ferro/Si-Mn alloy production. Based on the review of published studies on airborne Mn dust and welding fumes and Mn-B, Baker et al. (Citation2014) suggested that exposure to concentrations below 10 μg/m3 Mn in welding fume would result in negligible changes in homeostatic regulations of Mn levels in the blood and the internal organs and tissues. Pesch et al. (Citation2012) did not observe a correlation between Mn-B and Mn in welding fumes up to 50–100 μg/m3, however, an increase of Mn-B with increased respirable Mn-A was detected which indicates that selection of different biomarkers might lead to different conclusions.
Hoet et al. (Citation2012) investigated the association between current levels of Mn in plasma (Mn-P) and airborne Mn in welders and suggested that an after shift Mn-P values could distinguish welders exposed to more than 20 μg/m3 in Mn-A from those who exposed to less than 20 μg/m3 Mn-A based on the Mn-P cut-off value of 2 μg/m3 (a sensitivity of 69% and a specificity of 82%). The authors suggested that Mn-P might be considered as a promising biomarker of current exposure to Mn-A in welders. However, a proposed threshold value could be considered with caution, as it remains in line with “normal” values on Mn in plasma in general population. In addition, due to the rapid clearance and very short half-life of Mn in plasma, Mn-P levels might serve only as an indicator of recent exposure and not suitable for the evaluation of cumulative long-term exposure.
Due to the different half-lives in the body, different biomarkers of Mn exposure represent different exposure time windows (current, recent, or cumulative [time integrated] exposure). The long-term occupational exposure of interest to Mn at occupational settings would lead to accumulation of Mn in the body and the blood Mn levels would reflect both current exposure and release of Mn into systemic circulation from internal Mn store depots (e.g. bone) due to past exposure. If blood is collected after exposure (e.g. retired workers, patients diagnosed with manganism), Mn in blood would primarily reflect body burden (assuming that dietary exposure to Mn would not significantly affect blood Mn levels). Following inhalation exposure to poorly soluble Mn oxide particulates, a certain amount of Mn particulate matter would be retained in the lungs, also providing a long-term depot of Mn that slowly release Mn ion into blood. Lucchini et al. (Citation1995) studied Fe-Mn alloy production workers 1–2 weeks after cessation of exposure and found a good logarithmic correlation between Mn-B and Mn-A CEI which reflects a cumulative exposure and Mn release from internal depots. Ellingsen et al. (Citation2006) also reported the higher Mn-B in former welders several years after cessation of exposure. Mn-B concentrations in these workers were significantly correlated only with Mn-A collected one day before blood sampling; however, the association disappeared when Mn-A levels were measured two days before blood sampling which indicates that Mn-B reflected a recent exposure. It should be noted that blood Mn thresholds should be considered with a caution due to the multiply factors that can influence homeostatic regulation of blood Mn (Bailey et al. Citation2018).
In the conducted meta-analysis, Meyer-Baron et al. (Citation2009) summarized epidemiological studies published between 1987 and 2008 and 13 studies examining 958 exposed and 815 unexposed workers were included in the meta-analysis. Mean concentrations of inhalable Mn ranged from 0.05 to 1.59 mg/m3, mean concentrations of Mn in whole blood ranged from 8.1 to 48.4 µg/L. Nineteen neuropsychological performance variables were analyzed. The analysis of exposure-effect relationships showed that larger effect sizes were more consistently associated with exposure to high concentrations of inhalable Mn-A than with Mn-B. The authors concluded that due to the inconsistencies in the relationship between Mn-B and effect sizes, Mn-B as a biomarker might not be sufficiently meaningful as a risk factor for the neurofunctional alterations. Information about individual Mn cumulative exposure would be useful for investigation of exposure-effect relationships and enhance the potential for human health risk assessment of Mn.
3.2.3. Mn in other biological matrices
Mn in blood components (erythrocytes (Mn-E), serum (Mn-Se), plasma (Mn-P)), as well as in other biological matrices including urine (Mn-U), bones (Mn-B), fingernails (Mn-Fn), toenails (Mn-Tn), hair (Mn-H) and saliva (Mn-Sa) have been suggested as potential markers of exposure and abnormal neurological outcomes. Studies that assessed the association of Mn in these biological fluids or tissues with measures of external exposure to Mn dust or with neurological outcomes at different occupational settings are briefly described below. More details regarding these studies are found in Supplemental Materials 2–6.
Reviewed studies provide inconsistent and/or limited evidence that Mn levels in biological fluids and tissues other than the blood adequately reflect Mn in occupational air or could be considered as a reliable biomarker for neurofunctional impairments.
3.2.3.1. Mn-Se in workers following Mn dust exposure at Mn mines and ore milling factories
At a Mn ore milling factory in Singapore, Chia et al. (Citation1993) found “markedly” higher mean Mn-Se levels in 17 workers exposed to Mn oxide (total dust) (mean level 1.59 mg Mn/m3) compared to 17 control subjects from a nearby hospital. A correlation was not reported between Mn-Se and Mn-A concentrations. Significant differences were observed between Mn-exposed and control groups in neurobehavior tests (the Santa Ana dexterity for the nondominant hand, finger tapping for the dominant hand, the Benton visual retention test, the digit symbol test, the pursuit aiming test (correct and sum), and the trail making test. Despite the observed differences at the group level, no significant correlation was observed between the tests scores and the Mn-Se concentrations in each worker. The small sample size is a limitation of this study and the selection of reference group from hospital workers might have introduced selection bias. For more details, see Supplemental Material 2, Table S2.3.
Summary
Reviewed studies provide inconsistent and limited evidence that Mn levels in serum reflects Mn in occupational air under current exposure scenario.
3.2.3.2. Mn-U in workers following Mn dust exposure at Mn mines and ore milling factories
In a study in Singapore, Chia et al. (Citation1993) found “markedly” higher mean Mn levels in urine (measured as µg/L) among 17 Mn-exposed baggers at an Mn ore milling plant (mean Mn exposure: 7.4 years; range: of 1–14 years) compared to 17 referents. Data on correlation between Mn-U and Mn-A was not reported. No correlation was found between neurological outcomes (the Santa Ana dexterity test for the nondominant hand, finger tapping for the dominant hand, the Benton visual retention test, the digit symbol test, the pursuit aiming test (correct and sum), and the trail making test) and Mn-U concentrations despite the existing significant differences in Mn-U between the Mn-exposed and control groups. Main limitations of this study, mentioned above, include the small sample size and a potential for selection bias.
Boojar et al. (Citation2002) examined 145 workers employed in a large Mn mine in Iran. Workers were exposed to Mn oxide and Mn silicates and examined at 0, 4.3, and 7.5 years following the start of work (Stage 1, 2, or 3) in parallel with 65 matched controls. The Mn concentrations in urine were significantly higher in the exposed workers than in the controls (p <0.05). Mn concentration in urine of Mn-exposed workers (Stages 2 and 3) increased with increased duration of exposure. However, the Mn workers also had a long-term exposure to high concentrations of Mn from well drinking water and therefore, reported findings could not be exclusively associated with occupational airborne Mn. No information was provided about time lags between Mn-A and Mn-U sampling.
Gan et al. (Citation1988) examined workers from two Mn ore milling plants (n = 22) and three dry-cell battery manufacturing plants (n = 46) in Singapore. The highest exposures to MnO2 were in the Mn mills where workers were exposed to Mn oxide dust with a mean of 4.16 mg/m3. There were no significant differences in the prevalence of the clinical symptoms and signs between the exposed and control groups. Mn-A concentrations correlated significantly with Mn-U concentrations (correlation coefficient, r = 0.77). Mn-U in the exposed group was significantly higher than that in the comparison (control) group (p < 0.001). Mn-U concentrations were not related to age, ethnic group, smoking status, duration of exposure and type of work. The control group was represented by the workers at the dry cell batteries plants (from compressing areas) and may have introduced selection bias. For more details, see Supplemental Material 2, Table S2.2 and Table S2.3.
Summary
Three reviewed studies measured Mn in serum, urine, and hair as biomarker of exposure (Gan et al. Citation1988; Chia et al. Citation1993; Boojar et al. Citation2002). Reported findings suggest a wide variability of Mn-U levels in the subjects exposed to high levels of insoluble Mn oxide particles (4.16 mg/m3) with the highest reported Mn-U concentration at >90 µg/L. However, high urinary Mn concentrations did not necessarily associate with clinical symptoms, and asymptomatic workers did not necessarily have low urinary Mn levels (Gan et al. Citation1988). Gan et al. (Citation1988) suggested that the urinary Mn concentrations reflect the levels of recent exposure and absorption. Finding reported by Boojar et al. (Citation2002) indicate that estimating Mn exposure based only on a single occupational source may exclude a significant proportion of total exposure from other sources. A dietary habit, including drinking water consumption might play a significant role in the internal levels of exposure in Mn exposed workers. Overall, reviewed studies provide inconsistent and limited evidence that Mn levels in urine reflect Mn in occupational air under current exposure scenarios.
3.2.3.3. Mn-H in workers following Mn dust exposure at Mn mines and ore milling factories
In Iran, Boojar et al. (Citation2002) reported that the Mn-H concentrations in 145 miners exposed to insoluble Mn oxide and Mn silicate dust for 0, 4.3, and 7.5 years (stage 1, 2, or 3) were significantly higher than in the 65 controls (p < 0.05). Increased Mn-H concentrations in Mn exposed workers were observed at the highest duration of exposure (stage 2 and 3). However, the Mn exposed workers in this study also had excessive amounts of Mn from well water as the only source of drinking water in this mine located in desert. No details were provided on time lag between Mn-A and Mn-H sampling. For more details, see Supplemental Material 2, Table S2.4.
Summary
Reviewed studies provide limited and inconsistent evidence that Mn levels in serum, urine, and hair reflect Mn in occupational air in workers exposed to insoluble Mn oxide dust at Mn mines and Mn ore milling factories.
3.2.3.4. Mn-U in workers following Mn dust exposure at dry-cell batteries production plants
Three studies assessed Mn in urine and hair (Roels et al. Citation1992; Bader et al. Citation1999; Dietz et al. Citation2001). For more details, see Supplemental Material 3, Table S3.2.
In Belgium, Roels et al. (Citation1992) compared 92 male workers in a dry cell battery plant and 101 age- and area-matched controls (from polymer processing factory but with no work-related exposure to Mn). Mn workers were exposed for an average (geometric mean) of 5.3 years (range 0.2–17.7 years) to a respirable dust concentration of 215 μg Mn/m3 and a total dust concentration of 948 μg Mn/m3. A significant difference in Mn-U concentrations was observed between Mn oxide-exposed and control subjects (0.84 versus 0.09 µg/g creatinine, geometric mean, p < 0.0.001). Seventy one percent of the Mn-U concentrations in the Mn-exposed group exceeded the highest value found in the control group. On a group basis, Mn-U was significantly correlated with current Mn-A (total dust) and Mn-A (respirable fraction) (rs = 0.83, p < 0.05, both). No correlation was reported between Mn-U and external exposure parameters measured as cumulative exposure, Mn-CEI (total dust), Mn-CEI (respirable fraction), and duration of exposure at the individual level. No relationship was found at the group level between the prevalence of the abnormal neurofunctional tests (eye-hand coordination, hand steadiness, and simple reaction time) and Mn-U concentrations. The prevalence of abnormal test results for the three psychomotor performances increased with increasing concentrations of Mn-CEI (respirable fraction, total dust).
In Germany, Bader et al. (Citation1999) examined 100 workers from three dry cell battery factories and 17 control subjects. Mn-A (Inhalable dust) was measured by a stationary active sampling during working activities. The Mn-A concentrations in the three workplace areas (mean) were 4 μg/m3, 40 μg/m3, and 400 μg/m3. Mn-U levels were not different between Mn exposed workers and referents and Mn-U, as a risk factor for abnormal neurological effects, was not examined. The selected control group (former steel production and Mn oxide mill workers) may have introduced selection bias which limited reliability of reported results. Mn-U levels in the control group were higher than in the exposed workers (p < 0.05).
In a related cross-sectional study in Germany, Dietz et al. (Citation2001) compared a group of 11 workers with more than 10 years (with a maximum duration of employment 37 years) of exposure to high levels of Mn oxide (total dust) and in 11 age-matched workers from the same factory (reference group) to examine a relationship between the neurophysiological and clinical investigations and Mn-levels in urine. No significant differences were observed in Mn-U levels between Mn exposed and non-exposed subjects. Further, there was no significant correlation between Mn-CEI and Mn-U concentrations. However, the statistical power of these conclusions is limited due to small samples size. Limited details were provided on the methods and how results were obtained in the study, but the authors refer readers to the study conducted by Bader et al. (Citation1999).
3.2.3.5. Mn-H in workers following Mn dust exposure at dry-cell batteries production plants
Bader et al. (Citation1999) reported that Mn-H (axillary hair, proximal section) correlated with Mn-A in group-based calculations but not on an individual level. Mn-H concentrations in the highly- and low-exposed workers were significantly higher than in the control subjects (both, p < 0.05, U-test). Mn-H in axillary hair was tested instead of scalp hair because axillary hair is less prone to external contamination by Mn dust. Overall, Bader et al. (Citation1999) considered a detection of Mn-H (proximal sections) as a suitable indicator of long-term Mn exposure and concluded that the proximal hair sequence is the most adequate area for monitoring purposes as it reflected the body burden; however, its validity is restricted to a 4-week exposure interval, background variations and analytical techniques problems. Lack of information on the previous exposure at the plant limited generalizability of reported results.
Dietz et al. (Citation2001) did not observed significant differences in Mn-H between Mn-exposed workers (n = 11) and non-exposed subjects (n = 11). There was no significant correlation between Mn CEI and Mn concentration in axillary hair. However, the statistical power of these conclusions is limited due to small sample size (see Supplemental Materials 3, Table S3.3).
Summary
Overall, limited evidence suggests that in workers employed at dry cell batteries production facilities, Mn-U levels are inconsistently related to the Mn-A levels, can differentiate Mn-exposed with non-exposed subjects, as well as reflect the neurofunctional changes in exposed workers. Roels et al. (Citation1992) found a significant difference in Mn-U concentrations (based on creatinine level in urine) between Mn oxide-exposed and control subjects. However, no differences for Mn-U (based on mass basis) were reported between Mn-exposed and Mn non-exposed workers by Bader et al. (Citation1999). A comparison of reported data in the reviewed studies on Mn-U is limited by reported different Mn-U units (e.g. µg/L or µg/g creatinine). Lack of relationship between Mn-U and Mn-A could be, in part, explained by the quick elimination of Mn from urine. Bader et al. (Citation1999) found that Mn-U levels in controls were higher than in workers in the high- and the middle exposure groups and results remained consistent when the Mn-U concentrations were adjusted for urinary creatinine levels. These findings could reflect an individual variability in homeostatic mechanisms, as well as different dietary habits in the investigated groups, or an additional (indirect) contribution of Mn to systemic circulation through other media (e.g. household dust, soil during gardening).
The suitability of Mn analysis in hair for biomonitoring purposes is hampered by high background physiological variation in hair Mn levels and analytical problems (e.g. the external contamination of the matrix, accuracy, precision, specificity, limit of detection), and availability of reference values. It could not be excluded that changes in Mn-H levels could be due to the exogenous factors. Reviewed studies provide limited and inconsistent evidence that Mn levels in urine and hair reflect Mn in occupational air or neurofunctional impairments in Mn-workers exposed at dry-cell batteries production plants.
3.2.3.6. Mn-U in workers following exposure at Mn oxide and Mn salts production plant
In Belgium, three studies examined the suitability of Mn-U as a potential biomarker of occupational inhalation Mn exposure with dose-related associations to adverse neurofunctional outcomes in Mn-exposed workers at Mn oxides and salts.
Roels, Lauwerys, Genet, et al. (Citation1987) examined 141 male workers in a Mn oxide and Mn salts producing plant and 104 matched control subjects recruited from a chemical plant located in the same area (Roels, Lauwerys, Genet, et al. Citation1987). Urine (spot) and blood samples were collected from each worker on the same day with air sampling. The Mn-A concentrations (total dust) were determined in the breathing zone of workers using personal air samplers during 3–8 h (median 7.5 h). The difference in average Mn-U levels between the Mn-exposed and non-exposed groups was almost 10-fold (GM, 1.56 versus 0.15 µg/g creatinine) and statistically significant (p < 0.001). No dose-response relationships were reported between Mn-U and the prevalence of abnormal neurological outcomes (Roels, Lauwerys, Genet, et al. Citation1987). At the end of 8-h work shift, the Mn-B levels did not change significantly, whereas the Mn-U levels dropped rapidly with estimated half-life of Mn in urine to be less than 30 h. Analyses of data grouped by workplace exposure showed that no relationship was observed between Mn-U (individual basis) and current Mn-A, no relationship was observed between Mn-U and Mn-B (individual basis) or between the Mn-U (individual basis) and duration of exposure, between the total Mn-exposed group (n = 141) and duration of exposure (Roels, Lauwerys, Buchet, et al. (Citation1987). However, a moderate but significant positive correlation was found between the GM of Mn-U (group basis) and current Mn-A (r = 0.62, p < 0.05).
Buchet et al. (Citation1993) studied workers from both dry cell production plant (Group 1, n = 29) and Mn oxide and salt producing plant (Group 2, n = 68) compared to a control group (n = 35). A statistically significant weak positive correlation was found between the concentration of Mn-U and homovanilic acid (HVA) in urine (r = 0.20, p = 0 04) of workers exposed to Mn oxide-containing dust at Mn oxide and salt producing plant and in 35 control male subjects. No significant correlation between the level of HVA in urine and Mn in airborne dust (Mn-A (total dust), Mn-A (respirable fraction) or Mn-A-(inhalable fraction) or duration of exposure (Buchet et al. Citation1993). Only a combined group of subjects exposed to Mn oxide from both plants was considered in the analysis. For details on these studies, see Supplemental Materials 4, Table S4.2.
Summary
Three identified studies conducted in Belgium (Roels, Lauwerys, Buchet, et al. Citation1987; Roels, Lauwerys, Genet, et al. Citation1987; Buchet et al. Citation1993) measured Mn in urine and hair. Roels, Lauwerys, Buchet, et al. (Citation1987) and Roels, Lauwerys, Genet, et al. (Citation1987) did not find any dose-response relationship between Mn-U and the prevalence of abnormal neurological outcomes. Reported findings can be explained, in part, by that only very small concentrations of Mn are excreted in urine (up to 1% of daily dose) and urinary excretion of Mn is more related to current Mn exposure. Despite that the mean concentration of Mn-A was only slightly higher in the Mn oxide and salt producing plant than in the dry alkaline battery factory, Mn-U (µg Mn/g creatinine) in workers from dry alkaline battery plant was 60 times lower in comparison to Mn-U in workers from Mn oxide and salts producing plants, but 3 times higher compared to controls [(0.51 (median) versus 31.6 (median) and 0.17 µg/g creatinine), respectively]. These data indicate different solubility and bioavailability of insoluble Mn oxides and soluble Mn salts. Recent uptake of Mn is likely to lead to a rapid increase in Mn-U (Buchet et al. Citation1993). Overall, it could be concluded that Mn-U level is of limited value as an internal marker of exposure at both individual and group levels.
3.2.3.7. Mn in blood components in workers at Fe-Mn and Si-Mn alloy production plants and smelters
Several studies examined the association between occupational exposure to Mn and Mn levels in red blood cells/erythrocytes (Jiang et al. Citation2007; Cowan, Fan, et al. Citation2009; Cowan, Zheng, et al. Citation2009), Mn-iron Ratio (MIR) in erythrocytes (eMIR) (Cowan, Fan, et al. Citation2009; Cowan, Zheng, et al. Citation2009), plasma (Cowan, Fan, et al. Citation2009; Cowan, Zheng, et al. Citation2009), or serum (Smargiassi et al. Citation1995; Mirmohammadi et al. Citation2017). Details of these studies are provided in Supplemental Material 5, Table S5.2).
Mn in erythrocytes (Mn-E) and plasma (Mn-P) has been measured simultaneously in several occupational studies among workers involved in the production of Fe-Mn smelter in China. Cowan, Fan, et al. (Citation2009) compared Mn-exposed Fe-Mn alloy smelter workers (n = 95) with control subjects (n = 106). Mn-E concentrations were significantly higher in the Mn-exposed group than in the controls (both, p < 0.01). Mn-A levels were significantly associated with eMIR (r = 0.77, p < 0.01) and Mn-P-MIR (r = 0.70, p < 0.01. In subsequent studies conducted by Cowan, Zheng, et al. (Citation2009), a total of 323 subjects were recruited from Fe-Mn alloy plants and organized in three groups by exposure levels: control (n = 106), low-exposure (122), and high-exposure (95) groups. The concentrations of Mn-E and Mn-P in Mn-exposed workers were significantly higher than those in control subjects and were significantly associated with Mn-A (r = 0.69, p < 0.01; r = 0.66, p < 0.01, respectively). No correlation was observed between Mn-E and Mn-P with duration of exposure. It was found that the Mn-exposed workers in the low-exposure group performed better in neurofunctional tests compared to controls (in the steadiness test of the subject’s hand (p < 0.01). After inclusion of available independent variables (e.g. age, years of education, sex, income, and years of employment) in the model, only the eMIR values were significantly associated with individual Purdue pegboard (PP) scores as well with standardized composite index of Purdue pegboard (SCI-PP) scores (r = −0.261, p = 0.002). For workers over the age of 40, the eMIR was associated with declined PP performance (r = −0.219, p = 0.069). After age ≥40 years (n = 40) and the plasma-MIR (pMIR) values greater than the pMIR cut-off value (2.75) were included in the linear regression analysis, pMIR became inversely and statistically significant associated with SCI-PP (r = −0.419, p = 0.009).
No significant differences were observed between levels of Mn in RBCs and plasma Mn of the Mn oxide exposed workers (n = 18) from a Mn Fe-alloy factory compared to control subjects (n =9) in the earlier study conducted in China by Jiang et al. (Citation2007). During a 1-year follow-up study, air Mn level in the breathing zone decreased due to an improved ventilation and was 0.26 mg/m3 (the GM) and close to the MAC value (0. 2 mg/m3) but Mn in RBCs among eight smelter workers, who were originally in the high Mn-exposed group, remained unchanged. However, a small sample size limited a statistical power of reported result.
Mirmohammadi et al. (Citation2017) examined a possible correlation between Mn-A and Mn-Se levels in 35 workers from an iron foundry compared to 35 control subjects employed at the Fe-Mn foundry factory in Iran. No correlation between the Mn-A and Mn-Se concentrations in the exposed subjects was reported. Limitations in study design and reporting decrease generalizability of reported findings.
Summary
Erythrocytes contained about 66% of the total amount of Mn in whole blood, the leucocytes, and platelets 30% and plasma less than 5% (Milne et al. Citation1990). Mn in plasma represents the most bioavailable fraction of Mn in the whole blood and presumably exists in a steady state with Mn in the brain and basal ganglia. Reported findings suggest that pMIR and eMIR can be sensitive indicators of internal Mn exposure and assessment with pMIR appeared to be more sensitive than eMIR (Jiang et al. Citation2007). However, because Mn-E are relatively high compared to Mn in serum or plasma, hemolysis during blood sampling, storage, or analyses would increase Mn levels in plasma/serum and compromise results. Due to low levels of Mn in plasma and serum, analytical criteria (the accuracy, precision, specificity, detection limit, and linearity), problems of the analytical technique (interference, matrix contamination), reference values and their physiological variations are critical. A short half-life of Mn in plasma limited the practical applicability of Mn levels in plasma/serum as a risk factor of long-term abnormal neurological outcomes.
Overall, results from reviewed studies suggest that Mn-E, Mn-P, and Mn-Se can be useful for discriminating occupationally exposed and control subjects on a short-term basis and during, approximately, 1 month after cessation of exposure, but are not suitable to assess a long-term Mn exposure on an individual basis. Available data from reviewed studies also suggest that the evidence of a relationship between Mn-E, Mn-P, or Mn-Se concentrations and Mn-A or neurofunctional impairments are limited, inconsistent, and poor. Mn levels in these biological fluids cannot be considered as reliable biomarkers of Mn exposure in workers exposed mainly to Mn oxide dust at the Fe-Mn and Si-Mn alloy production plants and smelters.
3.2.3.8. Mn-U in workers at Fe-Mn and Si-Mn alloy production plants and smelters
Mn in urine as a biomarker of external exposure with dose-related associations to neurofunctional impairments was measured in several occupational studies (Brown et al. Citation1991; Hua and Huang Citation1991; Mergler et al. Citation1994; Lucchini et al. Citation1995; Smargiassi et al. Citation1995; Lucchini et al. Citation1999; Apostoli et al. Citation2000; Bouchard et al. Citation2003; Ellingsen, Hetland, et al. Citation2003; Myers, Thompson, Naik, et al. Citation2003; Bast-Pettersen et al. Citation2004; Cowan, Fan, et al. Citation2009; Cowan, Zheng, et al. Citation2009; Hassani et al. Citation2016). Details of these studies are provided in Supplemental Material 5, Table S5.3.
Mn-U levels in Mn-exposed workers were significantly higher compared to control subjects in four studies conducted in Italy (Lucchini et al. Citation1995; Smargiassi et al. Citation1995; Lucchini et al. Citation1999; Apostoli et al. Citation2000), two studies conducted in Norway (Ellingsen, Hetland, et al. Citation2003; Bast-Pettersen et al. Citation2004) and one study in China (Cowan, Fan, et al. Citation2009; Cowan, Zheng, et al. Citation2009). However, no significant differences in Mn-U concentrations between Mn-exposed workers and referents were reported in Iran (Hassani et al. Citation2016), in Canada (Mergler et al. Citation1994; Bouchard et al. Citation2003), and in South Africa (Myers, Thompson, Ramushu, et al. Citation2003).
Mn-U of Mn exposed workers were associated with current levels of exposure (Apostoli et al. Citation2000; Ellingsen, Hetland, et al. Citation2003; Myers, Thompson, Naik, et al. Citation2003) or cumulative exposure levels (Lucchini et al. Citation1995; Myers, Thompson, Naik, et al. Citation2003). Ellingsen, Hetland, et al. (Citation2003) observed an association between Mn-U levels and soluble fractions of the Mn-A inhalable and respirable dust. Levels of Mn-U were significantly correlated to the duration of exposure in the workers employed at Fe-Mn alloy smelting plants in South Africa (Myers, Thompson, Naik, et al. Citation2003). No correlation between the Mn-U and current or cumulative Mn-A exposure levels, duration of exposure was found in other reviewed studies (Smargiassi et al. Citation1995; Lucchini et al. Citation1999; Apostoli et al. Citation2000; Cowan, Fan, et al. Citation2009; Cowan, Zheng, et al. Citation2009; Hassani et al. Citation2016). No association between Mn-U levels and insoluble fractions of airborne Mn (both Inhalable and respirable dust particulates) reported by Ellingsen, Hetland, et al. (Citation2003). After transformation of Mn-U and the Mn CEI levels in the logarithmic form, Lucchini et al. (Citation1995) observed a correlation between log Mn-U and log Mn CEI (r2=0.16, p = 0.0017). Reported results of a significant correlation between Mn-A (as Mn-CEI) and Mn-U on an individual basis are in discrepancy with the Roels, Lauwerys, Genet, et al. (Citation1987) and Roels et al. (Citation1992) previous data of significant correlation between Mn-U and current Mn-A exposure on a group basis only. According to Lucchini et al. (Citation1995), it could be explained, in part, by exposure to different Mn compounds and its different bioavailability. The t50 of Mn oxide deposited in the lung alveoli is approximately 2–3 months, however, t50 for Mn soluble salts (e.g. Mn sulfate, Mn chloride) at the Mn salts production plant are shorter (Oberdoerster and Cherian Citation1988).
Summary
Review of available data suggests that the evidence of a relationship between Mn concentrations in urine and external air Mn exposure or neurofunctional impairments is inconsistent and poor and Mn-U cannot be considered as reliable biomarkers of Mn exposure in ferro- and silico manganese alloy production plants and smelters.
3.2.3.9. Mn in bone, nails, hair, and saliva in workers at Fe-Mn and Si-Mn alloy production plants and smelters
Several studies examined the suitability of Mn in bone, nails, and hair as potential biomarkers of Mn exposure (Hua and Huang Citation1991; Cowan, Fan, et al. Citation2009; Cowan, Zheng, et al. Citation2009; Hassani et al. Citation2016; Rolle-McFarland et al. Citation2018; Rolle-McFarland et al. Citation2019). Details of these studies are provided in Supplemental Material 5, Table S5.4.
Mn concentrations scalp hair (Mn-Scalp), Mn-Sa, Mn-Fn, Mn-Tn were significantly higher in the Mn alloy smelter workers compared to the controls (Hua and Huang Citation1991; Cowan, Zheng, et al. Citation2009; Hassani et al. Citation2016; Rolle-McFarland et al. Citation2018) but not in bone (Rolle-McFarland et al. Citation2018). No relationship was found between Mn-A and Mn in scalp hair, duration of exposure and Mn-Sa or Mn in Mn-Scalp (Cowan, Zheng, et al. Citation2009), Mn CEI and in scalp hair (Rolle-McFarland et al. Citation2018) or Mn-Sa and duration of exposure (Cowan, Zheng, et al. Citation2009),. A relationship between Mn-A concentrations and Mn-Sa (r = 0.77, p < 0.01) was reported by Cowan, Zheng, et al. (Citation2009), between Mn CEI (16 years) and Mn-Bn or Mn-Fn concentrations (rs = 0.44, p < 0.01 and rs = 0.44, p < 0.01, respectively) was observed by Rolle-McFarland et al. (Citation2018). Hassani et al. (Citation2016) observed a correlation between toenails Mn (Mn-Tn) levels and neurobehavior symptoms (Q16, number of positive questions, p < 0.001, Mann–Whitney test) and significant inverse relationships between neurocognitive outcome measures (Spatial Working Memory Double error) and Mn-Tn (p = 0.012). Hassani et al. (Citation2016) concluded that toenails Mn levels could be used to distinguish Mn-exposed workers from unexposed subjects at the group level. Using regression models adjusted for age, education, factory of employment, and smoking status, Rolle-McFarland et al. (Citation2019) found that levels of Mn in bone were significantly associated with decreased performance on average AVLT (Auditory Verbal Learning Test) scores and Animal Naming scores. Mn-Bn and Mn-Fn were associated with cognitive function in Mn-exposed workers. Mn-Bn or Mn-Fn were not significantly associated with olfactory function. Similar to other studies, the major limitations of these cross-sectional studies include small sample size and lack of data on dietary Mn exposure patterns among workers. In addition, exogenous metal contamination on biological samples could not be excluded.
Summary
Based on the review of available data, it can be concluded that the evidence of a relationship between Mn concentrations in bone, nails, hair, saliva, and airborne Mn or neurofunctional impairments is inconsistent and limited and Mn levels in these biological fluids and tissues cannot be considered as reliable biomarkers of Mn exposure in ferro- and silico Mn alloy production plants and smelters.
3.2.3.10. Mn in blood components in welders exposed to welding fumes
Several studies have examined the suitability of Mn-Se as potential markers of occupational Mn exposure and adverse health effects (Jarvisalo et al. Citation1992; Li et al. Citation2004; Lu et al. Citation2005; Wang et al. Citation2008; Ellingsen et al. Citation2013; Ellingsen, Chashchin, et al. Citation2014). Details of these studies are provided in Supplemental Material 6, Table S6.2.
Levels of Mn in serum of the current welders were significantly greater compared to the referents or the former welders with manganism but the levels of Mn in serum of the former welders were not significantly different from those of the referents (Ellingsen et al. Citation2013; Ellingsen, Chashchin, et al. Citation2014). In multiple regression analysis that included the current welders and referents, Mn-Se was associated with Mn exposure (Ellingsen, Chashchin, et al. Citation2014). In the current welders, Mn-Se significantly correlated with blood Mn (r = 0.69) and Mn in urine (r = 0.64); no correlations among the biomarkers were seen in the former welders or in the referents (Ellingsen et al. Citation2013). In multiple regression analysis that included the current welders, Mn-Se was significantly associated with years of welding, as well as with levels of biologically accessible Mn in air collected two days before the biological sample collection (Ellingsen et al. Citation2013; Ellingsen, Chashchin, et al. Citation2014). No significant correlation was observed between Mn-Se levels and duration of exposure (<10 years, 10 years, >10 years) (Li et al. Citation2004; Lu et al. Citation2005; Wang et al. Citation2008) or between Mn-Se and Mn-A (respirable) (rs = −0.12, p = 0.14) (Stanislawska et al. Citation2020). However, a significant negative weak correlation was observed between Mn-Se and Mn-A (inhalable) (rs = 0.04, p = 0.77) (Stanislawska et al. Citation2020). A significant correlation between plasma Mn and Mn-A (Mn-A > 10 μg/m3 (personal sampling with samplers under helmet during a full shift) was reported by Hoet et al. (Citation2012). The authors reported that on the first day of the workweek, a plasma Mn value of 2 g/L could distinguish Mn-air exposure above or below 20 g/m3 with a sensitivity of 69% and a specificity of 82%. However, the relationship between Mn-A and plasma Mn on the second day of workweek was less strong compared to Monday which suggest that plasma Mn reflects current exposure. No association between plasma Mn Mn CEI was found in Bowler, Nakagawa, et al. (Citation2007); Bowler, Roels, et al. (Citation2007) and Smith et al. (Citation2007).
Levels of Mn in serum of the current welders were significantly greater compared to the control subjects in a number of studies (Jarvisalo et al. Citation1992; Li et al. Citation2004; Lu et al. Citation2005; Wang et al. Citation2008; Stanislawska et al. Citation2020). Stanislawska et al. Citation2020 reported that the main component of the welding fumes in the inhalable fraction of Mn-exposed welders was Fe (78.1%), followed by compounds of chromium in the air (8.5%), Mn-A (8.3%), and Ni-A (5.2%). Bowler, Roels, et al. (Citation2007) found that Mn in blood was 9.6 (mean) µg/L, range: 5.13–15.3 µg/L, 43% of welders had Mn-B >10 µg/L, however, plasma Mn levels were normal [mean (SD): 0.58 (0.13) µg/L, range: 0.22–0.85 µg/L]. In a subsequent study in bridge welders, Smith et al. (Citation2007) did not report an association between plasma with cumulative respiratory exposure index. In welders, 6% (range 3–9%) of whole blood Mn was contained in the plasma fraction, however, no association between whole blood and plasma Mn levels was observed (Pearson’s r = 0.258, p = 0.12). No control group was included in both studies. Levels of plasma Mn in the current welders were significantly greater compared to the control subjects in the studies conducted by Hoet et al. (Citation2012). A possible association between Mn-Se or plasma Mn and neurological impairments was not studied in these studies.
Based on review and analysis of available evidence, it could be concluded that the evidence of a relationship between Mn concentrations in erythrocytes, serum, plasma and airborne Mn or neurofunctional impairments is inconsistent and poor. Thus, Mn levels in these biological matrices cannot be considered as reliable biomarkers of Mn exposure or neurofunctional impairments at target occupational settings and exposure scenarios.
3.2.3.11. Mn-U in welders exposed to welding fumes
Several studies on welders have examined the suitability of Mn in urine as potential biomarkers of occupational Mn exposure (Jarvisalo et al. Citation1992; Sjogren et al. Citation1996; Barrington et al. Citation1998; Li et al. Citation2004; Halatek et al. 2005; Ellingsen et al. Citation2006, Bowler, Roels, et al. Citation2007; Ellingsen et al. Citation2007; Ellingsen et al. Citation2008; Laohaudomchok et al. Citation2011; Hoet et al. Citation2012; Ellingsen et al. Citation2013; Ellingsen, Chashchin, et al. Citation2014; Ellingsen, Kusraeva, et al. Citation2014; Hassani et al. Citation2016; Ellingsen et al. Citation2019). Details of these studies are provided in Supplemental Material 6, Table S6.3.
No differences between Mn-U concentrations in the Mn-exposed welders and controls were reported in three studies (Halatek et al. 2005; Ellingsen et al. Citation2006; Hoet et al. Citation2012). Hoet et al. (Citation2012) reported that urinary Mn concentrations were below 20 μg/L (LOQ) in 65% of Mn-exposed welders and in 80% of control referents. No correlation was reported between Mn-U in active and former welders and between Mn-U levels and Mn CEI or Mn-A concentrations (Bowler, Roels, et al. Citation2007; Smith et al. Citation2007; Hoet et al. Citation2012). No significant correlations between Mn-U and Mn-A were observed in boilermakers (welding school) after work shift following 1–6, 7–9, 10–12, 1–12 months duration of exposure, however, pre- and post-work shift Mn-U concentrations and Mn-A were significantly correlated (Laohaudomchok et al. Citation2011). No association was reported between Mn-U levels and duration of exposure (Barrington et al. Citation1998; Li et al. Citation2004) or between Mn-U and current Mn-A or Mn CEI (Jarvisalo et al. Citation1992). Mn-A (Total dust) (the mean of days 1 and 2 before the collection of biological samples) were significantly correlated with Mn-U in multiply linear regression analysis (r = 0.39, p < 0.05) (Ellingsen et al. Citation2013). The correlation was significant between the Mn-U concentrations and Mn-B (Pearson’s correlation coefficient r = 0.63) and between Mn-U and Mn-Se (r = 0.64). The Mn-U concentrations (mg/L) were around 21% of the Mn-Se concentration in the control subjects and 67% in the welders (p < 0.001) (Ellingsen et al. Citation2013). It has been shown that the fluoride content of the welding fume contributes to the increased solubility of Mn, but other components used as fluxing agents in welding rods like potassium (K) and sodium (Na) may also contribute to the increased Mn solubility and bioavailability. Hassani et al. (Citation2016) reported a statistically significant association between Mn-U and Mn-A concentrations in gas transmission pipeline welders. No analysis of association between Mn-U and neurofunctional impairments were performed in most reviewed studies due to lack of Mn-U differences between Mn-exposed workers and controls.
Summary
Based on review of available evidence, it could be concluded that the evidence for a relationship between Mn concentrations in urine and airborne Mn or neurofunctional impairments is inconsistent and poor. Thus, Mn-U cannot be considered as a reliable biomarker of Mn exposure at target occupational settings and exposure scenarios.
3.2.3.12. Mn in bone, hair, and saliva in welders
Numerous studies on welders have examined the suitability of Mn in bone, hair, and saliva as potential biomarkers of occupational Mn exposure. Six of the reviewed studies evaluated the correlation of Mn in toenails with other biological media collected concurrently and included a wide range of participants (from 30 to 868) (Wang et al. Citation2008; Cavallari Citation2010; Laohaudomchok et al. Citation2011; Grashow et al. Citation2014; Ward et al. Citation2018; Vinnikov et al. Citation2018). Details of these studies are provided in Supplemental Material 6, Table S6.4.
Laohaudomchok et al. (Citation2011) examined correlations between Mn exposure and Mn concentrations in toenails collected from all ten toes of 49 welders recruited from a boilermaker union (preliminary results were reported in a research report by (Cavallari Citation2010). Mn-Tn was significantly correlated with Mn-CEI for exposure windows 7 to 12 months prior to the toenail clipping date. There was little correlation between Mn-Tn and Mn-CEI for the 1-6-month exposure window or for the whole 12-month period. Grashow et al. (Citation2014) used the same set of population of boilermakers from Laohaudomchok et al. (Citation2011) study but used slightly different exposure windows (1–3, 4–6, 7–9, 10–12 and 1–12 months) and a simplified exposure metric (exposure hours rather than Mn CEI). Mn-Tn was significantly associated only with hours of welding 7-9 months prior to toenail clipping. The authors note that, in this occupational group, Mn-Tn reflects intermediate-term exposures better than exposures occurring over many years. Ward et al. (Citation2018) analyzed Mn in toenails as a marker of exposure to respirable Mn in 45 male career welders recruited from a semi-trailer manufacturer, and 35 age-matched male referents recruited from the same manufacturer. Mn CEI was based on full-shift personal air sampling and work history data collected from a questionnaire. Information from a food frequency questionnaire was used to estimate dietary Mn intake. Mn CEI was computed for 3 exposure windows: past 3 months, 7–12 months, and over a lifetime. The average respirable airborne Mn concentration, Mn CEI for all three exposure windows, and Mn-Tn were significantly higher in the welders than in the referents. A linear mixed regression analysis showed a significant association between Mn-Tn and Mn CEI for time window 7-12 months prior to toenail clipping. Mn-Tn was not significantly associated with Mn CEI for past 3 months, Mn-CEI over a lifetime, or with estimated dietary intake of Mn. A receiver operating characteristic (ROC) curve analysis revealed that a cutoff value of 4.14 µg/g Mn-Tn best discriminated between welders and referents. A threshold value for Mn-Tn of 4.66 µg/g best discriminated between subjects exposed to Mn concentrations above and below the ACGIH TLV of 0.02 mg/m3. The authors concluded that Mn-Tn may serve as a sensitive and specific biomarker of occupational exposure to Mn. Hassani et al. (Citation2016) revealed strong positive correlations between Mn levels in toenails and urine (r = 0.65) and blood (r = 0.65). No relationships were found between Mn levels in toenails and whole blood in welders (Wongwit et al. Citation2004). Coelho et al. (Citation2014) found a weak positive correlation between toenails and fingernails Mn (r = 0.23). Limitations associated with the use of Mn-Tn as a marker of exposure include variations in toenail growth for different toes and among individuals (Grashow et al. Citation2014; Ward et al. Citation2018), subjects need to allow their toenails to grow for at least 2 weeks, and it is unclear how the use of personal hygiene (foot) products affects tissue metal concentrations (Ward et al. Citation2018).
Summary
In summary, the authors of the studies summarized in this section suggest that, in some circumstances, Mn in toenails of welders may serve as a biomarker of occupational cumulative exposure to Mn that occurred 7–12 months earlier. Mn in toenails may be sensitive enough to discriminate occupationally exposed welders from unexposed subjects, as well as subjects exposed to Mn above the ACGIH TLV of 0.02 mg/m3 from subjects exposed to concentrations below this value. However, available data are insufficient to establish a dose-response relationship. In addition, Mn CEI calculations based on the retrospective work histories could introduce errors in exposure estimates. Such errors would reduce the correlations between Mn biomarkers and Mn-CEI. Practical advantages of toenails compared to other biological materials include ease of collection, transport, and storage (Laohaudomchok et al. Citation2011; Grashow et al. Citation2014; Ward et al. Citation2018). Although hair and fingernails share these advantages, they are thought to be more subject to external contamination (Laohaudomchok et al. Citation2011). Gutiérrez-González et al. (Citation2019) highlighted common limitations of studies on toenails as biomarkers of exposure: lack of standardized protocol on toenail preparation, analyses, and reporting, and certified reference material for evaluation of the results. In addition, the lack of information about sample mass in many studies limited a comparison of reported data, as the limit of detection (LoD) and the Mn concentrations in toenails are influenced by sample size.
In summary, only limited studies reported a significant difference between Mn-exposed and control groups, or a significant dose–response between Mn in biological fluids (other than whole blood) and tissues with current or cumulative Mn-A exposure and/or neurofunctional impairments. Reported internal Mn levels are inconsistently related to Mn-A or to effects on CNS functions in different exposure scenarios at different occupational settings. It can be concluded that the available evidence for a relationship between external Mn exposure and internal (biomarker level) exposure is limited and inconsistent. None of the reviewed potential biomarkers can be considered as a reliable biomarker of Mn exposure or/and neurofunctional impairments at different occupational settings and exposure scenarios.
3.2.4. Solubility and bioavailability of Mn compounds
Bioavailability of Mn compounds are particularly important for assessing the “internal dose” of Mn exposure and the risk of neurotoxic effects in workers exposed to different Mn compounds. Physico-chemical characteristics of Mn, solubility and bioavailability of Mn compounds are important determinants of its absorption, distribution, retention, excretion, and toxicity (Aschner et al. Citation1999).
Bioavailability of Mn compounds are particularly important for assessing the internal dose of Mn exposure and the relative risk of neurotoxic effects in workers exposed to different Mn compounds. In occupational setting, workers are exposed to dust or fumes of different Mn compounds with different solubility. Buchet et al. (Citation1993) studied excretion of Mn concentrations in the urine of 68 male workers exposed to Mn-containing dust (mainly MnO2) in a dry alkaline battery plant or an MnO2 and Mn soluble salts producing plant, as well as in 35 control subjects. The technological work processes in both plants had not been modified in the prior 15 years. The workers were exposed to inhalable (total) dust at 0.95 and 1.37 mg/m3 in the dry alkaline battery plant and the MnO2 and Mn salt producing plant, respectively. Mn concentrations in air were relatively similar in both plants; however, Mn levels in urine of workers from the Mn oxides and soluble salts production plants (GM: 31.6 µg/g creatinine; range: 3.1 to 301.6 µg/g creatinine) was higher compared to Mn levels in urine of workers from dry alkaline battery plant (GM: 0.51 µg/g creatinine; range: 0.06–10 µg/g creatinine). Buchet et al. (Citation1993) concluded that, based on Mn levels in urine, the bioavailability of Mn from soluble salts is higher than that of insoluble Mn oxide. Following lower exposure to Mn in blood, the dry alkaline battery workers had less adverse health effects compared to the workers from Mn oxides (MnO, MnO2, Mn3O4) and soluble Mn salts (MnSO4, MnCO3, Mn (NO3)2, Mn acetate) producing plant (Roels, Lauwerys, Genet, et al. Citation1987; Roels et al. Citation1992). Dorman et al. (Citation2001) reported that inhalation exposure to soluble forms of Mn (MnSO4) results in higher brain Mn concentrations in rats than those achieved following exposure to an insoluble form of Mn MnO2). In rats, the uptake of Mn was faster and the peak concentrations in blood after administration of soluble MnCl2 were higher compared to insoluble Mn3O4 or MnO2 (Drown et al. Citation1986; Roels et al. Citation1997). The Mn uptake by the striatum was higher after the intratracheal instillation of MnCl2 compared to the equal amount of MnO2 (Roels et al. Citation1997). These findings suggest that Mn soluble compounds have a different uptake and distribution pattern compared to the insoluble compounds, particularly in the lung, with a different primary mechanism of toxic action (systemic versus local) and health outcomes. Welders exposed to a certain level of soluble Mn in welding fumes are subject to a higher absorption rate of Mn into systemic circulation and accumulation in the brain as compared to workers exposed to insoluble MnO2 particulates (Ellingsen et al. Citation2019). It can be suggested that a spectrum of adverse effects, latent period before appearance of pre- and clinical symptoms, and exposure thresholds will be different in workers exposed to insoluble Mn compounds compared to workers exposed to more soluble and bioavailable Mn compounds. These data highlighted the importance to consider physical and chemical properties and, particularly, solubility and bioavailability of Mn compounds in the exposure and human health risk assessment.
4. Overall summary and conclusions
The present critical review was undertaken following a systematic approach to identify existing trends and consolidate available evidence on the relationship between occupational inhalation exposure to Mn under different exposure scenarios at occupational settings and Mn concentrations in the whole blood primarily. The review also summarized evidence on other body fluids or tissues with limited use in clinical practice for diagnosing of Mn long-term intoxication (e.g. erythrocytes, serum, plasma, urine, toenails, fingernails, bone, saliva, and scalp, axillary, and pubic hair). Our review also examined the relationship between internal Mn exposure and abnormal neurological outcomes using the same consideration.
Mn is mostly used in the steel and welding industry. Most of the studies in this review focused on welders and on workers producing Fe-Mn/Si-Mn alloys; a limited number of studies in other occupational settings, such as mines, dry cell battery manufacturing, refineries, and Mn oxide and Mn soluble salts production plants were also identified.
The 76 articles identified in this review describe Mn levels in blood and other biological matrices. In the various studies, workers were exposed to insoluble and soluble Mn-containing dust and Mn-containing fumes in different exposure scenarios. Human data are considered to be more relevant than animal data in relation to the mechanism of neurotoxic effects of Mn and the use in risk assessment (ATSDR Citation2012). Therefore, in this review, the focus was on the critical evaluation of human studies.
A number of relevant occupational cross-sectional and longitudinal studies in workplace settings investigated the relationship between internal blood Mn and external Mn exposure and/or neurotoxic effects. Several studies that only investigated the relationship between external exposure and blood Mn or blood Mn and neurotoxic effects were also included in the analysis.
In this review, we followed the three proposed criteria of reliable biomarkers of Mn exposure, as described by Hoet and Roels (Citation2015). provides summaries of the evidence for Mn in blood according to the three criteria, which focus on distinguishing exposed and non-exposed workers, demonstrating a dose-related association with external exposure, and demonstrating a dose-related association with neurofunctional impairment.
In the reviewed studies, most authors reported significantly higher mean Mn levels in Mn-exposed workers compared to the controls. However, reported findings are inconsistent at different exposure scenarios. Only limited evidence is available regarding the suitability of using Mn levels in blood as a biomarker of occupational Mn exposure at the individual level Most of the studies reported a relationship between Mn external and Mn internal exposures at the group level but not at the individual levels. Because only a limited number studies reported (1) a significant dose–response between Mn-B and current or cumulative Mn-A exposure and/or neurofunctional impairments and (2) values that are inconsistently related to Mn in the air or to effects on CNS functions in different exposure scenarios, it could be concluded that the relationship between external Mn exposure and internal (biomarker level) exposure is limited and inconsistent. Based on the totality of available evidence, lack of consistency in the reported findings across different exposure scenarios, the evidence for Criteria 1 can be considered as “modest, inconsistent,” for Criteria 2 as “some, inconsistent, limited,” and for Criteria 3 as “poor, inconsistent, limited.”
Inconsistencies in the reported relationship between the concentration of Mn in blood and external Mn exposure can be associated with a tight homeostatic regulation of Mn in blood, as an essential element, and individual and inter-individual variability in homeostatic regulation. The design and methodological approaches of the reviewed studies varied greatly, with critical differences in the reported details on the profile of external Mn exposure, inhalation exposure characterization; presentation of Mn exposure levels (e.g. current exposure levels or cumulative exposure index) are not always specified, all of which limited a direct comparison and interpretation of results. Lack of or limited details on the type and duration of air Mn sampling, the location of samplers (e.g. in welders), the type of sampler’s head used in the measurement of total dust particulates, reported time lag between air Mn and blood sampling, whether statistical analyses were done on an individual or on a group basis, and the use and type of respiratory protection often prevents a comparison of results. In addition, a comparison of workplace exposure previously evaluated by “total” Mn with the current air sampling data measured as the inhalable and respirable particulates of Mn in the breathing zone of the exposed workers remains challenging.
Studies investigating the effects of occupational exposure to Mn-containing dust or fumes and association between blood Mn (or other biological fluids and tissues) and neurofunctional impairment are limited by many methodological issues. The incomplete characterization of the chemical form and solubility of Mn compounds and particle size are common weaknesses in the occupational studies. Lack of consistency in the reported dose-response relationship between Mn-B and neurofunctional impairment is not only due to Mn physico-chemical properties but also a result of inconsistencies in 1) the lag time between blood Mn and neurofunctional testing; 2) the lack of consistency in the selection of neurofunctional tests; 3) the lack of knowledge on causality of Mn in the development of pre-clinical signs of low dose Mn inhalation exposure (most experimental studies are conducted with soluble Mn salts administered as solutions and at high doses versus real-life exposure to insoluble Mn compounds as dust particulates at concentrations near or lower existing time weighted average); 4) the lack of specific and sensitive tests to detect the early signs of neurological impairments. In addition, the prevalence of cross-sectional human studies and lack of information to control for confounding factors in the final analysis limited interpretation of the reported findings and an ability to relate blood Mn to the observed neurological impairment. Mechanisms of action and diversity of adverse and non-adverse effects following exposure to Mn, particularly at low doses, are poorly understood and clinical significance of the reported findings on associations between Mn in biological fluids and tissues is rarely discussed in the reviewed studies, which further limits its use in clinical practice and risk assessments.
Exposure to Mn fumes represents a separate and a different type of exposure compared to the exposure to Mn particulates. In rare cases, workers, especially in welding industry, are exposed solely to Mn-containing compounds and exposure information is often not adequate to rule out other toxic substances as the cause of the observed effect. In addition, even though welders are mainly exposed to the insoluble Mn oxides, co-exposure to other hazardous chemicals may modify particulates solubility and increase Mn uptake into blood.
Overall, findings reviewed in this study suggest that Mn in blood cannot be considered as a reliable biological indicator of external Mn exposure or Mn-related neurofunctional impartment(s) in workers exposed to Mn-containing dust or fumes at high or low exposure levels. No new reliable data were identified in the recently published literature to change the conclusions of this review. We note that this conclusion applies to the literature that was reviewed, which is limited to Mn occupational exposure studies. Animal studies which form the basis for pharmacokinetic models for Mn, indicate that blood Mn is a reasonable indicator for Mn inhalation exposure under controlled experimental conditions (Ramoju et al. Citation2017).
Results of the reviewed studies mirror the clinical, toxicological, and occupational exposure challenges of establishing exposure levels to Mn-containing dust and/or fumes and which exposure levels cause Mn to exceed a plateau level of homeostatic control before leading to early clinical signs of intoxication in workers. Because Mn levels in biological fluids could not adequately reflect an exposure level, Mn concentrations in blood of the exposed workers might mask a true risk.
WHO (Citation1981) estimated a systemic Mn absorption of 180 μg/d in healthy Western adult based on an average oral intake of 3.6 mg/day Mn via food and a gastro-intestinal absorption rate not higher than 5%. In case of the impairment of the homeostatic control over the systemic Mn status, the Mn absorbed via the gastrointestinal tract would lead to a concentration of about 40 μg/L of circulating blood (assuming 4.5 L of blood in the systemic circulation). A high level of Mn (>40 µg/L) was proposed to confirm chronic Mn intoxication in workers with medium and high exposure levels (IRSST Citation2005). In Germany, a BAR (Biologischer Arbeitsstoff-Referenzwert or BRV, Biological Reference Value) of 15 μg/L blood has been established by the MAK Commission. This value represents Mn concentrations in the working-aged general population (95th percentile) not occupationally exposed to Mn.
Recently, Mn in urine, bones, toenails, fingernails, saliva, and hair were suggested as biological markers of external Mn exposure and potential indicators of neurofunctional impairment(s) in the exposed workers. However, their clinical significance remains unknown. Despite the finding that 95% of Mn in blood is found in the cellular fraction of blood, Mn in erythrocytes is not a good indicator of tissue accumulation or body burden. While some correlations between Mn-B and/or erythrocytes and external exposure levels/adverse health effects have been reported, overall, the relationship between Mn concentrations in whole blood and external exposure levels is inconsistent and poor. Plasma Mn reflects the bioavailable fraction of Mn in blood which clears very quickly; therefore, the relationship between plasma Mn and external exposure or long-term neurotoxic effects is also inconsistent and poor. Mn to iron ratio (MIR) in erythrocytes appears to be a stronger indicator of exposure than the ratio MIR in plasma. Recently published reviews and identified original studies suggest that Mn in urine is not a reliable marker of exposure. Mn is primarily excreted via bile and urinary Mn excretion is low (<1%) and is a subject to wide inter-individual and diurnal variations, with Mn-U being poorly correlated to both the external Mn exposure level and adverse health effects. In addition, in most studies, Mn-U levels are adjusted to the creatinine excretion rate in urine. However, a creatinine excretion rate is influenced by many various factors (race, gender, age, physical activity, and muscle mass) and it is not clear if Mn is excreted in urine via the same passway as creatinine. Changes in saliva Mn reflect those in Mn-Se. An advantage of evaluating Mn in saliva is that it is a noninvasive biomarker. A review of the identified relevant data suggest that Mn-Bn might serve as a biomarker of cumulative Mn exposure (Mn accumulates in bone tissue and t1/2 of Mn in human bone is estimated to be approximately 8–9 years). In-vivo Neutron Activation Analysis measuring Mn levels in human bone has been applied for assessing past cumulative exposures to Mn. At this time, the method can differentiate between exposed and non-exposed subjects in occupational settings; however, measurement precision is not sufficient for assessing exposures on an individual level. While some studies have found a correlation between exposure level and Mn in hair, use of hair is problematic mainly due to a risk of exogenous contamination and the sensitivity of current analytical methods. It is proposed that hair might serve as a marker of exposure for 4–8 weeks only, potentially due to hair growth and loss. As a biological indicator, Mn in toenails of welders may be sensitive enough to discriminate occupationally exposed welders from unexposed subjects, as well as subjects exposed to Mn above the American Conference of Governmental Industrial Hygienists (ACGIH) TLV of 0.02 mg/m3 from subjects exposed to concentrations below this value (ACGIH Citation2012). However, data on nail Mn concentrations are insufficient to determine dose–response and/or dose–effect relationships with external exposure or effects on CNS. Although fingernails could be easily obtained by non-invasive techniques for research purposes, their scientific and practical significance for clinical diagnosis are problematic as they may be influenced by background Mn levels, external contamination, as well as by nail growth and loss processes.
Based on available evidence, including toxicokinetic properties of Mn species, and the inadequacy of current data, the usefulness of biological indicators of Mn exposure alone in clinical practice or occupational health screening remains questionable. However, the current state of research in this area suggests that Mn biomarkers in whole blood may be useful in biomonitoring and risk assessment. Further research on biomarkers of Mn exposure and biomarkers of neurofunctional impairment will serve to clarify the most appropriate biomarkers for use in practice.
Supplemental Material
Download MS Word (305.9 KB)Acknowledgements
The authors would like to thank Dr. Athena Keene from Afton Chemical, a member company of the International Manganese Institute (IMnI), for helpful comments on preliminary drafts of this article; although Dr. Keene also serves as the Afton Chemical representative on the IMnI Health, Safety, and Environment Committee, her comments were provided as an independent scientist with expertise in manganese toxicity. The authors gratefully acknowledge the comments of the three external reviewers selected by the editor and anonymous to the authors. As a result of their comments, we limited the scope of the paper and made a number of changes that improved the original draft of this article.
Declaration of interest
This work was conducted under a research contract to review the recent scientific literature on manganese biomarkers between the International Manganese Institute (IMnI, www.manganese.org), an industry association representing the international manganese industry, and Risk Sciences International (RSI, www.risksciences.com), a Canadian company established in 2006 in partnership with the University of Ottawa. Additional financial support was provided by the Natural Sciences and Engineering Research Council of Canada (NSERC, www.nserc.ca) to D. Krewski, who holds the NSERC Chair in Risk Science at the University of Ottawa. N. Farhat, D. Krewski, N. Karyakina, D. Mattison, F. Momoli, S. Ramoju and N. Shilnikova were compensated by RSI for their contributions to the review. N. Farhat was also supported in part by postdoctoral fellowships from the McLaughlin Centre for Population Health Risk Assessment at the University of Ottawa and from Carleton University under the Mitacs Accelerate program (www.mitacs.ca), a peer-reviewed university-industry partnership program with RSI as the industrial partner. B. Cline contributed to this article as a senior scientist at IMnI. The authors, whose affiliations are shown on the title page, had sole responsibility for preparation of this paper, including determining the strategy for reviewing the scientific literature summarized in this article, synthesizing the findings, and drawing conclusions. Although Dr. Cline briefed the IMnI Health, Safety and Environment Committee on the results the present review, the Committee did not provide input to this work. None of the authors have appeared before regulatory agencies on behalf of the sponsors or appeared as experts in legal proceedings concerning matters reviewed in this paper. The scientific opinions and conclusions expressed in the article are exclusively those of the authors and are independent of the sources of financial support.
Supplemental material
Supplemental material for this article is available online here at https://doi.org/10.1080/10408444.2022.2128718
Notes
1 The focus of Ellingsen et al. 2007 was on possible biomarkers of the effects of Mn on the male reproductive function. This study is not summarized in this section.
References
- ACGIH. 2012. Manganese, elemental and inorganic compounds: TLV chemical substances draft documentation, notice of intended change. Documentation of the threshold limit values and biological exposure indices. Cincinnati: American Conference of Governmental Industrial Hygienists.
- Andersen ME, Gearhart JM, Clewell HJ. 3rd. 1999. Pharmacokinetic data needs to support risk assessments for inhaled and ingested manganese. Neurotoxicology. 20(2–3):161–171.
- Apostoli P, Lucchini R, Alessio L. 2000. Are current biomarkers suitable for the assessment of manganese exposure in individual workers? Am J Ind Med. 37(3):283–290.
- Aschner M, Erikson KM, Herrero Hernández E, Hernández EH, Tjalkens R. 2009. Manganese and its role in Parkinson’s disease: from transport to neuropathology. Neuromolecular Med. 11(4):252–266.
- Aschner M, Vrana KE, Zheng W. 1999. Manganese uptake and distribution in the central nervous system (CNS). Neurotoxicology. 20(2–3):173–180.
- ATSDR. 2012. Toxicological profile for manganese. Atlanta (GA): Agency for Toxic Substances and Disease Registry.
- Bader M, Dietz MC, Ihrig A, Triebig G. 1999. Biomonitoring of manganese in blood, urine and axillary hair following low-dose exposure during the manufacture of dry cell batteries. Int Arch Occup Environ Health. 72(8):521–527.
- Bailey LA, Kerper LE, Goodman JE. 2018. Derivation of an occupational exposure level for manganese in welding fumes. Neurotoxicology. 64:166–176.
- Baker MG, Simpson CD, Stover B, Sheppard L, Checkoway H, Racette BA, Seixas NS. 2014. Blood manganese as an exposure biomarker: state of the evidence. J Occup Environ Hyg. 11(4):210–217.
- Baker MG, Stover B, Simpson CD, Sheppard L, Seixas NS. 2016. Using exposure windows to explore an elusive biomarker: blood manganese. Int Arch Occup Environ Health. 89(4):679–687.
- Baldwin M, Belanger S, Larribe F, Mergler D. 1993. Estime cumulatif de l’exposition anterieure au manganese dans une usine d’alliages de ferro- et de silicomangane. 15th Conference of the Industrial Hygiene Association of Quebec.
- Baldwin M, Bouchard M, Larribe F, Mergler D. 2008. Past occupational exposure to airborne manganese in a manganese alloy plant. J Occup Environ Hyg. 5(7):426–437.
- Baldwin M, Mergler D, Larribe F, Belanger S, Tardif R, Bilodeau L, Hudnell K. 1999. Bioindicator and exposure data for a population based study of manganese. Neurotoxicology. 20(2–3):343–353.
- Barrington WW, Angle CR, Willcockson NK, Padula MA, Korn T. 1998. Autonomic function in manganese alloy workers. Environ Res. 78(1):50–58.
- Bast-Pettersen R, Ellingsen DG, Hetland SM, Thomassen Y. 2004. Neuropsychological function in manganese alloy plant workers. Int Arch Occup Environ Health. 77(4):277–287.
- Beuter A, Lambert G, MacGibbon B. 2004. Quantifying postural tremor in workers exposed to low levels of manganese. J Neurosci Methods. 139(2):247–255.
- Blond M, Netterstrom B. 2007. Neuromotor function in a cohort of Danish steel workers. Neurotoxicology. 28(2):336–344.
- Blond M, Netterstrom B, Laursen P. 2007. Cognitive function in a cohort of Danish steel workers. Neurotoxicology. 28(2):328–335.
- Bonberg N, Pesch B, Ulrich N, Moebus S, Eisele L, Marr A, Arendt M, Jockel KH, Bruning T, Weiss T. 2017. The distribution of blood concentrations of lead (Pb), cadmium (Cd), chromium (Cr) and manganese (Mn) in residents of the German Ruhr area and its potential association with occupational exposure in metal industry and/or other risk factors. Int J Hyg Environ Health. 220(6):998–1005.
- Boojar MM, Goodarzi F, Basedaghat MA. 2002. Long-term follow-up of workplace and well water manganese effects on iron status indexes in manganese miners. Arch Environ Health. 57(6):519–528.
- Bouchard M, Mergler D, Baldwin M, Panisset M, Bowler R, Roels HA. 2007. Neurobehavioral functioning after cessation of manganese exposure: a follow-up after 14 years. Am J Ind Med. 50(11):831–840.
- Bouchard M, Mergler D, Baldwin M, Panisset M, Roels HA. 2007. Neuropsychiatric symptoms and past manganese exposure in a ferro-alloy plant. Neurotoxicology. 28(2):290–297.
- Bouchard M, Mergler D, Baldwin M, Sassine MP, Bowler R, MacGibbon B. 2003. Blood manganese and alcohol consumption interact on mood states among manganese alloy production workers. Neurotoxicology. 24(4–5):641–647.
- Bowler RM, Nakagawa S, Drezgic M, Roels HA, Park RM, Diamond E, Mergler D, Bouchard M, Bowler RP, Koller W. 2007. Sequelae of fume exposure in confined space welding: a neurological and neuropsychological case series. Neurotoxicology. 28(2):298–311.
- Bowler RM, Roels HA, Nakagawa S, Drezgic M, Diamond E, Park R, Koller W, Bowler RP, Mergler D, Bouchard M, et al. 2007. Dose-effect relationships between manganese exposure and neurological, neuropsychological and pulmonary function in confined space bridge welders. Occup Environ Med. 64(3):167–177.
- Brown DSO, Wills CE, Yousefi V, Nell V. 1991. Neurotoxic effects of chronic exposure to manganese dust. Neuropsychiatry Neuropsychol Behav Neurol. 4(3):238–250.
- Buchet JP, Magos C, Roels H, Ceulemans E, Lauwerys R. 1993. Urinary excretion of homovanillic acid in workers exposed to manganese. Int Arch Occup Environ Health. 65(2):131–133.
- Calne DB, Chu NS, Huang CC, Lu CS, Olanow W. 1994. Manganism and idiopathic Parkinsonism: similarities and differences. Neurology. 44(9):1583–1586.
- Casjens S, Dydak U, Dharmadhikari S, Lotz A, Lehnert M, Quetscher C, Stewig C, Glaubitz B, Schmidt-Wilcke T, Edmondson D, et al. 2018. Association of exposure to manganese and iron with striatal and thalamic GABA and other neurometabolites – neuroimaging results from the WELDOX II study. Neurotoxicology. 64:60–67.
- Casjens S, Pesch B, Robens S, Kendzia B, Behrens T, Weiss T, Ulrich N, Arendt M, Eisele L, Pundt N, et al. 2017. Associations between former exposure to manganese and olfaction in an elderly population: results from the Heinz Nixdorf Recall Study. Neurotoxicology. 58:58–65.
- Cavallari JM. 2010. Manganese exposure and cardiovascular responses among welders. CPWR (The Center for Construction Research and Training).
- Chandra SV, Shukla GS, Srivastava RS, Singh H, Gupta VP. 1981. An exploratory study of manganese exposure to welders. Clin Toxicol. 18(4):407–416.
- Chia SE, Phoon WH, Lee HS, Tan KT, Jeyaratnam J. 1993. Exposure to neurotoxic metals among workers in Singapore: an overview. Occup Med (Lond). 43(1):18–22.
- Coelho P, Costa S, Costa C, Silva S, Walter A, Ranville J, Pastorinho MR, Harrington C, Taylor A, Dall’Armi V, et al. 2014. Biomonitoring of several toxic metal(loid)s in different biological matrices from environmentally and occupationally exposed populations from Panasqueira mine area, Portugal. Environ Geochem Health. 36(2):255–269.
- Cowan DM, Fan Q, Zou Y, Shi X, Chen J, Aschner M, Rosenthal FS, Zheng W. 2009. Manganese exposure among smelting workers: blood manganese-iron ratio as a novel tool for manganese exposure assessment. Biomarkers. 14(1):3–16.
- Cowan DM, Zheng W, Zou Y, Shi X, Chen J, Rosenthal FS, Fan Q. 2009. Manganese exposure among smelting workers: relationship between blood manganese-iron ratio and early onset neurobehavioral alterations. Neurotoxicology. 30(6):1214–1222.
- Dietz MC, Ihrig A, Wrazidlo W, Bader M, Jansen O, Triebig G. 2001. Results of magnetic resonance imaging in long-term manganese dioxide-exposed workers. Environ Res. 85(1):37–40.
- Dorman DC, Struve MF, James RA, Marshall MW, Parkinson CU, Wong BA. 2001. Influence of particle solubility on the delivery of inhaled manganese to the rat brain: manganese sulfate and manganese tetroxide pharmacokinetics following repeated (14-day) exposure. Toxicol Appl Pharmacol. 170(2):79–87.
- Drown DB, Oberg SG, Sharma RP. 1986. Pulmonary clearance of soluble and insoluble forms of manganese. J Toxicol Environ Health. 17(2–3):201–212.
- Ellingsen DG, Chashchin M, Berlinger B, Konz T, Zibarev E, Aaseth J, Chashchin V, Thomassen Y. 2014. Biomarkers of iron status and trace elements in welders. J Trace Elem Med Biol. 28(3):271–277.
- Ellingsen DG, Chashchin V, Haug E, Chashchin M, Tkachenko V, Lubnina N, Bast-Pettersen R, Thomassen Y. 2007. An epidemiological study of reproductive function biomarkers in male welders. Biomarkers. 12(5):497–509.
- Ellingsen DG, Dubeikovskaya L, Dahl K, Chashchin M, Chashchin V, Zibarev E, Thomassen Y. 2006. Air exposure assessment and biological monitoring of manganese and other major welding fume components in welders. J Environ Monit. 8(10):1078–1086.
- Ellingsen DG, Haug E, Ulvik RJ, Thomassen Y. 2003. Iron status in manganese alloy production workers. J Appl Toxicol. 23(4):239–247.
- Ellingsen DG, Hetland SM, Thomassen Y. 2003. Manganese air exposure assessment and biological monitoring in the manganese alloy production industry. J Environ Monitor. 5(1):84–90.
- Ellingsen DG, Konstantinov R, Bast-Pettersen R, Merkurjeva L, Chashchin M, Thomassen Y, Chashchin V. 2008. A neurobehavioral study of current and former welders exposed to manganese. Neurotoxicology. 29(1):48–59.
- Ellingsen DG, Kusraeva Z, Bast-Pettersen R, Zibarev E, Chashchin M, Thomassen Y, Chashchin V. 2014. The interaction between manganese exposure and alcohol on neurobehavioral outcomes in welders. Neurotoxicol Teratol. 41:8–15.
- Ellingsen DG, Shvartsman G, Bast-Pettersen R, Chashchin M, Thomassen Y, Chashchin V. 2019. Neurobehavioral performance of patients diagnosed with manganism and idiopathic Parkinson disease. Int Arch Occup Environ Health. 92(3):383–394.
- Ellingsen DG, Zibarev E, Kusraeva Z, Berlinger B, Chashchin M, Bast-Pettersen R, Chashchin V, Thomassen Y. 2013. The bioavailability of manganese in welders in relation to its solubility in welding fumes. Environ Sci Proc Impacts. 15(2):357–365.
- Emara AM, El Ghawabi SH, Madkour OI, El Samra GH. 1971. Chronic manganese poisoning in the dry buttery industry. Br J Ind Med. 28(1):78–82.
- Farrell PJ, Aggett P, Milton B, Ramoju S, Mattison D, Birkett N, Krewski D. 2022. The use of categorical regression in evidence integration. ALTEX.
- Finley BL, Santamaria AB. 2005. Current evidence and research needs regarding the risk of manganese-induced neurological effects in welders. Neurotoxicology. 26(2):285–289.
- Freeland-Graves JH, Behmardi F, Bales CW, Dougherty V, Lin PH, Crosby JB, Trickett PC. 1988. Metabolic balance of manganese in young men consuming diets containing five levels of dietary manganese. J Nutr. 118(6):764–773.
- Gan SL, Tan KT, Kwok SF. 1988. Biological threshold limit values for manganese dust exposure. Singapore Med J. 29(2):105–109.
- Grashow R, Zhang J, Fang SC, Weisskopf MG, Christiani DC, Cavallari JM. 2014. Toenail metal concentration as a biomarker of occupational welding fume exposure. J Occup Environ Hyg. 11(6):397–405.
- Gutiérrez-González E, García-Esquinas E, de Larrea-Baz NF, Salcedo-Bellido I, Navas-Acien A, Lope V, Gómez-Ariza JL, Pastor R, Pollán M, Pérez-Gómez B. 2019. Toenails as biomarker of exposure to essential trace metals: a review. Environ Res. 179(Pt A):108787.
- Hałatek T, Sinczuk-Walczak H, Szymczak M, Rydzynski K. 2005. Neurological and respiratory symptoms in shipyard welders exposed to manganese. Int J Occup Med Environ Health. 18(3):265–274.
- Hammer SE, Ervik T, Ellingsen DG, Thomassen Y, Weinbruch S, Benker N, Berlinger B. 2021. Particle characterisation and bioaccessibility of manganese in particulate matter in silico- and ferromanganese smelters. Environ Sci Proc Impacts. 23(10):1488–1499.
- Hassani H, Golbabaei F, Shirkhanloo H, Tehrani-Doust M. 2016. Relations of biomarkers of manganese exposure and neuropsychological effects among welders and ferroalloy smelters. Industrial Health. 54(1):79–86.
- Hoet P, Roels HA. 2015. Chapter 14, Significance and usefulness of biomarkers of exposure to manganese. In Costa LG, Aschner M, editors. Manganese in health and disease. London: The Royal Society of Chemistry; p. 355–401.
- Hoet P, Vanmarcke E, Geens T, Deumer G, Haufroid V, Roels HA. 2012. Manganese in plasma: a promising biomarker of exposure to Mn in welders. A pilot study. Toxicol Lett. 213(1):69–74.
- Hua MS, Huang CC. 1991. Chronic occupational exposure to manganese and neurobehavioral function. J Clin Exp Neuropsychol. 13(4):495–507.
- Huang CC, Chu NS, Lu CS, Chen RS, Calne DB. 1998. Long-term progression in chronic manganism: ten years of follow-up. Neurology. 50(3):698–700.
- IRSST. 2005. Management of occupational management of occupational manganism consensus of an experts’ panel. Report R 417. Canada: Institut de Recherche Robert Sauvé en Santé et en Sécurité du Travail.
- Jankovic J. 2005. Searching for a relationship between manganese and welding and Parkinson’s disease. Neurology. 64(12):2021–2028.
- Jarvisalo J, Olkinuora M, Kiilunen M, Kivisto H, Ristola P, Tossavainen A, Aitio A. 1992. Urinary and blood manganese in occupationally nonexposed populations and in manual metal arc welders of mild steel. Int Arch Occup Environ Health. 63(7):495–501.
- Jiang Y, Zheng W, Long L, Zhao W, Li X, Mo X, Lu J, Fu X, Li W, Liu S, et al. 2007. Brain magnetic resonance imaging and manganese concentrations in red blood cells of smelting workers: search for biomarkers of manganese exposure. Neurotoxicology. 28(1):126–135.
- Kim E, Kim Y, Cheong HK, Cho S, Shin YC, Sakong J, Kim KS, Yang JS, Jin YW, Kang SK. 2005. Pallidal index on MRI as a target organ dose of manganese: structural equation model analysis. Neurotoxicology. 26(3):351–359.
- Kim EA, Cheong HK, Choi DS, Sakong J, Ryoo JW, Park I, Kang DM. 2007. Effect of occupational manganese exposure on the central nervous system of welders: 1H magnetic resonance spectroscopy and MRI findings [evaluation studies]. Neurotoxicology. 28(2):276–283.
- Kim Y, Kim KS, Yang JS, Park IJ, Kim E, Jin Y, Kwon KR, Chang KH, Kim JW, Park SH, et al. 1999. Increase in signal intensities on T1-weighted magnetic resonance images in asymptomatic manganese-exposed workers. Neurotoxicology. 20(6):901–907.
- Ky SQ, Deng HS, Xie PY, Hu W. 1992. A report of two cases of chronic serious manganese poisoning treated with sodium para-aminosalicylic acid. Br J Ind Med. 49(1):66–69.
- Laohaudomchok W, Lin X, Herrick RF, Fang SC, Cavallari JM, Christiani DC, Weisskopf MG. 2011. Toenail, blood, and urine as biomarkers of manganese exposure. J Occup Environ Med. 53(5):506–510.
- Li GJ, Zhang LL, Lu L, Wu P, Zheng W. 2004. Occupational exposure to welding fume among welders: alterations of manganese, iron, zinc, copper, and lead in body fluids and the oxidative stress status. J Occup Environ Med. 46(3):241–248.
- Long Z, Jiang YM, Li XR, Fadel W, Xu J, Yeh CL, Long LL, Luo HL, Harezlak J, Murdoch JB, et al. 2014. Vulnerability of welders to manganese exposure–a neuroimaging study. Neurotoxicology. 45:285–292.
- Lu L, Zhang LL, Li GJ, Guo W, Liang W, Zheng W. 2005. Alteration of serum concentrations of manganese, iron, ferritin, and transferrin receptor following exposure to welding fumes among career welders. Neurotoxicology. 26(2):257–265.
- Lucchini R, Apostoli P, Perrone C, Placidi D, Albini E, Migliorati P, Mergler D, Sassine MP, Palmi S, Alessio L. 1999. Long-term exposure to “low levels” of manganese oxides and neurofunctional changes in ferroalloy workers. Neurotoxicology. 20(2–3):287–297.
- Lucchini R, Bergamaschi E, Smargiassi A, Festa D, Apostoli P. 1997. Motor function, olfactory threshold, and hematological indices in manganese-exposed ferroalloy workers. Environ Res. 73(–-2):175–180.
- Lucchini R, Selis L, Folli D, Apostoli P, Mutti A, Vanoni O, Iregren A, Alessio L. 1995. Neurobehavioral effects of manganese in workers from a ferroalloy plant after temporary cessation of exposure. Scand J Work Environ Health. 21(2):143–149.
- Mattison DR, Milton B, Krewski D, Levy L, Dorman DC, Aggett PJ, Roels HA, Andersen ME, Karyakina NA, Shilnikova N, et al. 2017. Severity scoring of manganese health effects for categorical regression. Neurotoxicology. 58:203–216.
- McClellan RO. 2020. Health effects of nuclear weapons and releases of radioactive materials. In Gupta RC, editor. Handbook of toxicology of chemical warfare agents. Amsterdam, the Netherlands: Elsevier Publishing; p. 707–743.
- Mehrifar Y, Bahrami M, Sidabadi E, Pirami H. 2020. The effects of occupational exposure to manganese fume on neurobehavioral and neurocognitive functions: An analytical cross-sectional study among welders. Excli J. 19:372–386.
- Mercier MJ, Robinson AE. 1993. Use of biologic markers for toxic end-points in assessment of risks from exposure to chemicals. Int Arch Occup Environ Health. 65(1 Suppl):S7–S10.
- Mergler D, Baldwin M. 1997. Early manifestations of manganese neurotoxicity in humans: an update. Environ Res. 73(1–2):92–100.
- Mergler D, Huel G, Bowler R, Iregren A, Bélanger S, Baldwin M, Tardif R, Smargiassi A, Martin L. 1994. Nervous system dysfunction among workers with long-term exposure to manganese. Environ Res. 64(2):151–180.
- Meyer-Baron M, Knapp G, Schaper M, van Thriel C. 2009. Performance alterations associated with occupational exposure to manganese–a meta-analysis. Neurotoxicology. 30(4):487–496.
- Milne DB, Sims RL, Ralston NV. 1990. Manganese content of the cellular components of blood. Clin Chem. 36(3):450–452.
- Mirmohammadi S, Moghaddasi Y, Yazdani J, Yousefinejad R, Esfandyari Y, Gorgani M, Habibpour M. 2017. Correlation between airborne manganese concentration at the workstations in the iron foundry and manganese concentration in workers’ blood. Med Pr. 68(4):449–458.
- Moher D, Liberati A, Tetzlaff J, Altman DG, PRISMA Group. 2009. Preferred reporting items for systematic reviews and meta-analyses: the PRISMA statement. PLoS Med. 6(7):e1000097.
- Myers JE, Fine J, Ormond-Brown D, Fry J, Thomson A, Thompson ML. 2009. Estimating the prevalence of clinical manganism using a cascaded screening process in a South African manganese smelter. Neurotoxicology. 30(6):934–940.
- Myers JE, Thompson ML, Naik I, Theodorou P, Esswein E, Tassell H, Daya A, Renton K, Spies A, Paicker J, et al. 2003. The utility of biological monitoring for manganese in ferroalloy smelter workers in South Africa. Neurotoxicology. 24(6):875–883.
- Myers JE, Thompson ML, Ramushu S, Young T, Jeebhay MF, London L, Esswein E, Renton K, Spies A, Boulle A, et al. 2003. The nervous system effects of occupational exposure on workers in a South African Manganese Smelter. Neurotoxicology. 24(6):885–894.
- Oberdoerster G, Cherian G, et al. 1988. Manganese. In Clarkson T, Friberg L, Nordberg G, editors. Biological monitoring of toxic metals. New York: Plenum Press; p. 283–301.
- Park RM, Baldwin M, Bouchard MF, Mergler D. 2014. Airborne manganese as dust vs. fume determining blood levels in workers at a manganese alloy production plant. Neurotoxicology. 45:267–275.
- Park RM, Bouchard MF, Baldwin M, Bowler R, Mergler D. 2014. Respiratory manganese particle size, time-course and neurobehavioral outcomes in workers at a manganese alloy production plant. Neurotoxicology. 45:276–284.
- Pesch B, Weiss T, Kendzia B, Henry J, Lehnert M, Lotz A, Heinze E, Kafferlein HU, Van Gelder R, Berges M, et al. 2012. Levels and predictors of airborne and internal exposure to manganese and iron among welders. J Expo Sci Environ Epidemiol. 22(3):291–298.
- Ramoju SP, Mattison DR, Milton B, McGough D, Shilnikova N, Clewell HJ, Yoon M, Taylor MD, Krewski D, Andersen ME. 2017. The application of PBPK models in estimating human brain tissue manganese concentrations. Neurotoxicology. 58:226–237.
- Rodier J. 1955. Manganese poisoning in Moroccan miners. Br J Ind Med. 12(1):21–35.
- Roels H, Lauwerys R, Buchet JP, Genet P, Sarhan MJ, Hanotiau I, de Fays M, Bernard A, Stanescu D. 1987. Epidemiological survey among workers exposed to manganese: effects on lung, central nervous system, and some biological indices. Am J Ind Med. 11(3):307–327.
- Roels H, Lauwerys R, Genet P, Sarhan MJ, de Fays M, Hanotiau I, Buchet JP. 1987. Relationship between external and internal parameters of exposure to manganese in workers from a manganese oxide and salt producing plant. Am J Ind Med. 11(3):297–305.
- Roels H, Meiers G, Delos M, Ortega I, Lauwerys R, Buchet JP, Lison D. 1997. Influence of the route of administration and the chemical form (MnCl2, MnO2) on the absorption and cerebral distribution of manganese in rats. Arch Toxicol. 71(4):223–230.
- Roels HA, Ghyselen P, Buchet JP, Ceulemans E, Lauwerys RR. 1992. Assessment of the permissible exposure level to manganese in workers exposed to manganese dioxide dust. Br J Ind Med. 49(1):25–34.
- Rolle-McFarland D, Liu Y, Mostafaei F, Zauber SE, Zhou Y, Li Y, Fan Q, Zheng W, Nie LH, Wells EM. 2019. The association of bone, fingernail and blood manganese with cognitive and olfactory function in Chinese workers. Sci Total Environ. 666:1003–1010.
- Rolle-McFarland D, Liu Y, Zhou J, Mostafaei F, Zhou Y, Li Y, Fan Q, Zheng W, Nie LH, Wells EM. 2018. Development of a Cumulative Exposure Index (CEI) for manganese and comparison with bone manganese and other biomarkers of manganese exposure. Int J Environ Res Public Health. 15(7):1341.
- Shilnikova N, Karyakina N, Farhat N, Ramoju S, Cline B, Momoli F, Mattison D, Jensen N, Terrell R, Krewski D. 2022. Biomarkers of environmental manganese exposure. Crit Rev Toxicol. 52:325–343.
- Sjogren B, Iregren A, Frech W, Hagman M, Johansson L, Tesarz M, Wennberg A. 1996. Effects on the nervous system among welders exposed to aluminium and manganese. Occup Environ Med. 53(1):32–40.
- Smargiassi A, Mergler D, Bergamaschi E, Vetton MV, Lucchini R, Apostoli P. 1995. Peripheral markers of catecholamine metabolism among workers occupationally exposed to manganese (Mn). Toxicol Lett. 77(1–3):329–333.
- Smith D, Gwiazda R, Bowler R, Roels H, Park R, Taicher C, Lucchini R. 2007. Biomarkers of Mn exposure in humans [Research Support, N.I.H., Extramural]. Am J Ind Med. 50(11):801–811.
- Smyth LT, Ruhf RC, Whitman NE, Dugan T. 1973. Clinical manganism and exposure to manganese in the production and processing of ferromanganese alloy. J Occup Med. 15(2):101–109.
- Stanislawska M, Janasik B, Kuras R, Malachowska B, Halatek T, Wasowicz W. 2020. Assessment of occupational exposure to stainless steel welding fumes – A human biomonitoring study. Toxicol Lett. 329:47–55.
- Suzuki Y, Fujii N, Yano H, Ohkita T, Ichikawa A, Nishiyama K. 1978. Effect of the inhalation of manganese dioxide dust on monkey lung. Tokushima J Exp Med. 25:119–125.
- US EPA. 1984. Health assessment document for manganese. Final draft. Cincinnati (OH): US Environmental Protection Agency, Office of Research and Development. [accessed 2 Nov 2020]. https://nepis.epa.gov/Exe/ZyPDF.cgi/30001APG.PDF?Dockey=30001APG.PDF
- Verity MA. 1999. Manganese neurotoxicity: a mechanistic hypothesis. Neurotoxicology. 20(2–3):489–497.
- Vinnikov D, Semizhon S, Rybina T, Zaitsev V, Pleshkova A, Rybina A. 2018. Occupational exposure to metals and other elements in the tractor production. PLoS One. 13(12):e0208932.
- Wang D, Du X, Zheng W. 2008. Alteration of saliva and serum concentrations of manganese, copper, zinc, cadmium and lead among career welders. Toxicol Lett. 176(1):40–47.
- Ward EJ, Edmondson DA, Nour MM, Snyder S, Rosenthal FS, Dydak U. 2018. Toenail manganese: a sensitive and specific biomarker of exposure to manganese in career welders. Ann Work Exposures Health. 62(1):101–111.
- WHO 1981. Manganese. Geneva, Switzeland: World Health Organization.
- Wongwit W, Kaewkungwal J, Chantachum Y, Visesmanee V. 2004. Comparison of biological specimens for manganese determination among highly exposed welders. Southeast Asian J Trop Med Public Health. 35(3):764–769.
- Young T, Myers JE, Thompson ML. 2005. The nervous system effects of occupational exposure to manganese – measured as respirable dust – in a South African manganese smelter. Neurotoxicology. 26(6):993–1000.
- Yuan H, He S, He M, Niu Q, Wang L, Wang S. 2006. A comprehensive study on neurobehavior, neurotransmitters and lymphocyte subsets alteration of Chinese manganese welding workers. Life Sci. 78(12):1324–1328.
- Zeise L, Bois FY, Chiu WA, Hattis D, Rusyn I, Guyton KZ. 2013. Addressing human variability in next-generation human health risk assessments of environmental chemicals. Environ Health Perspect. 121(1):23–31.