Abstract
Despite the growing epidemiological evidence of an association between toxin exposure and developmental neurotoxicity (DNT), systematic testing of DNT is not mandatory in international regulations for admission of pharmaceuticals or industrial chemicals. However, to date around 200 compounds, ranging from pesticides, pharmaceuticals and industrial chemicals, have been tested for DNT in the current OECD test guidelines (TG-443 or TG-426). There are calls for the development of new approach methodologies (NAMs) for DNT, which has resulted in a DNT testing battery using in vitro human cell-based assays. These assays provide a means to elucidate the molecular mechanisms of toxicity in humans which is lacking in animal-based toxicity tests. However, cell-based assays do not represent all steps of the complex process leading to DNT. Validated models with a multi-organ network of pathways that interact at the molecular, cellular and tissue level at very specific timepoints in a life cycle are currently missing. Consequently, whole model organisms are being developed to screen for, and causally link, new molecular targets of DNT compounds and how they affect whole brain development and neurobehavioral endpoints. Given the practical and ethical restraints associated with vertebrate testing, lower animal models that qualify as 3 R (reduce, refine and replace) models, including the nematode (Caenorhabditis elegans) and the zebrafish (Danio rerio) will prove particularly valuable for unravelling toxicity pathways leading to DNT. Although not as complex as the human brain, these 3 R-models develop a complete functioning brain with numerous neurodevelopmental processes overlapping with human brain development. Importantly, the main signalling pathways relating to (neuro)development, metabolism and growth are highly conserved in these models. We propose the use of whole model organisms specifically zebrafish and C. elegans for DNT relevant endpoints.
1. Introduction
In humans, the development of the central nervous system is extremely complex, involving many different processes at the molecular, cellular and tissue level. Embryonic and foetal development of the central nervous system occurs in strictly controlled timeframes, involving a complex interplay between multiple developmental processes that occur both pre- and postnatally (Grandjean and Landrigan Citation2014; Heyer and Meredith Citation2017) (). These processes include neurogenesis, migration, axon guidance, synaptogenesis, myelination, phenotypic specification of neurons (e.g. glutamatergic or dopaminergic neurons), expression and maturation of receptors and ion channels to facilitate synaptogenesis, axon guidance, neuronal network formation and brain segmentation (Smirnova et al. Citation2014; Bal-price et al. Citation2018; Hessel et al. Citation2018). Perturbation of these neurodevelopmental processes in developing humans may result in neurodevelopmental disorders (NDD) which affect 10–15% of all births (Bourgeron Citation2015; Astle et al. Citation2021), and are increasing worldwide (Landrigan et al. Citation2012). Examples of NDD include intellectual disability (ID), Communication Disorders, Autism Spectrum Disorder (ASD), Attention-Deficit/Hyperactivity Disorder (ADHD), Specific Learning Disorders and Motor Disorders (American Psychiatric Association Citation2013). The exact mechanisms and aetiologies of NDD are generally unknown, but exposure to environmental toxic compounds is a contributing factor (Grandjean and Landrigan Citation2014; Abreu-Villaça and Levin Citation2017; Jedynak et al. Citation2021; Julvez et al. Citation2021). While various epidemiology and exposome studies show associations between NDD and chemical exposure, toxicity studies are required to demonstrate causality between chemical exposure and the development of NDD. This should be done in the area of developmental neurotoxicity (DNT) (De Felice et al. Citation2015; Heyer and Meredith Citation2017; Bellinger Citation2018; Cheroni et al. Citation2020; Nannaware et al. Citation2024).
Figure 1. The cellular processes involved in brain development. The key biological processes in human brain development are shown, with their approximate time span. The majority of processes occur in utero, with some of the more complex processes, such as myelination, synaptogenesis and neuronal specification, occurring at later stages of gestation and during early childhood. Specifically, the blood brain barrier continues to form until the early teenage years. The processes which can be modelled in cell culture (green stars), Caenorhabditis elegans (purple stars) and zebrafish, Danio rerio (orange stars) are indicated where these processes are present and can be measured. This information is based on literature (Keeney et al. Citation2015; Hessel et al. Citation2018; Sun and Hobert Citation2023) and generated with BioRender.com.
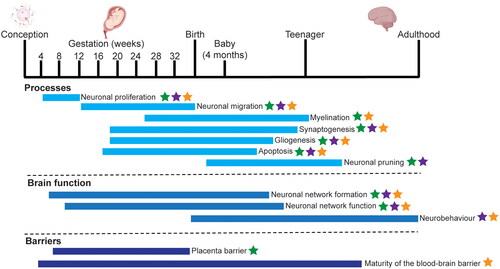
In humans, up until the third trimester, the blood–brain barrier is immature (Haddad-Tóvolli et al. Citation2017) and has a reduced ability to detoxify exogenous compounds, consequently the developing nervous system is more vulnerable to the neurotoxic effects of compounds than the adult nervous system (Rice and Barone Citation2000; Smirnova et al. Citation2014). While various epidemiology and exposome studies show associations between NDD and compound exposure, toxicity studies are urgently needed to demonstrate causality between toxic compound exposure and the development of NDD. This should be done within the area of developmental neurotoxicity (DNT). Although neurodegenerative disorders that occur later in life, such as Parkinson’s or Alzheimer’s Disease may be caused by chemical exposure (Yan et al. Citation2016; Shan et al. Citation2023), the focus of this review is on neurodevelopmental disorders, and not on neurodegeneration.
Systematic testing of compounds for DNT is not mandatory in any of the chemical regulatory sectors (Sachana, Shafer, et al. Citation2021). The current DNT test guidelines from the OECD have only been used on a limited number of chemicals and are tested for regulatory purposes with the OECD-TG-426 (OECD Citation2007, Citation2018a) or the DNT cohort of the OECD-TG-443 (OECD Citation2018b). (Makris et al. Citation2009; Aschner et al. Citation2017; Crofton and Mundy Citation2021; Sachana, Shafer, et al. Citation2021; Martin et al. Citation2022). Such limited use of the OECD-TG is the result of several factors, namely that testing is expensive, time- and resource-consuming, as well as requiring a large number of animals.
Together this makes animal tests unsuited to screen large numbers of compounds. Furthermore, the predictivity of these tests for human DNT-related health effects is uncertain given species differences on brain development and functioning (Fritsche, Barenys, et al. Citation2018; Sheets and Slikker Citation2018). This limited testing, coupled with an increasing need to assess the hazards of compounds, including environmental chemicals, industrial contaminants, pharmaceuticals, pesticides and xenobiotics, has resulted in calls for the development and use of new approach methodologies (NAMs) for DNT based on knowledge from human biology and physiology (Hessel et al. Citation2018; Desprez et al. Citation2019; Schmeisser et al. Citation2023). Other information can be required from epidemiological findings. For example, lead (Lidsky and Schneider Citation2003) and methyl mercury (Antunes Dos Santos et al. Citation2016) are known developmental neurotoxicants and thus the advice during pregnancy is to limit exposure to these chemicals. Recent concerns from epidemiology studies have been raised for potential developmental neurotoxicants, including bisphenols and PFAS (Per- and polyfluoroalkyl substances) (Oh et al. Citation2022; Carstens et al. Citation2023). PFAS are persistent chemicals that were already present in high concentrations in the environment, as well as in humans, before DNT effects were suspected. This highlights the urgency to identify the DNT-potential of compounds prior to high production volumes, as well as the need for retrospective testing for the DNT potential of currently produced compounds.
The OECD has recently developed an in vitro battery for testing how compounds impact key processes in brain development, the DNT-IVB. This DNT-IVB includes, mostly, human-based cell models that measure effects of toxic compounds on key cellular neuronal processes such as proliferation, migration, network formation (Masjosthusmann et al. Citation2020) (). While the DNT-IVB is valuable for the regulatory assessment of potential DNT compounds, it has some limitations, as firstly the assays do not cover all neurodevelopmental processes. For example, while there are a number of in vitro tests for early neurodevelopmental processes and key events (i.e. proliferation, differentiation, myelinisation, growth apoptosis or migration, see ) (Bal-price et al. Citation2018; Fritsche, Grandjean, et al. Citation2018; Hessel et al. Citation2018), there is a lack of available tests, in any form, for later processes or key events such as synaptogenesis, network function or neurobehavior. Testing the effects of compounds on functional endpoints such as network functioning or neurobehavior will be important to ensure that the function of the brain is not affected by compound exposure. Secondly, while cell-based assays are used to model and evaluate key neurodevelopmental processes and how these processes are affected by compounds (Li J et al. Citation2019; Sachana, Willett, et al. Citation2021), they also have limitations in terms of their metabolic capacity. Third, whole brain development and behavioural effects cannot be evaluated in a cell-based system (Li J et al. Citation2019). Further, behavioural and cognitive effects cannot be evaluated in a cell-based system. This is particularly striking as perturbation of DNT processes that result in neurodevelopmental disorders that occur at a very young age therefore have a high societal impact (Thapar et al. Citation2017). Therefore, in addition to the cell-based assays, non-mammalian small model organism models can be employed where the nervous system has a high level of evolutionary conservation with mammals. In this review, we will demonstrate the added value of nematode and zebrafish models for DNT testing. We will review the neurodevelopmental processes that can be measured in zebrafish and nematode models which are crucial for proper brain development.
Table 1. Key events that are crucial to adverse outcomes in developmental neurotoxicity.
2. Literature review methodology
This review provides an overview of developmental neurotoxicity testing using nematode (Caenorhabditis elegans) and zebrafish (Danio rerio) as experimental models, demonstrating their added value to the DNT field. Literature searches were performed using PubMed, Embase.com (Elsevier) and Toxline (US EPA) to identify publications on developmental neurotoxicity testing in the zebrafish and in C. elegans. A combination of the following search terms was used in title or abstract covered the period of January 2013 until March 2024 including, but not limited to: Caenorhabditis elegans, nematode, Danio rerio, zebrafish, in vitro battery, adverse outcome pathways, key events, adverse outcomes, developmental neurotoxicity, neurotoxicity, neurodevelopmental disorders, synaptogenesis, cognition, memory, learning, locomotion, toxicity. If needed references within the selected papers were studied and used as references. Only English-language text was considered. All relevant primary research articles and reviewers were reviewed. In total, we include 196 texts in this review. Occasionally there was a requirement to access older articles i.e. pre-2013, however they were only included if they were applicable to the topic, for example the C. elegans lineage papers. The resulting citations were screened for relevance with regards to health effects focusing on neurodevelopmental disorders relevant for humans. The focus of this review is on the added benefit of whole organism models. It should be noted that there are many highly cited article that concern cell-based assays, (Aschner et al. Citation2017; Bal-Price et al. Citation2017; Bal-Price and Meek Citation2017; Bal-price et al. Citation2018; Fritsche, Barenys, et al. Citation2018; Masjosthusmann et al. Citation2020; Sachana, Shafer, et al. Citation2021; Sachana, Willett, et al. Citation2021) and thus the authors rely on the knowledge described in these papers. For the information concerning the list of key neurodevelopmental processes in , we rely on three recent papers: Li J et al. (Citation2019), Spinu et al. (Citation2019) and Masjosthusmann et al. Citation2020).
3. We need suitable alternative whole organism models, such as nematodes and zebrafish, to be able to better explore the effects of developmental neurotoxicants
To further develop NAM based risk assessment for DNT, more information is required that takes into account the complexity of the human brain, together with the different phases and barriers involved during development (). To achieve this, we propose to utilise whole organismal systems that qualify as 3 R (Replace, Reduce and Refine) models for DNT, namely the nematode worm Caenorhabditis elegans and the zebrafish Danio rerio. Both nematodes (in this review, “nematodes” specifically refers to C. elegans) and zebrafish are multicellular organisms, and thus more complex than cell culture assays, yet are more simple than rodent models. Importantly C. elegans is not considered to be an animal experiment and therefore is exempt from ethical restraints. Zebrafish is not considered as an animal experiment up to 120hpf by the EU Directive 2010/63/EU on the protection of animals used for scientific purposes (Directive 2010/63/EU Citation2010; Strähle et al. Citation2012). Both nematodes and zebrafish have several advantages over mammalian animal testing, including a short generation time and a high reproduction rate as well as being favourable for high throughput applications. Traditionally raised on solid media, C. elegans is regarded as an oral toxicity model, but like zebrafish can also be raised in liquid culture. In contract, zebrafish are considered an absorption model for compound administration, thus these two different modes of exposure provide added benefit for observing toxicity of compounds. The absorption, distribution, metabolism and excretion (ADME) of molecules in regard to small organisms is very important. The concentration of chemicals in the external environment may be different to the internal concentration, and this is dependent on the dose concentration, exposure duration and developmental as well as the chemical properties, i.e. the Kow, all of which must be taken into consideration stage (Dexter et al. Citation2012; Brox et al. Citation2016; Wittkowski et al. Citation2019; Weiner et al. Citation2024).
Importantly, brain development of nematodes and zebrafish have striking overlap with human brain development (de Esch et al. Citation2012; Kozol et al. Citation2016; Ruszkiewicz et al. Citation2018; Witvliet et al. Citation2021), for example concerning bilateral asymmetry (Taylor et al. Citation2010), anatomy (Witvliet et al. Citation2021; Haynes et al. Citation2022) and circuitry (Nagai et al. Citation2021). Although not as complex as the human brain, both models develop a complete functioning brain that results in measurable behaviour (Tilson Citation1993; Cronin et al. Citation2005; Husson et al. Citation2012; Legradi et al. Citation2015; Javer et al. Citation2018; Shen et al. Citation2020). The gene homology between these models and humans is high, with C. elegans having up to 80% homology (Lai et al. Citation2000; Hillier et al. Citation2005; Shaye and Greenwald Citation2011; Kim et al. Citation2018) and zebrafish displaying 70% homology to humans (Barbazuk et al. Citation2000; Postlethwait et al. Citation2000; Howe et al. Citation2013). Together, this enables the elucidation of the molecular pathways and mechanisms underpinning adverse events (Lai et al. Citation2000; Howe et al. Citation2013; Sandner et al. Citation2021). The advantage of these whole organism models, in comparison to cell models, is that they can be used to test later KEs or even simple AOs.
3.1. Caenorhabditis elegans as a model for DNT
The free-living and non-parasitic nematode worm Caenorhabditis elegans offers a simple example of animal biology (Corsi et al. Citation2015). The nematode has a short egg-to-egg cycle of just 3 days at 20 °C, at which point each worm will produce 250–300 genetically identical offspring. After the egg laying period, the worm lives for a further 3–4 weeks before death. C. elegans is biologically well-characterised, with significant conservation to mammals at the genetic (between 60 and 80%) and protein (83%) levels (Lai et al. Citation2000; Hillier et al. Citation2005; Kaletta and Hengartner Citation2006). During growth and development, there is striking conservation of the developmental steps between C. elegans and vertebrates including cell division, differentiation, migration and cell death (Sulston and Horvitz Citation1977). How each of the 302 neurons connect to each other, the neurotransmitters and synaptic connections as well as their role in behaviour have been mapped into a connectome (White et al. Citation1986; Cook et al. Citation2019, Citation2023). The 6393 neurotransmitter synapses in C. elegans are the same as those found in humans, namely cholinergic, GABAergic, glutamatergic, dopaminergic and serotonergic (White et al. Citation1986; Brownlee and Fairweather Citation1999), and despite not having adrenaline and with some differences in sodium dependent ion channels (Bargmann Citation1998; Ruszkiewicz et al. Citation2018), the translational relevance of C. elegans to humans for DNT is significant.
The neurons of the nematode are broadly organised into a bundle of nerves in the head and tail as well as a spinal-cord like ventral nerve cord (White et al. Citation1986). Wiring diagrams of the C. elegans brain have been published (Brittin et al. Citation2021; Witvliet et al. Citation2021) together with the connectome (Cook et al. Citation2019; Brittin et al. Citation2021; Witvliet et al. Citation2021) which demonstrate the compact yet highly interconnected neural system (Cook et al. Citation2023). The majority of neurodevelopmental processes occur during embryonic development, including neurogenesis, growth of dendrites and axons and neuronal migration (Sun and Hobert Citation2023). Synaptic addition and pruning is evident during early post-embryonic development and is maintained into later life with sexually dimorphic characteristics arising at later embryonic and post-embryonic stages (Sun and Hobert Citation2023). Specifically observing the anterior bundle of ganglia located at the pharynx, which can be considered the nematode brain, as the nematode ages the brain increases in connectivity and is remodelled, similar to other animals (Witvliet et al. Citation2021). With the increase in synaptic connectivity, behaviours change from adaption to learning to information processing, behaviours which are observed as the nematode ages (Witvliet et al. Citation2021), It should be noted that axons of nematode neurons are not myelinated (White et al. Citation1986), possibly due to their short length of less than 1 mm. Perhaps unique to C. elegans is the fact that nematode neurons do not require trophic support from glial cells, likely a consequence of the predetermined lineages of all C. elegans cells, including neurons (Bacaj et al. Citation2008; Yoshimura et al. Citation2008; Oikonomou and Shaham Citation2011; Shaham Citation2015). Specialised glial cells (or sheath cells) isolate the nerve ring, perhaps serving a similar function to that of the blood brain barrier in humans, although the function of such a barrier has yet to be firmly established (Oikonomou and Shaham Citation2011; Stout et al. Citation2014). Together, this provides significant opportunities to study the effect of glial cells on the nervous system to dissect glial-dependent processes in neurodevelopment (Oikonomou and Shaham Citation2011; Shaham Citation2015).
Due to its well characterised nervous system, C. elegans is increasingly used as a model for neurodevelopmental and neurotoxicity assessment (Avila et al. Citation2010; Ruszkiewicz et al. Citation2018; Silva MV Citation2020; Rapti Citation2020) (, ). Many mechanisms and KEs have been described for DNT in C. elegans (Li J et al. Citation2019) and these nematodes are also useful for exploring later KEs and AOs, such as behaviour and Parkinson’s Disease motor defects, which can be used as a substitute for mammalian testing (Sammi et al. Citation2019). It is compelling that C. elegans can be used to explore KEs at all life stages, as a “whole life approach”, meaning that KEs and AOs can be identified that are not often observed with short term exposures, and the exposures can be connected to specific vulnerable age groups (Hering et al. Citation2022).
Figure 2. Use of whole organism models in developmental neurotoxicity. The nematode Caenorhabditis elegans is a 3 R model throughout the duration of the 4-week life span. The zebrafish Danio rerio, is only considered a 3 R-model until 120 h post fertilisation (hpf). Both models develop a Central nervous system and neurobehaviour can be tested in various assays, for C. elegans, a mobility assays can be undertaken (Hughes et al. Citation2022) and in zebrafish larvae the light/dark transition test (Atzei et al. Citation2021). Figure generated with BioRender.com using authors’ own images and relevant references (Kalueff et al. Citation2014; Kozol et al. Citation2016; Hughes et al. Citation2022).
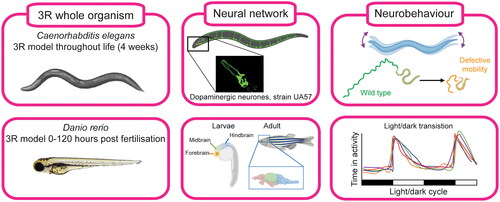
3.2. Zebrafish embryo as a model for DNT
Zebrafish (Danio rerio) are already well-established as a valuable model for studying chemical-induced environmental and human toxicology. By 5 dpf, the zebrafish have developed major tissues, including the brain and spinal cord (Panula et al. Citation2010). Zebrafish provide a powerful model as they are capable of external fertilisation, have high reproduction rates, and the embryos are small ranging from 0.7 mm as a newly fertilised zygote, to 0.9 mm at the 14-somite stage and up to approximately 1.5 mm just prior to hatching (Kimmel et al. Citation1995). At hatching, 3–4 dpf, the larvae are ∼3mm in length (Kimmel et al. Citation1995; Parichy et al. Citation2009) and transparent, allowing direct observation of developmental delay and malformations. Until 5–6 dpf, the developing zebrafish larvae feeds on its yolk thereby eliminating the need for nutritional supplementation and zebrafish up to 5 dpf are not considered experimental animals under the European legislation (Directive 2010/63/EU Citation2010; Strähle et al. Citation2012). The liver matures early, from 72 hpf, and is fully formed and active at 120 hpf (Loerracher and Braunbeck Citation2021). This enables the metabolism of toxicants, a process that is highly conserved with mammals (Goldstone et al. Citation2010; Nawaji et al. Citation2020).
There is a high level of evolutionary conservation of processes involved in the development of the zebrafish brain. Gastrulation starts at approximately 6 hpf, when body plan is established and cells that will give rise to the nervous system move to their positions (Kimmel et al. Citation1995; de Esch et al. Citation2012). The main brain structures, as well as many principal subdivisions of the brain, are found in the zebrafish and humans (Kalueff et al. Citation2014), including the hypothalamus, optic tracts, olfactory system, spinal cord and cranial nerves (Mueller and Wullimann Citation2003). By 24 hpf, brain morphogenesis has advanced with distinct regions present, namely the forebrain, including the diencephalon and telencephalon, midbrain, hindbrain and spinal cord, with clusters of neurons that are interconnected by axons (Hanneman and Westerfield Citation1989; Wilson et al. Citation1990). By 48 hpf the brain ventricles have been formed (de Esch et al. Citation2012) and the embryo starts to respond to touch stimuli (). Glial cell subtypes, such as oligodendrocytes, Schwann cells and astrocytes are also present by 48 hpf (Neely and Lyons Citation2021). A drawback is that the neocortex is not present in the zebrafish brain (Ijaz and Hoffman Citation2016), this is an important domain of the human brain that is required for higher cognitive processes (Diaz and Gleeson Citation2009; Galakhova et al. Citation2022). Similarly, the pons, cortico-thalamic and cortico-spinal tracts are structures that are found in the human brain, but absent in zebrafish (Kozol et al. Citation2016). Neurotransmitter systems, such as dopamine, GABA, glutamate, noradrenaline, serotonin, histamine, and acetylcholine are all present in zebrafish (Panula et al. Citation2010; Rico et al. Citation2011; Horzmann and Freeman Citation2016). The blood–brain barrier of zebrafish is fully functional at 10 dpf, and is based on endothelial tight junctions thus is quite similar to that of higher vertebrates (Rubin and Staddon Citation1999; Jeong et al. Citation2008). Additionally, the hypothalamus-pituitary-thyroid axis is conserved in zebrafish allowing detection of DNT precipitated by thyroid disrupting compounds by behavioural assays in ZFE and larvae up to 5 dpf (Walter et al. Citation2019; Couderq et al. Citation2020).
The rapid development of the zebrafish central nervous system allows the first functional test to take place at 24 hpf, while more advanced testing is possible from 4 dpf onwards. At 24 hpf it is possible to study the tail flick assay for DNT, since tail-coiling activity can be video tracked and measured in zebrafish (de Oliveira et al. Citation2021). Both tail-coiling and the photomotor response can be undertaken from 24 hpf and are therefore the first neurobehavioral tests that can be undertaken in ZFE and are considered early endpoints of nervous system functioning. Studies of zebrafish neuronal and behavioural functions have led to the establishment of models that assess sensory, motor and cognitive functions as well as models for neurological disorders (see reviews by (de Esch et al. Citation2012; Khan et al. Citation2017; Meshalkina et al. Citation2017). One of the suitable readouts for DNT testing in the zebrafish is stimulus-provoked locomotion, since this provides integrated information on the functionality and status of the entire nervous system of the ZFE (Atzei et al. Citation2021). Ultimately, behavioural studies have identified strong associations between the functions of zebrafish and human brain regions (Kalueff et al. Citation2014). Due to the high homology of many processes between zebrafish and human, zebrafish are a suitable and DNT relevant model. Further, AOs of compounds, such as the effects on neurobehavior, can be measured and can be linked to the underlying mechanism(s) by studying gene expression or thyroid hormone levels. Some early or lower order traits that might be linked to cognitive functioning can be measured in zebrafish models, which has been nicely reviewed by Stewart and Kalueff (Citation2012), but as many assays take place after 120 hpf, this is when the zebrafish is considered an animal experiment.
4. How to use whole organism models to fill the gaps in DNT
Including 3 R relevant whole-body organisms in DNT testing, in parallel with cell-based assays has significant added value. C. elegans and zebrafish have a high degree of conservation to mammals in terms of the conservation of (neuro)developmental processes (Lein et al. Citation2005) due to the highly similar functional characteristics of neurons, receptors, and neurotransmitters (Panula et al. Citation2010; Rico et al. Citation2011; Bargmann Citation2012; Bargmann and Marder Citation2013; Horzmann and Freeman Citation2016; Kozol et al. Citation2016; Hughes and Celikel Citation2019). Strikingly, these models also display early traits for cognitive functioning (Bahl and Engert Citation2020; Liu et al. Citation2020; Chai et al. Citation2022) including simple learning and memory formation (Roberts et al. Citation2013; Shomrat and Levin Citation2013; Inoue et al. Citation2015; Rahmani and Chew Citation2021; Van Damme et al. Citation2021; Reemst et al. Citation2023). In addition, C. elegans and zebrafish are relatively simple and cheap to maintain, compared to rodent models, and amenable to high throughput screening.
As shown in , there are a number of conserved neurodevelopmental processes which can be measured in cell-based assays, as well as in nematode and zebrafish models. The added value of the 3 R models, which currently utilise only cell-based assays, is that neurobehavior can be measured as well as the underlying mechanisms (the AO). Therefore, combining human-relevant cell-based assays with nematodes and zebrafish models will inform on how disruption of key neurodevelopmental processes might affect neurobehavior. The added value of the whole organism models for neurodevelopmental processes that are difficult to measure in cell-based assays alone (Paparella et al. Citation2020) will now be discussed in more detail.
4.1. Synaptogenesis and network formation
Synaptic function is crucial to regulate complex processes including sensory perception, learning, memory and decision-making. Synapses are specialised asymmetric cellular junctions between the presynaptic cell (the axon) and the post-synaptic cell (the dendrite or soma). The process of synaptogenesis is crucial for the formation of a neuronal network and is a highly conserved process across evolution (Cherra and Jin Citation2015). However, toxins are able to impact synaptogenesis, as defects in the process may lead to neurological, psychiatric and cognitive disorders (Vester and Caudle Citation2016). To this end, the impact of toxins, including pesticides and persistent organic pollutants on synaptogenesis is being explored using human iPSCs (Pamies et al. Citation2018; Pistollato et al. Citation2020; Zhong et al. Citation2020; Davidsen et al. Citation2021). Despite providing a platform to assess the effects of toxicants during neurodevelopment, modelling synaptogenesis in cell culture is particularly challenging and labour intensive (Rosca et al. Citation2020). To this end, it is possible to use whole-organism alternatives, which have the advantage of investigating the impact of compounds on the process of synaptogenesis and how this might affect neurobehavior.
Due to the simple fully mapped nervous system and availability of reporter strains and optogenetics, C. elegans is an excellent system with which to explore axon guidance (Quinn and Wadsworth Citation2008; Wu et al. Citation2011; Chen and Pan Citation2021), fate decision (Jukam and Desplan Citation2010) and synaptogenesis (Jin Citation2002; Cherra and Jin Citation2015; Hong and Park Citation2016; Mizumoto et al. Citation2023). During development from an early larval stage to adulthood, the geometry of the nematode brain is maintained, but there are changes in synaptic connectivity (Witvliet et al. Citation2021). Developments in microscopy techniques have provided valuable information on patterns of neural activity (Reviewed in Randi and Leifer (Citation2020) and (Emmons et al. Citation2021)) which further enables exploration of the impact of compounds on neurodevelopment.
Wiring diagrams for zebrafish are also available (Kunst et al. Citation2019) and these show that the zebrafish develops a complete brain including neuronal network functioning, for which proper synaptogenesis and dendritic spine morphology are crucial. In zebrafish, synaptogenesis progressively increases in all regions of the central nervous system during development. The availability of an electron microscopy dataset for a 5 dpf zebrafish brain allows for the identification of chemical synapses as well as enabling the structure-function relationship of neuronal activity to be elucidated (Svara et al. Citation2022), with new tools being designed to enable imaging of synapse dynamics in intact fish over a period of weeks (Du et al. Citation2018). By using transgenic lines in combination with confocal microscopy, it is possible to gain insight into how compound exposure might impact (neuro)behaviour in the ZFE (DeMarco et al. Citation2022). For example, dendritic spines are the location of excitatory synapses in the human brain, and disruption of their development is impeded in NDD. Using transgenic zebrafish lines enables the visualisation of these important neurons, how they develop and the impact of how compound exposure might affect synaptogenesis (Caioni et al. Citation2021; DeMarco et al. Citation2022).
4.2. Alteration in sensory function and motor function
Behaviour is dependent on the integration of different functions in the brain and between brain areas of the nervous system. Changes to behaviour as a consequence of toxin exposure can be used to detect the neurotoxic effects of compounds, with behaviour being 10–100 times more sensitive than survival as an endpoint (Gerhardt Citation2007; Peterson et al. Citation2017; Dutra Costa et al. Citation2020). Behavioural screening combines general morphological abnormalities in embryonic development with observations of behaviour in an intact animal. To assess impairment of neurobehavior, different tests are required to cover cognitive, sensory-motor, psychological and psychomotor parameters (Antonangeli et al. Citation2023). Most of the regulatory tests to assess neurobehaviour traditionally use rodents which are complex as well as resource and time expensive, however there is a shift towards the utilisation of 3 R whole organism models as suitable alternatives, specifically nematodes and zebrafish (Lein et al. Citation2005; Maximino et al. Citation2015; Ruszkiewicz et al. Citation2018; Silva MV Citation2020; Moulin et al. Citation2021).
In C. elegans, despite having a simple nervous system compared to higher organisms (Hughes and Celikel Citation2019) changes to behaviour are associated with different neuronal modalities. The nematodes display various neurobehaviors, including movement, attractive-repellent behaviour, egg-laying and feeding (Meyer and Williams Citation2014). Changes in behaviour are a useful indicator of toxicity (Chowdhury et al. Citation2021; Wang et al. Citation2021; Wang et al. Citation2023) and in C. elegans behaviour can be measured according to the distance moved, the number of body bends, head thrashing and swimming speed. Additional assays have been developed that employs a gel environment through which the worms burrow, an action that engages complex neuromuscular actuation (Lesanpezeshki et al. Citation2019). More complex neurobehavior involve the pharynx a neuromuscular pump at the anterior of the worm. Pump rate and timing are controlled by a series of 20 neurons (McVey et al. Citation2012) with deviations from the expected pumps per minute a sensitive and is powerful indicator of toxicity (Izquierdo et al. Citation2021; Wellenberg et al. Citation2021). Lastly, assessing the impact of compounds on sensory perception (chemotaxis) is already well established in ecotoxicology (Queirós et al. Citation2021; Zhou et al. Citation2022).
Since the focus is on 3 R models, only assays that are available before 120 hpf in the zebrafish will be highlighted here. Neurobehavior such as locomotor response can be assessed with automated video tracking systems. Common systems include EthoVision XT from Noldus (www.noldus.com) and the ZebraBox from Viewpoint (France, www.viewpoint.fr). Similar to mammals, zebrafish possess all of the classical sensory modalities such as vision, olfaction, taste, tactile, balance and hearing. Their sensory pathways share homology with humans (Kozol et al. Citation2016) and therefore testing behaviour assays in the zebrafish might be used to measure human-relevant DNT effects. In zebrafish, the motor network is initially quite simple and increases in complexity during development resulting in simple reflexive behaviours through to more complex decision-making behaviours (Saint-Amant Citation2006). Very early locomotor activity can be identified in ZFE at 24 hpf, as shown by spontaneous tail coiling (Selderslaghs et al. Citation2010; de Oliveira et al. Citation2021). Additionally, the photomotor response is an established and reproducible phenotype of the zebrafish embryo, observed 24 hpf in response to a predefined sequence of light stimuli without the need of developed eyes (Carbaugh et al. Citation2020). At 2 dpf, locomotion can be assessed by measuring the touch/escape response (Legradi et al. Citation2015). Here, upon a light tap to the head, the zebrafish embryo will display a burst of swimming with many sharp turns.
Concerning other behavioural features, as major neuromodulator systems are present by 96 hpf, zebrafish are able to exhibit coordinated behaviour in response to different stimuli (Saint-Amant Citation2006; Orger and de Polavieja Citation2017). Between 96 and 120 hpf various neurobehaviour assays are available in the zebrafish. For example, response to visual stimuli is measured in the light-dark transition test () and with a visual motor response test. The response to sound is measured in the acoustic startle response and the touch response (thigmotaxis) can also be observed (Rosa et al. Citation2022). All these behaviours are a result of the interaction of various brain regions and neurotransmitters systems, thus combining the visual motor response assays with acoustic startle response assays might distinguish compounds that cause ocular deficits resulting from toxic compound exposure and compounds that have direct effects on the nervous system.
4.3. Cognition, memory and learning
In addition to sensory and motor function defects, exposure to environmental compounds can also result in cognitive decline. Cognitive impairment, autism, attention-deficit hyperactivity disorder (ADHD) and dyslexia are just some of the NDDs that impact children (Grandjean and Landrigan Citation2014). While epidemiological studies have shown that toxins are a significant contributor to the increase in the number of childhood NDDs (Grandjean and Landrigan Citation2014), unravelling the causes of NDD-related cognitive decline is a challenge due to the complexity of the human brain and its development. Whole organism models in which overall regions of the central nervous system are conserved are interesting models to study parts of learning and memory. However, a better definition of “learning and memory” is urgently required, as currently this is a very broad category. In any case, the use of nematodes and zebrafish to provide a specific description of specific behaviour related to “learning and memory” and consequently provide a better description of the AO is possible. Although not as complex as human learning and memory formation, C. elegans and zebrafish can be used to study molecular mechanisms of simple learning and memory formation, thus providing a better understanding of how neural processes integrate different information to memory formation. Therefore, efforts have been made to develop learning paradigms in these model organisms (Reemst et al. Citation2023).
C. elegans has been shown to have the ability to both learn and remember, and their use in this field has enabled the cellular and molecular basis of learning and behaviour to be elucidated (reviewed in Rahmani and Chew (Citation2021). Despite having a compact nervous system, the fundamental mechanism of learning and memory in nematodes are conserved with vertebrates (Hawkins and Byrne Citation2015; Rahmani and Chew Citation2021; Van Damme et al. Citation2021). There are many examples of where nematodes display (non-)associative learning, imprinting and learning of smell and temperatures to avoid (Ardiel and Rankin Citation2010; Sasakura and Mori Citation2013). More importantly, the neural networks that govern these behaviours have been elucidated due to the simple and conserved sensorimotor network (Hughes and Celikel Citation2019). Similar to the rodent model, T-mazes have been 3D-printed and used with nematodes to identify different behaviours, including cognition and associative learning (Qin and Wheeler Citation2006; Oliver et al. Citation2016). Together, C. elegans is emerging as a useful model to explore simple cognition, and how ageing and exposure to toxins impact cognitive behaviours (Ardiel and Rankin Citation2010; Stein and Murphy Citation2012; Li Q et al. Citation2020).
Many basic neural systems mediating learning and memory are evolutionarily conserved in zebrafish. Mammalian brain regions known to be key for memory, including the hippocampus and amygdala, have functional homologous structures in the zebrafish brain (Kozol et al. Citation2016; Reemst et al. Citation2023). There are various assays in the zebrafish for learning and memory assays for non-associative learning (habituation, recognition and novelty learning) and associative learning (aversive and positive-reward based learning) (Reemst et al. Citation2023). Larval stages, up to 120 hpf, have been predominantly used to study habituation learning e.g. the response to an auditory, tractile or visual stimuli, which has been demonstrated as early as 4 dpf (Roberts et al. Citation2013). In addition to this relatively simple form of learning, it was reported that 6–8 dpf larvae can perform in an associative place-preference test. However, at these time points the zebrafish assays are considered animal experiments. Additionally, molecular markers and underlying mechanisms crucial for proper learning and memory can be studied in the zebrafish up to 120 hpf (Hinz et al. Citation2013) which can be predictive of AO at later stages of development.
5. Conclusions and future perspectives
The potential for integrating NAMs into DNT testing is clearly demonstrated by the use of the IVB (Masjosthusmann et al. Citation2020; Sachana, Shafer, et al. Citation2021). To this end, there is ongoing work by a number of parties to explore how to best integrate in vitro assays and non-mammalian models for DNT testing. These include the OECD, the USA Environmental Protection Agency and the National Toxicology Program in the USA who are supporting the inclusion of zebrafish embryo assays into the testing battery (Silva M et al. Citation2015; Behl et al. Citation2019; Marx-Stoelting et al. Citation2021; Muriana et al. Citation2021; Sachana, Shafer, et al. Citation2021).
The IVB are human cell-based assays that are capable of measuring proliferation, migration, differentiation, neurite outgrowth and neuronal network formation and function (Blum et al. Citation2022). However, it remains a struggle to identify perturbations at a molecular and cellular level associated with neurobehaviour and motor function defects or neurodevelopmental or neurodegenerative disorders (van Thriel et al. Citation2012; Bal-Price and Meek Citation2017). An additional challenge is evident when comparing the concentration-response and response-response relationships from individual in vitro assays to organism- and population-level endpoints, used to predict whole-body dose-response relationships that are required for safety risk assessment (Boyes et al. Citation2007), which have yet to be attempted.
In addition, many of the current toxicity tests that explore the effect of early exposure to toxins, do not consider the effects that may occur later in life. This is of importance in terms of the onset of neurodegenerative diseases, such as Alzheimer’s and Parkinson’s Disease, a set of age-related diseases that are increasing in prevalence and may have a developmental origin (Shan et al. Citation2023). Consequently, alternative models with a short lifespan are ideal, and C. elegans, which has a lifespan of 3–4 weeks and phenocopies many human age-related diseases, is a compelling solution. While lifespan experiments are possible in zebrafish, additional ethical documentation is required.
To this end, we propose that the nematode C. elegans and zebrafish D. rerio are highly promising alternative test models for testing DNT with good predictive power and complementary to cell-based in vitro models. While work is underway to include zebrafish in the DNT test battery (Silva M et al. Citation2015; Behl et al. Citation2019; Marx-Stoelting et al. Citation2021; Muriana et al. Citation2021; Sachana, Shafer, et al. Citation2021), the inclusion of C. elegans is lagging, despite the nematode having the potential to be a valuable asset to the DNT battery. Although chemical exposure in C. elegans are in agreement with other organisms (Boyd et al. Citation2010, Citation2016; Harlow et al. Citation2016; Racz et al. Citation2017; Wittkowski et al. Citation2019), a validated set of experimental guidelines are needed for nematode assays in DNT relating to human risk assessment. A starting point to show the advantages of these 3 R model systems and how they can be leveraged is to screen the same compounds across all organisms (Behl et al. Citation2019; Blum et al. Citation2022) and to predict DNT across a series of positive and negative compound sets (Kadereit et al. Citation2012; Mundy et al. Citation2015; Blum et al. Citation2022; Martin et al. Citation2022) to show the added value of the systems for regulatory purposes. Therefore, the results need to be discussed in the OECD DNT expert group, but first a ToxTemp protocol needs to be developed. This is an important first step to bring the model systems into the regulatory context. A challenge remains in linking and validating the data from these models to humans, but this is not insurmountable, as has been shown with zebrafish (Vorhees et al. Citation2021). The efforts of the OECD to include zebrafish embryo tests in the DNT test battery (Muriana et al. Citation2021; Sachana, Shafer, et al. Citation2021) shows that there is both interest and demand for the use of alternative models to fill gaps in the AOPs and paves the way for the same to be done using C. elegans.
The added value of these 3 R-whole organism systems to the current test battery enables later key events to be explored as well as the whole-life effect from early-life exposures. The ability to integrate assessments of compound exposure, with phenotypic effects as well as the genetic and molecular key events means that nematodes and zebrafish provide an extremely powerful approach to DNT in vitro assays, and they have an added value in combination with cell-based assays since later key events and simple AOs can be measured in these 3 R-whole organism systems.
Authors contributions
SH: methodology, investigation, writing (original draft), editing. EVSH: methodology, investigation, writing (original draft), editing.
Abbreviations | ||
3R | = | replace, reduce, refine |
AO | = | adverse outcome |
AOP | = | adverse outcome pathway |
DNT | = | developmental neurotoxicity |
dpf | = | days post fertilisation |
GABA | = | gamma-aminobutyric acid |
Hpf | = | hours post fertilisation |
iPSCs | = | induced pluripotent stem cells |
IVB | = | in vitro battery |
KE | = | key event |
MIE | = | molecular initiating event |
NAMs | = | new approach methodologies |
NDD | = | neurodevelopmental disorders |
OECD | = | organisation for economic co-operation and development |
OCED-TG | = | organisation for economic co-operation and development test guidelines |
US EPA | = | United States Environment Protection Agency |
ZFE | = | zebrafish embryos |
Acknowledgements
The authors would like to thank Dr. Nynke Kramer (Wageningen University and Research) and Dr. Victoria de Leeuw (RIVM) for discussions and critical reading of this manuscript. We are grateful to the anonymous external reviewers, selected by the Editor-in-Chief, who all provided valuable contributions to the final version of the manuscript.
Declaration of interest
The authors declare that they have no known competing financial interests or personal relationships that could have influenced the work reported in this review. Funding to EVSH was provided by the Dutch Ministry of Health Welfare and Sports. EVSH is a member of the OECD DNT Expert group and heading an OECD subgroup on the added value of the zebrafish for DNT.
References
- Abreu-Villaça Y, Levin ED. 2017. Developmental neurotoxicity of succeeding generations of insecticides. Environ Int. 99:55–77. doi: 10.1016/j.envint.2016.11.019.
- American Psychiatric Association. 2013. Diagnostic and statistical manual of mental disorders. 5th ed. Washington (DC): American Psychiatric Publishing.
- Antonangeli LM, Kenzhebekova S, Colosio C. 2023. Neurobehavioral effects of low-dose chronic exposure to insecticides: a review. Toxics. 11(2):192. doi: 10.3390/toxics11020192.
- Antunes Dos Santos A, Appel Hort M, Culbreth M, López-Granero C, Farina M, Rocha JB, Aschner M. 2016. Methylmercury and brain development: a review of recent literature. J Trace Elem Med Biol. 38:99–107. doi: 10.1016/j.jtemb.2016.03.001.
- Ardiel EL, Rankin CH. 2010. An elegant mind: learning and memory in Caenorhabditis elegans. Learn Mem. 17(4):191–201. doi: 10.1101/lm.960510.
- Aschner M, Ceccatelli S, Daneshian M, Fritsche E, Hasiwa N, Hartung T, Hogberg HT, Leist M, Li A, Mundi WR, et al. 2017. Reference compounds for alternative test methods to indicate developmental neurotoxicity (DNT) potential of chemicals: example lists and criteria for their selection and use. ALTEX. 34(1):49–74. doi: 10.14573/altex.1604201s.
- Astle DE, Holmes J, Kievit R, Gathercole SE. 2021. Annual Research Review: the transdiagnostic revolution in neurodevelopmental disorders. J Child Psychol Psychiatry. 63(4):397–417. doi: 10.1111/jcpp.13481.
- Atzei A, Jense I, Zwart EP, Legradi J, Venhuis BJ, van der Ven LTM, Heusinkveld HJ, Hessel EVS. 2021. Developmental neurotoxicity of environmentally relevant pharmaceuticals and mixtures thereof in a zebrafish embryo behavioural test. Int J Environ Res Public Health. 18(13):6717. doi: 10.3390/ijerph18136717.
- Avila D, Helmcke K, Aschner M. 2010. The Caenorhabditis elegans model as a reliable tool in neurotoxicology. Hum Exp Toxicol. 31(3):236–243. doi: 10.1177/0960327110392084.
- Bacaj T, Tevlin M, Lu Y, Shaham S. 2008. Glia are essential for sensory organ function in C. elegans. Science. 322(5902):744–747. doi: 10.1126/science.1163074.
- Bahl A, Engert F. 2020. Neural circuits for evidence accumulation and decision making in larval zebrafish. Nat Neurosci. 23(1):94–102. doi: 10.1038/s41593-019-0534-9.
- Bal-Price A, Lein PJ, Keil KP, Sethi S, Shafer T, Barenys M, Fritsche E, Sachana M, Meek ME. 2017. Developing and applying the adverse outcome pathway concept for understanding and predicting neurotoxicity. NeuroToxicology. 59:240–255. doi: 10.1016/j.neuro.2016.05.010.
- Bal-Price A, Meek MEB. 2017. Adverse outcome pathways: application to enhance mechanistic understanding of neurotoxicity. Pharmacol Ther. 179:84–95. doi: 10.1016/j.pharmthera.2017.05.006.
- Bal-Price A, Pistollato F, Sachana M, Bopp SK, Munn S, Worth A. 2018. Strategies to improve the regulatory assessment of developmental neurotoxicity (DNT) using in vitro methods. Toxicol Appl Pharmacol. 354:7–18. doi: 10.1016/j.taap.2018.02.008.
- Barbazuk WB, Korf I, Kadavi C, Heyen J, Tate S, Wun E, Bedell JA, McPherson JD, Johnson SL. 2000. The syntenic relationship of the zebrafish and human genomes. Genome Res. 10(9):1351–1358. doi: 10.1101/gr.144700.
- Bargmann CI. 1998. Neurobiology of the Caenorhabditis elegans genome. Science. 282(5396):2028–2033. doi: 10.1126/science.282.5396.2028.
- Bargmann CI. 2012. Beyond the connectome: how neuromodulators shape neural circuits. Bioessays. 34(6):458–465. doi: 10.1002/bies.201100185.
- Bargmann CI, Marder E. 2013. From the connectome to brain function. Nat Methods. 10(6):483–490. doi: 10.1038/nmeth.2451.
- Behl M, Ryan K, Hsieh J-H, Parham F, Shapiro AJ, Collins BJ, Sipes NS, Birnbaum LS, Bucher JR, Foster PMD, et al. 2019. Screening for developmental neurotoxicity at the national toxicology program: the future is here. Toxicol Sci. 167(1):6–14. doi: 10.1093/toxsci/kfy278.
- Bellinger DC. 2018. An overview of environmental chemical exposures and neurodevelopmental impairments in children. Pediatr Med. 1:9–9. doi: 10.21037/pm.2018.11.03.
- Blum J, Masjosthusmann S, Bartmann K, Bendt F, Dolde X, Dönmez A, Förster N, Holzer AK, Hübenthal U, Keßel HE, et al. 2022. Establishment of a human cell-based in vitro battery to assess developmental neurotoxicity hazard of chemicals. Chemosphere. 311(Pt 2):137035. doi: 10.1016/j.chemosphere.2022.137035.
- Bourgeron T. 2015. What do we know about early onset neurodevelopmental disorders? Cambridge (MA): MIT Press.
- Boyd WA, McBride SJ, Rice JR, Snyder DW, Freedman JH. 2010. A high-throughput method for assessing chemical toxicity using a Caenorhabditis elegans reproduction assay. Toxicol Appl Pharmacol. 245(2):153–159. doi: 10.1016/j.taap.2010.02.014.
- Boyd WA, Smith MV, Co CA, Pirone JR, Rice JR, Shockley KR, Freedman JH. 2016. Developmental effects of the ToxCastTM phase I and phase II chemicals in Caenorhabditis elegans and corresponding responses in zebrafish, rats and rabbits. Environ Health Perspect. 124(5):586–593. doi: 10.1289/ehp.1409645.
- Boyes WK, Moser VC, Geller AM, Benignus VA, Bushnell PJ, Kamel F. 2007. Integrating epidemiology and toxicology in neurotoxicity risk assessment. Hum Exp Toxicol. 26(4):283–293. doi: 10.1177/0960327106070481.
- Brittin CA, Cook SJ, Hall DH, Emmons SW, Cohen N. 2021. A multi-scale brain map derived from whole-brain volumetric reconstructions. Nature. 591(7848):105–110. doi: 10.1038/s41586-021-03284-x.
- Brownlee DJ, Fairweather I. 1999. Exploring the neurotransmitter labyrinth in nematodes. Trends Neurosci. 22(1):16–24. doi: 10.1016/s0166-2236(98)01281-8.
- Brox S, Seiwert B, Küster E, Reemtsma T. 2016. Toxicokinetics of polar chemicals in zebrafish embryo (Danio rerio): influence of physicochemical properties and of biological processes. Environ Sci Technol. 50(18):10264–10272. doi: 10.1021/acs.est.6b04325.
- Caioni G, Merola C, Perugini M, d‘Angelo M, Cimini AM, Amorena M, Benedetti E. 2021. An experimental approach to study the effects of realistic environmental mixture of linuron and propamocarb on zebrafish synaptogenesis. Int J Environ Res Public Health. 18(9):4664. doi: 10.3390/ijerph18094664.
- Carbaugh CM, Widder MW, Phillips CS, Jackson DA, DiVito VT, van der Schalie WH, Glover KP. 2020. Assessment of zebrafish embryo photomotor response sensitivity and phase-specific patterns following acute- and long-duration exposure to neurotoxic chemicals and chemical weapon precursors. J Appl Toxicol. 40(9):1272–1283. doi: 10.1002/jat.3984.
- Carstens KE, Freudenrich T, Wallace K, Choo S, Carpenter A, Smeltz M, Clifton MS, Henderson WM, Richard AM, Patlewicz G, et al. 2023. Evaluation of per- and polyfluoroalkyl substances (PFAS) in vitro toxicity testing for developmental neurotoxicity. Chem Res Toxicol. 36(3):402–419. doi: 10.1021/acs.chemrestox.2c00344.
- Chai CM, Torkashvand M, Seyedolmohadesin M, Park H, Venkatachalam V, Sternberg PW. 2022. Interneuron control of C. elegans developmental decision-making. Curr Biol. 32(10):2316–2324.e2314. doi: 10.1016/j.cub.2022.03.077.
- Chen C-H, Pan C-L. 2021. Live-cell imaging of PVD dendritic growth cone in post-embryonic C. elegans. STAR Protocols. 18(2):100402.
- Cheroni C, Caporale N, Testa G. 2020. Autism spectrum disorder at the crossroad between genes and environment: contributions, convergences, and interactions in ASD developmental pathophysiology. Mol Autism. 11(1):69. doi: 10.1186/s13229-020-00370-1.
- Cherra SJ, III, Jin Y. 2015. Advances in synapse formation: foraging connections in the worms. Wiley Interdiscip Rev Dev Biol. 4(2):85–97. doi: 10.1002/wdev.165.
- Chowdhury MI, Sana T, Panneerselvan L, Dharmarajan R, Megharaj M. 2021. Acute toxicity and transgenerational effects of perfluorobutane sulfonate on Caenorhabditis elegans. Environ Toxicol Chem. 40(7):1973–1982. doi: 10.1002/etc.5055.
- Cook SJ, Jarrell TA, Brittin CA, Wang Y, Bloniarz AE, Yakovlev MA, Nguyen KCQ, Tang LT-H, Bayer EA, Duerr JS, et al. 2019. Whole-animal connectomes of both Caenorhabditis elegans sexes. Nature. 571(7763):63–71. doi: 10.1038/s41586-019-1352-7.
- Cook SJ, Kalinski CA, Hobert O. 2023. Neuronal contact predicts connectivity in the C. elegans brain. Curr Biol. 33(11):2315–2320.e2. doi: 10.1016/j.cub.2023.04.071.
- Corsi AK, Wightman B, Chalfie M. 2015. A transparent window into biology: a primer on Caenorhabditis elegans. Genetics. 200(2):387–407. doi: 10.1534/genetics.115.176099.
- Couderq A, Leemans M, Fini J-B. 2020. Testing for thyroid hormone disruptors, a review of non-mammalian in vivo models. Mol Cell Endocrinol. 508:110779. doi: 10.1016/j.mce.2020.110779.
- Crofton KM, Mundy WR. 2021. External scientific report on the interpretation of data from the developmental neurotoxicity in vitro testing assays for use in integrated approaches for testing and assessment. EFS3. 18(10):6924E. doi: 10.2903/sp.efsa.2021.EN-6924.
- Cronin CJ, Mendel JE, Mukhtar S, Kim YM, Stirbl RC, Bruck J, Sternberg PW. 2005. An automated system for measuring parameters of nematode sinusoidal movement. BMC Genet. 6(1):5. doi: 10.1186/1471-2156-6-5.
- Davidsen N, Lauvås AJ, Myhre O, Ropstad E, Carpi D, Gyves EM, Berntsen HF, Dirven H, Paulsen RE, Bal-Price A, et al. 2021. Exposure to human relevant mixtures of halogenated persistent organic pollutants (POPs) alters neurodevelopmental processes in human neural stem cells undergoing differentiation. Reprod Toxicol. 100:17–34. doi: 10.1016/j.reprotox.2020.12.013.
- de Esch C, Slieker R, Wolterbeek A, Woutersen R, de Groot D. 2012. Zebrafish as potential model for developmental neurotoxicity testing: a mini review. Neurotoxicol Teratol. 34(6):545–553. doi: 10.1016/j.ntt.2012.08.006.
- De Felice A, Ricceri L, Venerosi A, Chiarotti F, Calamandrei G. 2015. Multifactorial origin of neurodevelopmental disorders: approaches to understanding complex etiologies. Toxics. 3(1):89–129. doi: 10.3390/toxics3010089.
- de Oliveira AAS, Brigante TAV, Oliveira DP. 2021. Tail coiling assay in zebrafish (Danio rerio) embryos: stage of development, promising positive control candidates, and selection of an appropriate organic solvent for screening of developmental neurotoxicity (DNT). Water. 13(2):119. doi: 10.3390/w13020119.
- DeMarco EC, Stoner GR, Robles E. 2022. A genetic labeling system to study dendritic spine development in zebrafish models of neurodevelopmental disorders. Dis Model Mech. 15(8):dmm049507.
- Desprez B, Birk B, Blaauboer B, Boobis A, Carmichael P, Cronin MTD, Curie R, Daston G, Hubesch B, Jennings P, et al. 2019. A mode-of-action ontology model for safety evaluation of chemicals: outcome of a series of workshops on repeated dose toxicity. Toxicol in Vitro. 59:44–50. doi: 10.1016/j.tiv.2019.04.005.
- Dexter PM, Caldwell KA, Caldwell GA. 2012. A predictable worm: application of Caenorhabditis elegans for mechanistic investigation of movement disorders. Neurotherapeutics. 9(2):393–404. doi: 10.1007/s13311-012-0109-x.
- Diaz AL, Gleeson JG. 2009. The molecular and genetic mechanisms of neocortex development. Clin Perinatol. 36(3):503–512. doi: 10.1016/j.clp.2009.06.008.
- Directive 2010/63/EU. 2010. Directive 2010/63/EU of the European Parliament and of the Council of 22 September 2010 on the protection of animals used for scientific purposes. Official Journal of the European Union. 276:33–79.
- Du XF, Xu B, Zhang Y, Chen MJ, Du JL. 2018. A transgenic zebrafish model for in vivo long-term imaging of retinotectal synaptogenesis. Sci Rep. 8(1):14077. doi: 10.1038/s41598-018-32409-y.
- Dutra Costa BP, Aquino Moura L, Gomes Pinto SA, Lima-Maximino M, Maximino C. 2020. Zebrafish models in neural and behavioral toxicology across the life stages. Fishes. 5(3):23. doi: 10.3390/fishes5030023.
- Emmons SW, Yemini E, Zimmer M. 2021. Methods for analyzing neuronal structure and activity in Caenorhabditis elegans. Genetics. 218(4):iyab072. doi: 10.1093/genetics/iyab072.
- Fritsche E, Barenys M, Klose J, Masjosthusmann S, Nimtz L, Schmuck M, Wuttke S, Tigges J. 2018. Current availablity of stem cell-based in vitro methods for developmental neurotoxicity (DNT) testing. Toxicol Sci. 165(1):21–30. doi: 10.1093/toxsci/kfy178.
- Fritsche E, Grandjean P, Crofton KM, Aschner M, Goldberg A, Heinonen T, Hessel EVS, Hogberg HT, Bennekou SH, Lein PJ, et al. 2018. Consensus statement on the need for innovation, transition and implementation of developmental neurotoxicity (DNT) testing for regulatory purposes. Toxicol Appl Pharmacol. 354:3–6. doi: 10.1016/j.taap.2018.02.004.
- Galakhova AA, Hunt S, Wilbers R, Heyer DB, de Kock CPJ, Mansvelder HD, Goriounova NA. 2022. Evolution of cortical neurons supporting human cognition. Trends Cogn Sci. 26(11):909–922. doi: 10.1016/j.tics.2022.08.012.
- Gerhardt A. 2007. Aquatic behavioral ecotoxicology—prospects and limitations. Hum Ecol Risk Assess Int J. 13(3):481–491. doi: 10.1080/10807030701340839.
- Goldstone JV, McArthur AG, Kubota A, Zanette J, Parente T, Jönsson ME, Nelson DR, Stegeman JJ. 2010. Identification and developmental expression of the full complement of Cytochrome P450 genes in zebrafish. BMC Genomics. 11(1):643. doi: 10.1186/1471-2164-11-643.
- Grandjean P, Landrigan PJ. 2014. Neurobehavioural effects of developmental toxicity. Lancet Neurol. 13(3):330–338. doi: 10.1016/S1474-4422(13)70278-3.
- Haddad-Tóvolli R, Dragano NRV, Ramalho AFS, Velloso LA. 2017. Development and function of the blood-brain barrier in the context of metabolic control. Front Neurosci. 11:224. doi: 10.3389/fnins.2017.00224.
- Hanneman E, Westerfield M. 1989. Early expression of acetylcholinesterase activity in functionally distinct neurons of the zebrafish. J Comp Neurol. 284(3):350–361. doi: 10.1002/cne.902840303.
- Harlow PH, Perry SJ, Widdison S, Daniels S, Bondo E, Lamberth C, Currie RA, Flemming AJ. 2016. The nematode Caenorhabditis elegans as a tool to predict chemical activity on mammalian development and identify mechanisms influencing toxicological outcome. Sci Rep. 6(1):22965. doi: 10.1038/srep22965.
- Hawkins RD, Byrne JH. 2015. Associative learning in invertebrates. Cold Spring Harb Perspect Biol. 7(5):a021709. doi: 10.1101/cshperspect.a021709.
- Haynes EM, Ulland TK, Eliceiri KW. 2022. A model of discovery: the role of imaging established and emerging non-mammalian models in neuroscience. Front Mol Neurosci. 15:867010. doi: 10.3389/fnmol.2022.867010.
- Hering I, Le DT, von Mikecz A. 2022. How to keep up with the analysis of classic and emerging neurotoxins: age-resolved fitness tests in the animal model Caenorhabditis elegans - a step-by-step protocol. Excli J. 21:344–353
- Hessel EVS, Staal YCM, Piersma AH. 2018. Design and validation of an ontology-driven animal-free testing strategy for developmental neurotoxicity testing. Toxicol Appl Pharmacol. 354:136–152. doi: 10.1016/j.taap.2018.03.013.
- Heyer DB, Meredith RM. 2017. Environmental toxicology: sensitive periods of development and neurodevelopmental disorders. Neurotoxicology. 58:23–41. doi: 10.1016/j.neuro.2016.10.017.
- Hillier LW, Coulson A, Murray JI, Bao Z, Sulston JE, Waterston RH. 2005. Genomics in C. elegans: so many genes, such a little worm. Genome Res. 15(12):1651–1660. doi: 10.1101/gr.3729105.
- Hinz FI, Aizenberg M, Tushev G, Schuman EM. 2013. Protein synthesis-dependent associative long-term memory in larval zebrafish. J Neurosci. 33(39):15382–15387. doi: 10.1523/JNEUROSCI.0560-13.2013.
- Hong J-H, Park M. 2016. Understanding synaptogenesis and functional connectome in C. elegans by imaging technology. Front Synaptic Neurosci. 8:18. doi: 10.3389/fnsyn.2016.00018.
- Horzmann KA, Freeman JL. 2016. Zebrafish get connected: investigating neurotransmission targets and alterations in chemical toxicity. Toxics. 4(3):19. doi: 10.3390/toxics4030019.
- Howe K, Clark MD, Torroja CF, Torrance J, Berthelot C, Muffato M, Collins JE, Humphray S, McLaren K, Matthews L, et al. 2013. The zebrafish reference genome sequence and its relationship to the human genome. Nature. 496(7446):498–503. doi: 10.1038/nature12111.
- Hughes S, Celikel T. 2019. Prominent inhibitory projections guide sensorimotor computation: an invertebrate perspective. Bioessays. 41(10):e1900088. doi: 10.1002/bies.201900088.
- Hughes S, van Dop M, Kolsters N, van de Klashorst D, Pogosova A, Rijs AM. 2022. Using a Caenorhabditis elegans Parkinson’s disease model to assess disease progression and therapy efficiency. Pharmaceuticals. 15(5):512. doi: 10.3390/ph15050512.
- Husson SJ, Costa WS, Schmitt C, Gottschalk A. 2012. Keeping track of worm trackers. The C. elegans Research Community. Pasadena (CA): WormBook. doi: 10.1895/wormbook.1.150.1.
- Ijaz S, Hoffman EJ. 2016. Zebrafish: a translational model system for studying neuropsychiatric disorders. J Am Acad Child Adolesc Psychiatry. 55(9):746–748. doi: 10.1016/j.jaac.2016.06.008.
- Inoue T, Hoshino H, Yamashita T, Shimoyama S, Agata K. 2015. Planarian shows decision-making behavior in response to multiple stimuli by integrative brain function. Zoological Lett. 1(1):7. doi: 10.1186/s40851-014-0010-z.
- Izquierdo PG, O'Connor V, Green AC, Holden-Dye L, Tattersall JEH. 2021. C. elegans pharyngeal pumping provides a whole organism bio-assay to investigate anti-cholinesterase intoxication and antidotes. Neurotoxicology. 82:50–62. doi: 10.1016/j.neuro.2020.11.001.
- Javer A, Ripoll-Sánchez L, Brown AEX. 2018. Powerful and interpretable behavioural features for quantitative phenotyping of Caenorhabditis elegans. Philos Trans R Soc Lond B Biol Sci. 373(1758):20170375. doi: 10.1098/rstb.2017.0375.
- Jedynak P, Maitre L, Guxens M, Gützkow KB, Julvez J, López-Vicente M, Sunyer J, Casas M, Chatzi L, Gražulevičienė R, et al. 2021. Prenatal exposure to a wide range of environmental chemicals and child behaviour between 3 and 7 years of age - an exposome-based approach in 5 European cohorts. Sci Total Environ. 763:144115. doi: 10.1016/j.scitotenv.2020.144115.
- Jeong J-J, Kwon H-B, Ahn J-C, Kang D, Kwon S-H, Park JA, Kim K-W. 2008. Functional and developmental analysis of the blood-brain barrier in zebrafish. Brain Res Bull. 75(5):619–628. doi: 10.1016/j.brainresbull.2007.10.043.
- Jin Y. 2002. Synpatogenesis: insights from worm and fly. Curr Opin Neurobiol. 12(1):71–79. doi: 10.1016/s0959-4388(02)00292-1.
- Jukam D, Desplan C. 2010. Binary fate decision in differentiating neurons. Curr Opin Neurobiol. 20(1):6–13. doi: 10.1016/j.conb.2009.11.002.
- Julvez J, López-Vicente M, Warembourg C, Maitre L, Philippat C, Gützkow KB, Guxens M, Evandt J, Andrusaityte S, Burgaleta M, et al. 2021. Early life multiple exposures and child cognitive function: a multi-centric birth cohort study in six European countries. Environ Pollut. 284:117404. doi: 10.1016/j.envpol.2021.117404.
- Kadereit S, Zimmer B, van Thriel C, Hengstler JG, Leist M. 2012. Compound selection for in vitro modeling of developmental neurotoxicity. Front Biosci (Landmark Ed). 17(7):2442–2460. doi: 10.2741/4064.
- Kaletta T, Hengartner MO. 2006. Finding function in novel targets: C. elegans as a model organism. Nat Rev Drug Discov. 5(5):387–398. doi: 10.1038/nrd2031.
- Kalueff AV, Echevarria DJ, Stewart AM. 2014. Gaining translational momentum: more zebrafish models for neuroscience research. Prog Neuropsychopharmacol Biol Psychiatry. 55:1–6. doi: 10.1016/j.pnpbp.2014.01.022.
- Keeney JG, Davis JM, Siegenthaler J, Post MD, Nielsen BS, Hopkins WD, Sikela JM. 2015. DUF1220 protein domains drive proliferation in human neural stem cells and are associated with increased cortical volume in anthropoid primates. Brain Struct Funct. 220(5):3053–3060. doi: 10.1007/s00429-014-0814-9.
- Khan KM, Collier AD, Meshalkina DA, Kysil EV, Khatsko SL, Kolesnikova T, Morzherin YY, Warnick JE, Kalueff AV, Echevarria DJ. 2017. Zebrafish models in neuropsychopharmacology and CNS drug discovery. Br J Pharmacol. 174(13):1925–1944. doi: 10.1111/bph.13754.
- Kim W, Underwood RS, Greenwald I, Shaye DD. 2018. OrthoList 2: a new comparative genomic analysis of human and Caenorhabditis elegans Genes. Genetics. 210(2):445–461. doi: 10.1534/genetics.118.301307.
- Kimmel CB, Ballard WW, Kimmel SR, Ullmann B, Schilling TF. 1995. Stages of embryonic development of the zebrafish. Dev Dyn. 203(3):253–310. doi: 10.1002/aja.1002030302.
- Kozol RA, Abrams AJ, James DM, Buglo E, Yan Q, Dallman JE. 2016. Function over form: modeling groups of inherited neurological conditions in Zebrafish. Front Mol Neurosci. 9:55. doi: 10.3389/fnmol.2016.00055.
- Kunst M, Laurell E, Mokayes N, Kramer A, Kubo F, Fernandes AM, Förster D, Dal Maschio M, Baier H. 2019. A cellular-resolution atlas of the larval Zebrafish brain. Neuron. 103(1):21–38.e25. doi: 10.1016/j.neuron.2019.04.034.
- Lai CH, Chou CY, Ch’ang LY, Liu CS, Lin W. 2000. Identification of novel human genes evolutionarily conserved in Caenorhabditis elegans by comparative proteomics. Genome Res. 10(5):703–713. doi: 10.1101/gr.10.5.703.
- Landrigan PJ, Lambertini L, Birnbaum LS. 2012. A research strategy to discover the environmental causes of autism and neurodevelopmental disabilities. Environ Health Perspect. 120(7):a258–260. doi: 10.1289/ehp.1104285.
- Legradi J, el Abdellaoui N, van Pomeren M, Legler J. 2015. Comparability of behavioural assays suing zebrafish larvae to assess neurotoxicity. Environ Sci Pollut Res Int. 22(21):16277–16289. doi: 10.1007/s11356-014-3805-8.
- Lein P, Silbergeld E, Locke P, Goldberg AM. 2005. In vitro and other alternative approaches to developmental neurotoxicity testing (DNT). Environ Toxicol Pharmacol. 19(3):735–744. doi: 10.1016/j.etap.2004.12.035.
- Lesanpezeshki L, Hewitt JE, Laranjeiro R, Antebi A, Driscoll M, Szewczyk NJ, Blawzdziewicz J, Lacerda CMR, Vanapalli SA. 2019. Pluronic gel-based burrowing assay for rapid assessment of neuromuscular health in C. elegans. Sci Rep. 9(1):15246. doi: 10.1038/s41598-019-51608-9.
- Li J, Settivari R, LeBaron MJ, Marty MS. 2019. An industry perspective: a streamlined screening strategy using alternative models for chemical assessment of developmental neurotoxicity. NeuroToxicology. 73:17–30. doi: 10.1016/j.neuro.2019.02.010.
- Li Q, Marcu D-C, Palazzo O, Turner F, King D, Spires-Jones TL, Stefan MI, Busch KE. 2020. High neural activity accelerates the decline of cognitive plasticity with age in Caenorhabditis elegans. Elife. 9:e59711. doi: 10.7554/eLife.59711.
- Lidsky TI, Schneider JS. 2003. Lead neurotoxicity in children: basic mechanisms and clinical correlates. Brain. 126(Pt 1):5–19. doi: 10.1093/brain/awg014.
- Liu P, Chen B, Wang Z-W. 2020. GABAergic motor neurons bias locomotor decision-making in C. elegans. Nat Commun. 11(1):5076. doi: 10.1038/s41467-020-18893-9.
- Loerracher AK, Braunbeck T. 2021. Cytochrome P450-dependent biotransformation capacities in embryonic, juvenile and adult stages of zebrafish (Danio rerio)-a state-of-the-art review. Arch Toxicol. 95(7):2299–2334. doi: 10.1007/s00204-021-03071-7.
- Makris SL, Raffaele K, Allen S, Bowers WJ, Hass U, Alleva E, Calamandrei G, Sheets L, Amcoff P, Delrue N, et al. 2009. A retrospective performance assessment of the developmental neurotoxicity study in support of OECD test guideline 426. Environ Health Perspect. 117(1):17–25. doi: 10.1289/ehp.11447.
- Martin MM, Baker NC, Boyes WK, Carstens KE, Culbreth ME, Gilbert ME, Harrill JA, Nyffeler J, Padilla S, Friedman KP, et al. 2022. An expert-driven literature review of "negative" chemicals for developmental neurotoxicity (DNT) in vitro assay evaluation. Neurotoxicol Teratol. 93:107117. doi: 10.1016/j.ntt.2022.107117.
- Marx-Stoelting P, Solano MdLM, Aoyama H, Adams RH, Bal-Price A, Buschmann J, Chahoud I, Clark R, Fang T, Fujiwara M, et al. 2021. 25th anniversary of the Berlin workshop on developmental toxicology: devTox database update, challenges in risk assessment of developmental neurotoxicity and alternative methodologies in bone development and growth. Reprod Toxicol. 100:155–162. doi: 10.1016/j.reprotox.2020.11.003.
- Masjosthusmann S, Blum J, Bartmann K, Dolde X, Holzer A, Stürzl L, Keßel EH, Förster N, Dönmez A, Klose J, et al. 2020. Establishment of an a priori protocol for the implementation and interpretation of an in-vitro testing battery for the assessment of developmental neurotoxicity. EFS3. 17(10):1938E. doi: 10.2903/sp.efsa.2020.EN-1938.
- Maximino C, Silva RXdC, da Silva SdNS, Rodrigues LdSDS, Barbosa H, de Carvalho TS, Leão LKDR, Lima MG, Oliveira KRM, Herculano AM. 2015. Non-mammalian models in behavioral neuroscience: consequences for biological psychiatry. Front Behav Neurosci. 9:233. doi: 10.3389/fnbeh.2015.00233.
- McVey KA, Mink JA, Snapp i, Timberlake WS, Todt CE, Negga R, Fitsanakis VA. 2012. Caenorhabditis elegans: an emerging model system for pesticide neurotoxicity. Enviro Anal Toxicol. S4:003.
- Meshalkina DA, Kysil EV, Warnick JE, Demin KA, Kalueff AV. 2017. Adult zebrafish in CNS disease modelling: a tank that’s half-full not half-empty, and still filling. Lab Anim. 46(10):378–387. doi: 10.1038/laban.1345.
- Meyer D, Williams PL. 2014. Toxicity testing of neurotoxic pesticides in Caenorhabditis elegans. J Toxicol Environ Health B Crit Rev. 17(5):284–306. doi: 10.1080/10937404.2014.933722.
- Mizumoto K, Jin Y, Bessereau J-L. 2023. Synaptogenesis: unmasking molecular mechanims using Caenorhabditis elegans. Genetics. 223(2):1–26. doi: 10.1093/genetics/iyac176.
- Moulin TC, Covill LE, Itskov PM, Williams MJ, Schiöth HB. 2021. Rodent and fly models in behavioral neuroscience: an evaluation of methodological advances, comparative research, and future perspectives. Neurosci Biobehav Rev. 120:1–12. doi: 10.1016/j.neubiorev.2020.11.014.
- Mueller T, Wullimann MF. 2003. Anatomy of neurogenesis in the early zebrafish brain. Brain Res Dev Brain Res. 140(1):137–155. doi: 10.1016/s0165-3806(02)00583-7.
- Mundy WR, Padilla S, Breier JM, Crofton KM, Gilbert ME, Herr DW, Jensen KF, Radio NM, Raffaele KC, Schumacher K, et al. 2015. Expanding the test set: chemicals with potential to disrupt mammalian brain development. Neurotoxicol Teratol. 52(Pt A):25–35. doi: 10.1016/j.ntt.2015.10.001.
- Muriana A, Alzualde A, Hsieh J, Ryan K, Behl M, Terron A, Woodland C, Kluver N, Hessel EVS, Ellis L, et al. 2021. An Inter-laboratory case study to harmonize zebrafish light-dark transition test to predict developmental neurotoxicity (WC11). 11th World Congress on Alternative and Animal Use in the Life Sciences 2021; Maastricht, Netherlands.
- Nagai J, Yu X, Papouin T, Cheong E, Freeman MR, Monk KR, Hastings MH, Haydon PG, Rowitch D, Shaham S, et al. 2021. Behaviorally consequential astrocytic regulation of neural circuits. Neuron. 109(4):576–596. doi: 10.1016/j.neuron.2020.12.008.
- Nannaware M, Mayilswamy N, Kandasubramanian B. 2024. PFAS: exploration of neurotoxicity and environmental impact. Environ Sci Pollut Res Int. 31(9):12815–12831. doi: 10.1007/s11356-024-32082-x.
- Nawaji T, Yamashita N, Umeda H, Zhang S, Mizoguchi N, Seki M, Kitazawa T, Teraoka H. 2020. Cytochrome P450 expression and chemical metabolic activity before full liver development in zebrafish. Pharmaceuticals. 13(12):456. doi: 10.3390/ph13120456.
- Neely SA, Lyons DA. 2021. Insights into central nervous system glial cell formation and function from zebrafish. Front Cell Dev Biol. 9:754606. doi: 10.3389/fcell.2021.754606.
- OECD. 2007. Test no. 426: developmental neurotoxicity study. Paris: OECD.
- OECD. 2018a. Developmental neurotoxicity study (OECD TG 426). Paris: OECD.
- OECD. 2018b. Test no. 443: extended one-generation reproductive toxicity study. Paris: OECD.
- Oh J, Shin HM, Kannan K, Busgang SA, Schmidt RJ, Schweitzer JB, Hertz-Picciotto I, Bennett DH. 2022. Childhood exposure to per- and polyfluoroalkyl substances and neurodevelopment in the CHARGE case-control study. Environ Res. 215(Pt 2):114322. doi: 10.1016/j.envres.2022.114322.
- Oikonomou G, Shaham S. 2011. The glia of Caenorhabditis elegans. Glia. 59(9):1253–1263. doi: 10.1002/glia.21084.
- Oliver CR, Gourgou E, Bazopoulou D, Chronis N, Hart NJ. 2016. On-demand isolation and manipulation of C. elegans by in vitro maskless photopatterning. PLoS One. 11(1):e0145935. doi: 10.1371/journal.pone.0145935.
- Orger MB, de Polavieja GG. 2017. Zebrafish behavior: opportunities and challenges. Annu Rev Neurosci. 40(1):125–147. doi: 10.1146/annurev-neuro-071714-033857.
- Pamies D, Block K, Lau P, Gribaldo L, Pardo CA, Barreras P, Smirnova L, Wiersma D, Zhao L, Harris G, et al. 2018. Rotenone exerts developmental neurotoxicity in a human brain spheroid model. Toxicol Appl Pharmacol. 354:101–114. doi: 10.1016/j.taap.2018.02.003.
- Panula P, Chen Y-C, Priyadarshini M, Kudo H, Semenova S, Sundvik M, Sallinen V. 2010. The comparative neuroanatomy and neurochemistry of zebrafish CNS systems of relevance to human neuropsychiatric diseases. Neurobiological Disorders. 40(1):46–57.
- Paparella M, Bennekou SH, Bal-Price A. 2020. An analysis of the limitations and uncertainties of in vivo developmental neurotoxicity testing and assessment to identify the potential for alternative approaches. Reprod Toxicol. 96:327–336. doi: 10.1016/j.reprotox.2020.08.002.
- Parichy DM, Elizondo MR, Mills MG, Gordon TN, Engeszer RE. 2009. Normal table of postembryonic zebrafish development: staging by externally visible anatomy of the living fish. Dev Dyn. 238(12):2975–3015. doi: 10.1002/dvdy.22113.
- Peterson EK, Buchwalter DB, Kerby JL, LeFauve MK, Varian-Ramos CW, Swaddle JP. 2017. Integrative behavioral ecotoxicology: bringing together fields to establish new insight to behavioral ecology, toxicology, and conservation. Curr Zool. 63(2):185–194. doi: 10.1093/cz/zox010.
- Pistollato F, Mendoza de Gyves E, Carpi D, Bopp SK, Nunes C, Worth A, Bal-Price A. 2020. Assessment of developmental neurotoxicity induced by chemical mixtures using an adverse outcome pathway concept. Environ Health. 19(1):23. doi: 10.1186/s12940-020-00578-x.
- Postlethwait JH, Woods IG, Ngo-Hazelett P, Yan YL, Kelly PD, Chu F, Huang H, Hill-Force A, Talbot WS. 2000. Zebrafish comparative genomics and the origins of vertebrate chromosomes. Genome Res. 10(12):1890–1902. doi: 10.1101/gr.164800.
- Qin J, Wheeler AR. 2006. Maze exploration and learning in C. elegans. Lab Chip. 2007(7):186–192.
- Queirós L, Marques C, Pereira JL, Gonçalves FJM, Aschner M, Pereira P. 2021. Overview of chemotaxis behavior assays in Caenorhabditis elegans. Curr Protoc. 1(5):e120. eng.
- Quinn CC, Wadsworth WG. 2008. Axon guidance: asymmetric signalling orients polarised outgrowth. Trends Cell Biol. 18(12):597–603. doi: 10.1016/j.tcb.2008.09.005.
- Racz PI, Wildwater M, Rooseboom M, Kerkhof E, Pieters R, Yebra-Pimentel ES, Dirks RP, Spaink HP, Smulders C, Whale GF. 2017. Application of Caenorhabditis elegans (nematode) and Danio rerio embryo (zebrafish) as model systems to screen for developmental and reproductive toxicity of Piperazine compounds. Toxicol in Vitro. 44:11–16. doi: 10.1016/j.tiv.2017.06.002.
- Rahmani A, Chew YL. 2021. Investigating the molecular mechanisms of learning and memory using Caenorhabditis elegans. J Neurochem. 159(3):417–451. doi: 10.1111/jnc.15510.
- Randi F, Leifer AM. 2020. Measuring and modeling whole-brain neural dynamics in Caenorhabditis elegans. Curr Opin Neurobiol. 65:167–175. doi: 10.1016/j.conb.2020.11.001.
- Rapti G. 2020. A perspective on C. elegans neurodevelopment: from early visionaries to a booming neuroscience research. J Neurogenet. 34(3–4):259–272. doi: 10.1080/01677063.2020.1837799.
- Reemst K, Shahin H, Shahar OD. 2023. Learning and memory formation in zebrafish: protein dynamics and molecular tools. Front Cell Dev Biol. 11:1120984. doi: 10.3389/fcell.2023.1120984.
- Rice D, Barone JS. 2000. Critical periods of vulnerability for the developing nervous system: evidence from humans and animal models. Environ Health Perspect. 108:511–533. doi: 10.2307/3454543.
- Rico EP, Rosemberg DB, Seibt KJ, Capiotti KM, Da Silva RS, Bonan CD. 2011. Zebrafish neurotransmitter systems as potential pharmacological and toxicological targets. Neurotoxicol Teratol. 33(6):608–617. doi: 10.1016/j.ntt.2011.07.007.
- Roberts AC, Bill BR, Glanzman DL. 2013. Learning and memory in zebrafish larvae. Front Neural Circuits. 7:126. doi: 10.3389/fncir.2013.00126.
- Rosa JGS, Lima C, Lopes-Ferreira M. 2022. Zebrafish larvae behavior models as a tool for drug screenings and pre-clinical trials: a review. Int J Mol Sci. 23(12):6647. doi: 10.3390/ijms23126647.
- Rosca A, Coronel R, Moreno M, González R, Oniga A, Martín A, López V, González MDC, Liste I. 2020. Impact of environmental neurotoxic: current methods and usefulness of human stem cells. Heliyon. 6(12):e05773. doi: 10.1016/j.heliyon.2020.e05773.
- Rubin LL, Staddon JM. 1999. The cell biology of the blood-brain barrier. Annu Rev Neurosci. 22(1):11–28. (doi: 10.1146/annurev.neuro.22.1.11.
- Ruszkiewicz JA, Pinkas A, Miah MR, Weitz RL, Lawes MJA, Akinyemi AJ, Ijomone OM, Aschner M. 2018. C. elegans as a model in developmental neurotoxicology. Toxicol Appl Pharmacol. 354:126–135. doi: 10.1016/j.taap.2018.03.016.
- Sachana M, Shafer T, Terron A. 2021. Toward a better testing paradigm for developmental neurotoxicity: OECD efforts and regulatory considarations. Biology. 10(2):86. doi: 10.3390/biology10020086.
- Sachana M, Willett A, Pistollato F, Bal-Price A. 2021. The potential of mechanistic information organised within the AOP framework to increase regulatory uptake of the developmental neurotoxicity (DNT) in vitro battery of assays. Reprod Toxicol. 103:159–170. doi: 10.1016/j.reprotox.2021.06.006.
- Saint-Amant L. 2006. Development of motor networks in zebrafish embryos. Zebrafish. 3(2):173–190. doi: 10.1089/zeb.2006.3.173.
- Sammi SR, Foguth RM, Nieves CS, De Perre C, Wipf P, McMurray CT, Lee LS, Cannon JR. 2019. Perfluorooctane sulfonate (PFOS) produces dopaminergic neuropathology in Caenorhabditis elegans. Toxicol Sci. 172(2):417–434. doi: 10.1093/toxsci/kfz191.
- Sandner G, Konig A, Wallner M, Weghuber J. 2021. Alternative model organisms for toxicological fingerprinting of relevant parameters in food and nutrition. Crit Rev Food Sci Nutr. 62(22):5956–5982.
- Sasakura H, Mori I. 2013. Behavioural plasticity, learning, and memory in C. elegans. Curr Opin Neurobiol. 23(1):92–99. doi: 10.1016/j.conb.2012.09.005.
- Schmeisser S, Miccoli A, von Bergen M, Berggren E, Braeuning A, Busch W, Desaintes C, Gourmelon A, Grafström R, Harrill J, et al. 2023. New approach methodologies in human regulatory toxicology - Not if, but how and when! Environ Int. 178:108082. doi: 10.1016/j.envint.2023.108082.
- Selderslaghs IWT, Hooyberghs J, De Coen W, Witters HE. 2010. Locomotor activity in zebrafish embryos: a new method to assess developmental neurotoxicity. Neurotoxicol Teratol. 32(4):460–471. doi: 10.1016/j.ntt.2010.03.002.
- Shaham S. 2015. Glial development and function in the nervous system of Caenorhabditis elegans. Cold Spring Harb Perspect Biol. 7(4):a020578. doi: 10.1101/cshperspect.a020578.
- Shan L, Heusinkveld HJ, Paul KC, Hughes S, Darweesh SKL, Bloem BR, Homberg JR. 2023. Towards improved screening of toxins for Parkinson’s risk. NPJ Parkinsons Dis. 9(1):169. doi: 10.1038/s41531-023-00615-9.
- Shaye DD, Greenwald I. 2011. OrthoList: a compendium of C. elegans genes with human orthologs. PLoS One. 6(5):e20085. doi: 10.1371/journal.pone.0020085.
- Sheets LP, Slikker JW. 2018. Animal/human concordance. In: Slikker Jr. W, Paule MG, Wang C, editors. Handbook of developmental neurotoxicology. 2nd ed. London: Academic Press; p. 527–538.
- Shen Q, Truong L, Simonich MT, Huang C, Tanguay RL, Dong Q. 2020. Rapid well-plate assays for motor and social behaviors in larval zebrafish. Behav Brain Res. 391:112625. doi: 10.1016/j.bbr.2020.112625.
- Shomrat T, Levin M. 2013. An automated training paradigm reveals long-term memory in planarians and its persistence through head regeneration. J Exp Biol. 216(Pt 20):3799–3810. eng.
- Silva M, Pham N, Lewis C, Iyer S, Kwok E, Solomon G, Zeise L. 2015. A comparison of ToxCast test results with in vivo and other in vitro endpoints for neuro, endocrine, and developmental toxicities: a case study using Endosulfan and Methidathion. Birth Defects Res B Dev Reprod Toxicol. 104(2):71–89. doi: 10.1002/bdrb.21140.
- Silva MV. 2020. Effects of low-dose chlorpyrifos on neurobehavior and potential mechanisms: a review of studies in rodents, zebrafish, and Caenorhabditis elegans. Birth Defects Res. 112(6):445–479. doi: 10.1002/bdr2.1661.
- Smirnova L, Hogberg HT, Leist M, Hartung T. 2014. Developmental neurotoxicty - challenges in the 21st century and in vitro opportunities. ALTEX. 31(2):129–156.
- Spinu N, Bal-Price A, Cronin MTD, Enoch SJ, Madden JC, Worth AP. 2019. Development and analysis of an adverse outcome pathway network for human neurotoxicity. Arch Toxicol. 93(10):2759–2772. doi: 10.1007/s00204-019-02551-1.
- Stein GM, Murphy CT. 2012. the intersection of aging, longevity pathways, and learning and memory in C. elegans. Front Genet. 3(259):259. doi: 10.3389/fgene.2012.00259.
- Stewart AM, Kalueff AV. 2012. The developing utility of zebrafish models for cognitive enhancers research. Curr Neuropharmacol. 10(3):263–271.
- Stout R, Verkhratsky A, Parpura V. 2014. Caenorhabditis elegans glia modulate neuronal activity and behavior. Front Cell Neurosci. 8:67. doi: 10.3389/fncel.2014.00067.
- Strähle U, Scholz S, Geisler R, Greiner P, Hollert H, Rastegar S, Schumacher A, Selderslaghs I, Weiss C, Witters H, et al. 2012. Zebrafish embryos as an alternative to animal experiments–a commentary on the definition of the onset of protected life stages in animal welfare regulations. Reprod Toxicol. 33(2):128–132. doi: 10.1016/j.reprotox.2011.06.121.
- Sulston JE, Horvitz HR. 1977. Post-embryonic cell lineages of the nematode, Caenorhabditis elegans. Dev Biol. 56(1):110–156. doi: 10.1016/0012-1606(77)90158-0.
- Sun H, Hobert O. 2023. Temporal transitions in the postembryonic nervous system of the nematode Caenorhabditis elegans: recent insights and open questions. Semin Cell Dev Biol. 142:67–80. doi: 10.1016/j.semcdb.2022.05.029.
- Svara F, Förster D, Kubo F, Januszewski M, Dal Maschio M, Schubert PJ, Kornfeld J, Wanner AA, Laurell E, Denk W, et al. 2022. Automated synapse-level reconstruction of neural circuits in the larval zebrafish brain. Nat Methods. 19(11):1357–1366. doi: 10.1038/s41592-022-01621-0.
- Taylor RW, Hsieh YW, Gamse JT, Chuang CF. 2010. Making a difference together: reciprocal interactions in C. elegans and zebrafish asymmetric neural development. Development. 137(5):681–691. doi: 10.1242/dev.038695.
- Thapar A, Cooper M, Rutter M. 2017. Neurodevelopmental disorders. Lancet Psychiatry. 4(4):339–346. doi: 10.1016/S2215-0366(16)30376-5.
- Tilson HA. 1993. Neurobehavioral methods used in neurotoxicological research. Toxicol Lett. 68(1–2):231–240. doi: 10.1016/0378-4274(93)90134-j.
- Van Damme S, De Fruyt N, Watteyne J, Kenis S, Peymen K, Schoofs L, Beets I. 2021. Neuromodulatory pathways in learning and memory: lessons from invertebrates. J Neuroendocrinol. 33(1):e12911. eng.
- van Thriel C, Westerink RH, Beste C, Bale AS, Lein PJ, Leist M. 2012. Translating neurobehavioural endpoints of developmental neurotoxicity tests into in vitro assays and readouts. Neurotoxicology. 33(4):911–924. doi: 10.1016/j.neuro.2011.10.002.
- Vester A, Caudle WM. 2016. The synapse as a central target for neurodevelopmental susceptibility to pesticides. Toxics. 4(3):18. doi: 10.3390/toxics4030018.
- Vorhees CV, Williams MT, Hawkey AB, Levin ED. 2021. Translating neurobehavioral toxicity across species from zebrafish to rats to humans: implications for risk assessment. Front Toxicol. 3:629229. doi: 10.3389/ftox.2021.629229.
- Walter KM, Dach K, Hayakawa K, Giersiefer S, Heuer H, Lein PJ, Fritsche E. 2019. Ontogenetic expression of thyroid hormone signalling genes: an in vitro and in vivo species comparison. PLoS One. 14(9):e0221230. doi: 10.1371/journal.pone.0221230.
- Wang Y, Gai T, Zhang L, Chen L, Wang S, Ye T, Zhang W. 2023. Neurotoxicity of bisphenol A exposure on Caenorhabditis elegans induced by disturbance of neurotransmitter and oxidative damage. Ecotoxicol Environ Saf. 252:114617. doi: 10.1016/j.ecoenv.2023.114617.
- Wang Y, Liu SS, Huang P, Wang ZJ, Xu YQ. 2021. Assessing the combined toxicity of carbamate mixtures as well as organophosphorus mixtures to Caenorhabditis elegans using the locomotion behaviors as endpoints. Sci Total Environ. 760:143378. doi: 10.1016/j.scitotenv.2020.143378.
- Weiner AMJ, Irijalba I, Gallego MP, Ibarburu I, Sainz L, Goñi-de-Cerio F, Quevedo C, Muriana A. 2024. Validation of a zebrafish developmental defects assay as a qualified alternative test for its regulatory use following the ICH S5(R3) guideline. Reprod Toxicol. 123:108513. doi: 10.1016/j.reprotox.2023.108513.
- Wellenberg A, Weides L, Kurzke J, Hennecke T, Bornhorst J, Crone B, Karst U, Brinkmann V, Fritz G, Honnen S. 2021. Use of C. elegans as a 3R-compliant in vivo model for the chemoprevention of cisplatin-induced neurotoxicity. Exp Neurol. 341:113705. doi: 10.1016/j.expneurol.2021.113705.
- White JG, Southgate E, Thomson JN, Brenner S. 1986. The structure of the nervous system of the nematode Caenorhabditis elegans. Philos Trans R Soc London B. 314:1–340.
- Wilson SW, Ross LS, Parrett T, S.s EJ. 1990. The development of a simple scaffold of axon tracts in the brain of the embryonic zebrafish, Brachydanio rerio. Development. 108(1):121–145. doi: 10.1242/dev.108.1.121.
- Wittkowski P, Marx-Stoelting P, Violet N, Fetz V, Schwarz F, Oelgeschläger M, Schönfelder G, Vogl S. 2019. Caenorhabditis elegans as a promising alternative model for environmental chemical mixture effect assessment—a comparative study. Environ Sci Technol. 53(21):12725–12733. doi: 10.1021/acs.est.9b03266.
- Witvliet D, Mulcahy B, Mitchell JK, Meirovitch Y, Berger DR, Wu Y, Liu Y, Koh WX, Parvathala R, Holmyard D, et al. 2021. Connectomes across development reveal principles of brain maturation. Nature. 596(7871):257–261. doi: 10.1038/s41586-021-03778-8.
- Wu Y, Ghitani A, Christensen R, Santella A, Du Z, Rondeau G, Bao Z, Colón-Ramos D, Shroff H. 2011. Inverted selective plane illumination microscopy (iSPIM) enables coupled cell identity lineaging and neurodevelopmental imaging in Caenorhabditis elegans. Proc Natl Acad Sci U S A. 108(43):17708–17713. doi: 10.1073/pnas.1108494108.
- Yan D, Zhang Y, Liu L, Yan H. 2016. Pesticide exposure and risk of Alzheimer’s disease: a systematic review and meta-analysis. Sci Rep. 6(1):32222. doi: 10.1038/srep32222.
- Yoshimura S, Murray JI, Lu Y, Waterston RH, Shaham S. 2008. mls-2 and vab-3 control glia development, hlh-17/Olig expression and glia-dependent neurite extension in C. elegans. Development. 135(13):2263–2275. doi: 10.1242/dev.019547.
- Zhong X, Harris G, Smirnova L, Zufferey V, Sá RdCdSE, Baldino Russo F, Baleeiro Beltrao Braga PC, Chesnut M, Zurich M-G, Hogberg HT, et al. 2020. Antidepressant paroxetine exerts developmental neurotoxicity in an iPSC-derived 3D human brain model. Front Cell Neurosci. 14:25. doi: 10.3389/fncel.2020.00025.
- Zhou R, Yu Y, Zhang W, Wang D, Bai Y, Wang Y, Bu Y. 2022. Sensory disturbance by six insecticides in the range of μg/L in Caenorhabditis elegans. Front Environ Sci. 10:859356. doi: 10.3389/fenvs.2022.859356.