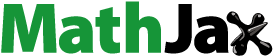
Abstract
In the risk assessment of agrochemicals, there has been a historical paucity of using data to refine the default adjustment factors, even though large datasets are available to support this. The current state of the science for addressing uncertainty regarding animal to human extrapolation (AFA) is to develop a “data-derived” adjustment factor (DDEF) to quantify such differences, if data are available. Toxicokinetic (TK) and toxicodynamic (TD) differences between species can be utilized for the DDEF, with human datasets being ideal yet rare. We identified a case for a currently registered herbicide, mesotrione, in which human TK and TD are available. This case study outlines an approach for the development of DDEFs using comparative human and animal data and based on an adverse outcome pathway (AOP) for inhibition of 4-hydroxyphenol pyruvate dioxygenase (HHPD). The calculated DDEF for rat to human extrapolation (AFA) for kinetics (AFAK = 2.5) was multiplied by the AFA for dynamics (AFAD = 0.3) resulting in a composite DDEF of ∼1 (AFA = 0.75). This reflects the AOP and available scientific evidence that humans are less sensitive than rats to the effects of HPPD inhibitors. Further analyses were conducted utilizing in vitro datasets from hepatocytes and liver cytosols and extrapolated to whole animal using in vitro to in vivo extrapolation (IVIVE) to support toxicodynamic extrapolation. The in vitro datasets resulted in the same AFAD as derived for in vivo data (AFAD = 0.3). These analyses demonstrate that a majority of the species differences are related to toxicodynamics. Future work with additional in vitro/in vivo datasets for other HPPD inhibitors and cell types will further support this result. This work demonstrates utilization of all available toxicokinetic and toxicodynamic data to replace default uncertainty factors for agrochemical human health risk assessment.
1. Introduction
Human health risk assessments based on data packages for agrochemical regulatory submission encompass comparably large databases on chemical toxicity. Risk assessments, as currently conducted for agrochemicals, utilize well established methodologies such as derivation of a reference dose (RfD), using an animal-based point of departure (POD) for the most sensitive critical effect, divided by factors to address data uncertainties and variability. The resulting RfD is considered an estimate of a subthreshold dose below which no appreciable deleterious risks are likely, and which can be later compared to chemical exposure to ascertain potential for hazard in exposed human populations (US EPA Citation2002).
Standard approaches for consideration of uncertainty and variability in RfDs have been well documented (US EPA Citation2002), and the idea of the composite adjustment factor (AF) is to account for the total unknowns or uncertainties related to the dataset and hazard outcomes. While default values of 10-fold have been recommended to address variations between species and between humans and validated as conservative in many cases (Dourson ML and Stara Citation1983; Calabrese and Gilbert Citation1993; Renwick and Lazarus Citation1998), these default values were set using approaches reflecting the scientific understanding of the time, and were not originally intended for blanket use (Lehman and Fitzhugh Citation1954; WHO Citation1958; Martin et al. Citation2013). Instead, the more modern recommendation has been to use available data to refine these uncertainties, thereby replacing default values of 10-fold with appropriate data (IPCS Citation2005; US EPA Citation2014). In this way, use of data to refine the default value is not explicitly more or less conservative but allows for generation of a more precise quantitative estimation of species differences and variabilities.
In the registration of agrochemicals, there has been a historical paucity of using data to refine the default adjustment factors, even though large datasets are available to support this. In these risk assessments, the most common AFs applied have been AFH (interhuman variability: intended to account for variability in response across exposed human populations) and AFA (interspecies extrapolation from experimental animal to human: to account for species differences when using animal-based PODs for human health risk assessment). Both the AFH and the AFA have a default value of 10-fold, resulting in application of a “standard” default composite adjustment factor of 100-fold.
The basic assumptions for the AFA are that the results seen in experimental animals are relevant to humans, that toxicokinetic (TK) and toxicodynamic (TD) differences exist between species, and that humans are more sensitive than animals at a given mg/kg-day dose (Pecquet and Haber Citation2023). The current state of the science for the two adjustment factors addressing variability (AFH) and uncertainty (AFA) is to develop a “data-derived” (also called “chemical-specific”) adjustment factor to quantify such differences if data are available. Renwick (Citation1991) initially proposed subdividing each of these AFs to account for differences in TK and TD, suggesting 3.16 each (100.5) for kinetic and dynamic differences. Later, Renwick (Citation1993) reported that kinetic differences between species are generally greater than dynamic differences between species, thus revising into factors of 4 for kinetics (AFAK) and 2.5 for dynamics (AFAD), as illustrated for the composite AFA in (Pecquet and Haber Citation2023).
Figure 1. Subdivision of AFA for the development of CSAFs/DDEFs. The composite adjustment factor for animal to human extrapolation (AFA) is divided into a factor for kinetics (AFAK) and dynamics (AFAD), which are combined in the derivation of a chemical specific adjustment factor (CSAF) or data derived extrapolation factor (DDEF). In this way, data for kinetics and dynamics can be incorporated individually based on available data. There are slight regulatory differences in this subdivision, where the International Programme for Chemical Safety (IPCS) utilizes uneven subdivision (kinetics = 4, dynamics = 2.5) and the US Environmental Protection Agency (US EPA) utilizes even subdivision of the defaults to 3.16, while allowing for utilization of a human equivalent dose (HED) for kinetics. CSAF: chemical specific adjustment factor; DDEF: data derived extrapolation factor; HEC: human equivalent concentration; HED: human equivalent dose.
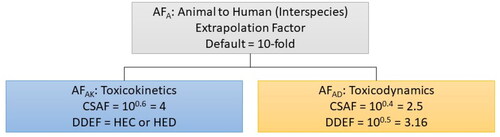
The International Programme on Chemical Safety (IPCS) adopted the Renwick (Citation1991, Citation1993) proposal in their guidance for chemical specific adjustment factors (CSAF) (IPCS Citation2005). The US EPA (Citation2014) adopted a similar approach to IPCS but termed them as data derived extrapolation factors (DDEFs) and recommended that (in the absence of chemical specific data) the TD portion remain at 3.16 while the TK portion could be accounted for by using allometric scaling to derive a human equivalent dose (HED). Both guidances advocate the replacement of 10-fold defaults with chemical-specific data when available. In global agrochemical risk assessment, the European Food Safety Authority (EFSA 2012), the US EPA Pesticide Program (US EPA Citation2015b), and the Canadian Pest Management Regulatory Agency (PMRA Citation2008, Citation2021) describe the use of data to refine the AFA when possible. Although each of these organizations mention the option to move away from defaults using data, the specific information needed to do so are ill defined, and as such, it can be difficult to determine the sufficiency of evidence needed across various agencies.
As has been stated in the literature, as well as in the regulatory guidance, use of chemical-specific data to refine uncertainty should be the “gold standard” for risk assessment (Dourson et al. Citation2013; Terry et al. Citation2016; Bhat et al. Citation2017). For example, Bhat et al. (Citation2017) noted that a reason for the underuse of CSAFs related to low regulatory acceptance of replacing default values, under the assumption that the default approaches were more conservative. This is an inappropriate assumption, as default approaches have higher uncertainty than data-derived approaches, meaning there is less confidence when using default values (Bhat et al. Citation2017).
As laid out in guidance for the development of CSAFs (IPCS Citation2005) and DDEFs (US EPA Citation2014), key considerations to replace defaults include identifying an adverse biological event associated with that adverse health outcome, and the concentration of the toxicant associated with the development of the biological event. Given the advancements in understanding mode of action (MOA) in the form of adverse outcome pathways (AOPs), and the generation of absorption, distribution, metabolism, and excretion (ADME) information for a standard data submission package, there are generally data available to refine at least a portion of the default adjustment factors.
This case study outlines an approach for the development of DDEFs for a currently registered herbicide, mesotrione, using comparative TK and TD data and based on an AOP of inhibition of 4-hydroxyphenol pyruvate dioxygenase (HPPD). We used available in vivo human and rat TK data to describe kinetics based on species differences in mesotrione plasma concentration, while we used human and rat in vivo TD data to describe dynamics based on species differences in tyrosine plasma concentration. This resulted in a composite AFA <1, which aligns with the documented decreased sensitivity of humans compared to rats for HPPD inhibition. We subsequently identified high concordance between the in vivo TD and in vitro TD datasets when comparing across HPPD inhibitors, supporting the derived AFAD value.
2. Case study to derive a composite AFA using mesotrione human data
2.1. Adverse outcome pathway (AOP) for 4-hydroxyphenol pyruvate dioxygenase (HPPD) inhibitors
The HPPD AOP has been heavily discussed in the published literature as well as governmental reports (Lock et al. Citation1996; Lewis and Botham Citation2013; US EPA Citation2015a, Citation2020b; Botham et al. Citation2023) (). In brief, mammalian HPPD inhibition blocks tyrosine catabolism resulting in an increase in systemic tyrosine levels (Hall et al. Citation2001). Normal tyrosine catabolism proceeds via the tyrosine aminotransferase (TAT) enzyme, which is the rate limiting catabolic step, resulting in formation of the metabolite 4-hydroxyphenylpyruvic acid (HPPA). HPPA is either excreted or further catabolized via HPPD to homogentisic acid or to other metabolic substrates, such as 4-hydroxyphenyllactate (HPLA) (US EPA Citation2020b). In the developing AOP, the molecular initiating event (MIE) is chemical binding to and inhibiting HPPD, and resulting in the buildup of HPPA and upstream elevation of plasma tyrosine (tyrosinemia) (US EPA Citation2020b; AOP Wiki Citation2024: https://aopwiki.org/aops/114). Elevation in plasma tyrosine has been associated with an adverse outcome (AO) for ocular effects (). One method for tyrosine clearance upon HPPD inhibition involves TAT-mediated tyrosine catabolism to HPPA, followed by urinary excretion of HPPA and/or HPLA (US EPA Citation2020b). Therefore, species specific differences in TAT activity and the urinary excretion of metabolites could result in differential sensitivity to HPPD inhibition and the development of tyrosinemia (US EPA Citation2020b). There are several registered agrochemicals that are demonstrated HPPD inhibitors, including mesotrione, bicyclopyrone, isoxaflutole, pyrasulfone, tembotrion, and tolpyralate, among others, which have been evaluated in a cumulative risk assessment by the US EPA (Citation2021). Both ocular effects (corneal abnormalities, opaque/cloudy eyes, ocular lesions) and developmental effects (decreased ossification, decreased pup body weight) were correlated with increased tyrosine in response to HPPD inhibition (US EPA Citation2021). However, developmental effects were demonstrated at higher doses (≥600 mg/kg-day) than ocular effects (>300 mg/kg-day) in the critical species. Therefore, the US EPA determined that chronic ocular toxicity was the most appropriate measure of toxicity to utilize in the cumulative risk assessment (US EPA Citation2021), and this is the focus of the adverse outcome utilized in the current assessment.
Figure 2. Tyrosine catabolic pathway in mammals (adapted from US EPA Citation2020b). Normal tyrosine catabolism involves the conversion to HPPA by the TAT enzyme. HPPA can either be further metabolized and/or excreted or be converted to homogentisic acid for further metabolism via the HPPD enzyme.
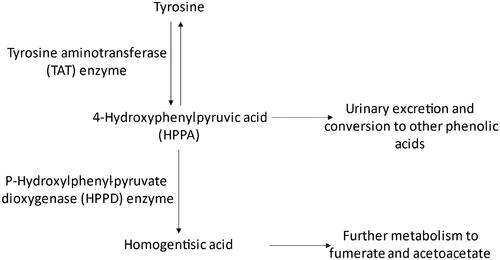
Figure 3. The AOP for HPPD inhibition leading to ocular effects as compared between sensitive (rat, dog) and insensitive (mouse, human) species. Differences in TAT activity in the less sensitive species results in higher clearance of plasma tyrosine which prevents downstream ocular effects. Each step in the AOP was assigned to either account for a toxicokinetic or toxicodynamic dose metric for use in refining uncertainties in the risk assessment. AOP: adverse outcome pathway; HPPD: 4-hydroxyphenol pyruvate dioxygenase; TAT: tyrosine aminotransferase; MIE: molecular initiating event; KE: key event; AO: adverse outcome; HPPA: 4-hydroxyphenylpyruvic acid.
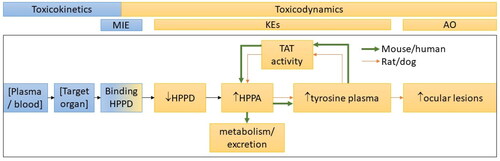
The most strongly associated and most sensitive adverse outcome resulting from tyrosinemia is ocular effects across all species tested, including humans (Rich et al. Citation1973; Burns et al. Citation1976; Lock et al. Citation1996; Lewis and Botham Citation2013; US EPA Citation2020b). Non-clinical data showing an association between high levels of plasma tyrosine and ocular effects exhibited a clear species-specific threshold. Humans are particularly insensitive to HPPD inhibition, as shown in clinical data for the synthetic HPPD inhibitor nitisinone [brand name ORFADIN®, NTBC, 2-(4-methylsulfonyl-2-nitrobenzoyl)-1,2-cyclohexanedione]. Nitisinone is marketed and approved as a human therapeutic for the treatment of hereditary type 1 tyrosinemia and acts by inhibiting the normal catabolism of tyrosine and thereby prevents the accumulation of downstream toxic catabolic intermediates (US FDA Citation2002). Based on clinical and non-clinical data, a plasma tyrosine threshold level of 1000 µmol/L for the development of ocular lesions was determined (US EPA Citation2020b). Clinically, plasma tyrosine in patient populations is controlled to <500 μmol/L, and a health protective POD has been established by the United States Food and Drug Administration (US FDA) for tyrosine-related adverse effects in humans at that threshold (Ward et al. Citation2017). Furthermore, species differences indicate the mouse and human as the most similar species regarding tyrosine response to HPPD inhibition, as the maximum level of serum tyrosine following complete inhibition of HPPD was higher in rat (2673 nmol/mL), dog (1814 nmol/mL), and rabbit (1480 nmol/mL), as compared to mouse (1154 nmol/mL) and human (1192 nmol/mL) (Botham et al. Citation2023).
The TAT enzyme-mediated tyrosine elimination pathway has a substantial impact on plasma tyrosine levels upon HPPD inhibition, and it has been demonstrated that TAT activity is increased in humans upon HPPD inhibition. As HPPD is inhibited, the levels of metabolites HPPA and HPLA in humans increase, resulting from increased TAT-mediated conversion of tyrosine (US EPA Citation2020b). This increased human TAT activity was demonstrated by comparing HPPA formation upon treatment of pooled liver cytosols across species treated with nitisinone (Botham et al. Citation2023). Additionally, in response to nitisinone, species differences in HPLA generation were higher for human hepatocytes (9-fold), as compared to the mouse (5-fold), and rat (1.9-fold), again suggesting increased TAT activity in humans aids in tyrosine clearance (US EPA Citation2020b). Supporting data in the LiverbeadTM assay found similar HPLA levels in mice and human cells following 4-h exposure to nitisinone, with levels in the rat being below the level of quantification (US EPA Citation2020b).
Therefore, the overall toxicology database demonstrates that humans are less sensitive than rat animal models to the effects of HPPD inhibitors (Lock et al. Citation2000; Lewis and Botham Citation2013; JMPR Citation2014; US EPA Citation2015a, Citation2020b; Botham et al. Citation2023). Specifically, species differences related to TAT enzyme activity suggest humans would not achieve sustained plasma tyrosine levels high enough for ocular or other adverse effects to occur, when exposure is estimated based on use scenarios for agrochemical HPPD inhibitors (US EPA Citation2015a). A human study is available that measures plasma mesotrione and plasma tyrosine following oral exposure (Hall et al. Citation2001). Additionally, data exist on these same metrics in the rat from unpublished studies conducted during data generation for regulatory submission by the registrant (see Supplemental Appendix A). As there are sufficient data demonstrating species specific responses to HPPD inhibition, and there are TK and TD data available for rats and humans, the AFA can be refined by derivation of a DDEF.
2.2. Choice of TK and TD dose metric to define a DDEF for mesotrione
The US EPA (Citation2014) guidance for DDEFs discusses the choice of a comparative metric for refining the species extrapolation factor (AFA). The TK metric should consider what the body does to the chemical (absorption, distribution to the target organ, metabolism, elimination, etc.), while the TD metric considers what the chemical does to the body (receptor binding, enzyme inhibition/activation, effects leading to adversity, etc.). The guidance notes that there is a continuum from TK through TD, whereby data that “incorporate intracellular processing related to the mode of action, such as enzyme inhibition, will reflect the uptake and delivery (kinetics) and at least part of the dynamics” (US EPA Citation2014). Therefore, we overlaid the AOP pathway with potential TK and TD responses that could be used in a DDEF (). This also helps to demonstrate the steps in the AOP at which humans and mice deviate from rats and dogs resulting in decreased sensitivity to HPPD inhibition (). From this exercise and based on the available human and rat datasets, the only available TK dose metric for mesotrione was concentration in the blood, measured by area under the time concentration curve (AUC) or maximum plasma concentration (Cmax) (Hall et al. Citation2001).
It should be noted that binding to HPPD exists on the continuum between TK and TD processes and might reflect a portion of both kinetics and dynamics. Based on the availability of in vivo human data following mesotrione exposure, options for dose metrics for TK include plasma mesotrione (Hall et al. Citation2001), while TD dose metrics include tyrosine plasma elevation (Botham et al. Citation2023).
2.2.1. Toxicokinetic dose metric
The goal of incorporating TK data between species is to define the differences in an equivalent internal dose metric based on the external dose. Ideally, the dose metric at the POD in animals would be used to determine the ratio of the external dose that would result in the same internal concentration in animals and humans, or, as the ratio of internal concentrations resulting from the same external dose (US EPA Citation2014). The available human and rat data for mesotrione kinetics are the AUC (measure of total systemic exposure) and Cmax (maximum plasma concentration) as shown in (Hall et al. Citation2001; for the raw rat mesotrione TK/TD dataset, see Supplemental Appendix A). In Hall et al. (Citation2001), three groups of six male human volunteers were exposed to a single oral capsule containing mesotrione at 0.1, 0.5, or 4 mg/kg. To ensure a consistent intake of tyrosine, volunteers were fed a controlled diet from 72 h pre-dosing through 96-h post-dosing (JMPR Citation2014). Plasma and urine samples were collected and analyzed for tyrosine and mesotrione. According to the DDEF guidance, the choice of internal dose metric for mesotrione should be the AUC, as effects related to subchronic or chronic exposure are normally related to AUC. When insufficient data are available to support a choice between Cmax and AUC, the AUC is recommended as it is a more protective measure likely reflecting greatest interspecies differences (IPCS Citation2005; US EPA Citation2014). Additionally, the AUC incorporates the entire exposure duration and time-normalized total dose. Therefore, differences in half-life are accounted for, supporting the AUC as the most appropriate dose metric.
Table 1. Available oral kinetic data for human and rat exposed to mesotrione.
While the most suitable AUC would be taken from a dose interval at which steady state has been achieved, there are no repeat dose TK studies available in humans. In repeat dose studies, steady state concentrations are achieved when the duration of exposure exceeds ∼5 elimination half-lives and the compound exhibits linear kinetics (Reichard et al. Citation2016). Therefore, if the T½ x 5 < 24 h, we can expect similar steady state kinetics from the single dose study as compared to a repeat dose study. This is because, given the short half-life of mesotrione, the compound would be systemically eliminated prior a repeat dose, meaning the acute duration study would be representative of the repeat dose scenario. To evaluate the use of an acute dose AUC(0-∞), we compared the half-life of mesotrione in humans and rats. At 0.5 mg/kg, the half-life (T½) in humans was 1.3 h and in rats was 1.6 h. In humans, 1.3 h x 5 = 6.5 h, and in rats 1.6 h x 5 = 8 h. Therefore, we anticipate that the kinetics of the single dose acute study are representative of the kinetics in a repeat dose study scenario. Final support for the position of no accumulation can be seen by deriving an accumulation ratio (AR) using EquationEquation (1)(1)
(1) .
(1)
(1)
Where: kel = ln(2)/T½ and tau = 24h (the interval between repeat doses). The AR is equal to 1 in both humans and rats (calculation for humans shown in EquationEquation (1)(2)
(2) ), meaning no accumulation is anticipated with repeat daily dosing. These calculations are supported by US EPA and JMPR (Joint FAO/WHO Meeting on Pesticide Residues) conclusions suggesting there is no potential for mesotrione accumulation (JMPR Citation2014; US EPA Citation2015a, Citation2020b). These data combined support the position that accumulation with multiple daily dosing is not expected, as the compound would be sufficiently eliminated prior to the next dose at the range of human exposures.
It is worth noting that the data in humans comes from a study using oral capsule exposure, while the data in experimental animals comes from a study using oral gavage exposure. While these routes are both considered oral, there are different kinetics between gavage (bolus) dosing and oral capsule. We do not anticipate these differences to substantially impact the results and note that gavage dosing in animal studies is typically utilized because the dose is controlled and more easily quantified as compared to dietary exposure. Additionally, after oral administration to humans, mesotrione AUC and Cmax (as well as tyrosine AUC and Cmax) correlated linearly with dose (Hall et al. Citation2001). Only two data points were available for orally dosed rats, but over the dose range considered (0.5 and 2 mg/kg), the rat toxicokinetics also look to be linear.
We selected the dose of 0.5 mg/kg to determine the AFAK for rat to human for mesotrione. This is because there are data in both animals and humans at this dose and it is the lowest dose available for the rat and therefore closest to a potential POD [as is recommended in the guidance (US EPA)]. Furthermore, the response metric can be directly compared at the same external dose at a roughly similar magnitude. The AFAK can then be calculated using EquationEquation (2)(2)
(2) :
(2)
(2)
Where: AUCH is the mesotrione AUC in humans and AUCA is the mesotrione AUC in animals.
Therefore, the resulting AFAK is 2.5 and can be used to replace the default AFAK for risk assessments based on a rat POD for mesotrione.
Support for this AFAK value comes from a systematic evaluation of all potential AFAKs derived for dose-normalized AUC values (Table S1). When dose normalized and compared across the range of rat and human AUC values, the derived AFAK ranges from 1.8 − 2.9 (mean 2.4) (Table S1). This indicates that the data are in the linear range as the mesotrione AFAK does not change with dose and the slope of the dose-AUC line between rats and humans is expected to be similar.
2.2.2. Toxicodynamic dose metric using tyrosine plasma concentration
In contrast to TK which aims to quantify differences in internal dosimetry, TD aims to quantify differences in concentration producing the same level of dynamics (toxicologic response) between animals and humans (US EPA Citation2014). As shown in , choices for the TD metric (key events) for HPPD inhibition include binding to/inhibition of HPPD enzyme, TAT enzyme activity, HPPA/HPLA formation, and plasma tyrosine. Each could be a suitable metric to account for species differences in TD based on the AOP. Ideally, measures of TD parameters at the target organ should be utilized; however, in the absence of liver-specific data, plasma concentration and/or urinary excretion profiles could be useful surrogates.
The choice of TD dose metric depends not only on an understanding of the AOP, but also on the type of data available, and as such, the only available in vivo TD data in humans are measures of plasma tyrosine (). As the most sensitive critical effect across HPPD inhibitors is ocular effects in response to elevated plasma tyrosine, and the mechanistic theory suggests differences in this metric are directly correlated to species sensitivity, comparative analysis of plasma tyrosine is appropriate to account for TD species differences. Typically, when the most appropriate choice is unclear, the dose metric should be the AUC, as it is a more protective measure likely reflecting greatest interspecies differences as compared to Cmax (IPCS Citation2005; US EPA Citation2014).
Table 2. Available toxicodynamic plasma tyrosine data for human and rat exposed orally to mesotrione.
Since the kinetic adjustment (AFAK) above accounts for the differences in internal dose and mesotrione kinetics, the AFAD can be derived using tyrosine plasma levels in humans as compared to rat at the same external dose (EquationEquation (3)(3)
(3) ).
(3)
(3)
Where: tyrosine AUCH is the human tyrosine response and tyrosine AUCA is the rat tyrosine response.
Ratios for plasma tyrosine AUC were calculated at the 0.5 mg/kg dose level for rat and humans, since this dose provides the most similar response levels of increased plasma tyrosine, is close to a potential POD in animals, and reflects the dose used to calculate the AFAK. The resulting AFAD is 0.3.
A secondary approach would be to conduct a similar analysis but using the ratio of Cmax instead of AUC (). While the appropriate dose metric has not been explicitly defined, there is some basis to justify a Cmax approach for TD effects, given there is a biological threshold associated with ocular effects from tyrosine levels. Therefore, the AFAD was also derived using Cmax and compared to the AFAD derived using AUC. Again, using the 0.5 mg/kg dose level, the AFAD based on Cmax was 0.3 (175 nmol/mL ÷ 508 nmol/mL) between human and rat. This rat to human AFAD is identical to the AFAD derived using the AUC values and increases confidence that selection of a different dose metric would not change the resulting DDEF. However, it is worth noting that conducting a full comparison of the AFAD values following dose-normalization of tyrosine Cmax and AUC results in deviations of the DDEF across dose (Table S2). This indicates that the tyrosine response could be dose dependent, and that there are possible differences in the slope of the tyrosine dose-response between rats and humans, as supported by the literature (Lewis and Botham Citation2013). This also aligns with the known species differences and supports use of the AFAK derived at the same external dose (0.5 mg/kg) for either AUC or Cmax. This choice is further supported in that both AFADs derived at this dose for Cmax and AUC are the same (0.3).
In summary, these data show a reduction in toxicodynamic sensitivity of humans compared to rats (lowering of the AFAD from 3-fold to below 1-fold) and demonstrate the same external dose of mesotrione in the rat is associated with a higher tyrosine elevation than in humans. This aligns well with the known AOP and current understanding of species differences.
2.2.3. Composite DDEF for mesotrione for interspecies extrapolation from rat to human
As discussed above (), the composite AFA results from the combined AFAK and AFAD subfactors. The default value of AFA is 10-fold, which includes either 3-fold (or a derived HED) for kinetics and 3-fold for dynamics. Replacing these default values with the DDEF results in a composite AFA of 0.75 for extrapolation from rat to human for mesotrione (EquationEquation (4)(4)
(4) ).
(4)
(4)
2.3. In vitro AFAD using TAT activity in a chemical agnostic approach
Additional analyses were conducted as an alternative comparative measure for AFAD based on in vitro TAT activity (measured as HPPA formation). In theory, under complete HPPD inhibition, this TD response is expected to be chemical agnostic as the biological response at complete inhibition should not depend on the chemical, only on the biology. Therefore, an AFAD derived from data measuring innate TAT activity would be applicable to all HPPD inhibitors under scenarios of complete inhibition.
In a recent publication, pooled cryopreserved primary hepatocytes from multiple species (including rat and human) were incubated with either vehicle control or nitisinone at 100 µM (to completely inhibit HPPD activity) for 4 h, supplemented with 100 mg/mL tyrosine (Botham et al. Citation2023). TAT activity was calculated from a surrogate measurement of HPPA formation using cell lysate (). HPPA formation increased significantly as compared to vehicle control for all species following nitisinone treatment. Additionally, the level of HPPA was higher in the human cells as compared to the rat, reflecting increased TAT activity. In the recent US EPA document on HPPD inhibitors, data were reported for HPPA levels following nitisinone (100 µM) treatment in hepatocytes from human and rat (). Utilizing both of these datasets, and applying appropriate scaling factors for in vitro to in vivo extrapolation (IVIVE), TAT activity was scaled from a cell based/mg protein level to a whole liver (per kg) and compared across species (namely 80 mg/g cytosolic protein and 24 g liver/kg in adult humans; in a rat, the liver weight is 40 g/kg with 89 mg/g cytosolic protein) () (Gibbs et al. Citation1998; Musther et al. Citation2017; Peters Citation2021).
Table 3. HPPA measured as a metric for TAT activity in hepatocytes across species adjusted for liver size.
An additional dataset in Botham et al. (Citation2023) examined HPPA production in pooled liver cytosols across species provided with excess TAT substrates (tyrosine, pyridoxal phosphate, and oxoglutarate) (). Data reported in reflect the averaged replicate pooled HPPA concentrations for each species. Using the same approach outlined above for hepatocytes, these averaged HPPA concentrations were IVIVE adjusted for liver cytosolic protein and scaled to whole liver for each species.
Table 4. HPPA measured as a metric for TAT activity in pooled liver cytosols across species adjusted for liver size (Botham et al. Citation2023).
The AFAD is calculated as the ratio of the IVIVE scaled HPPA concentration (or rate of formation) between species (EquationEquation (5)(5)
(5) ).
(5)
(5)
Where: HPPAA is the measured animal HPPA metric and HHPAH is the measured human HPPA metric.
In deriving an AFAD based on a surrogate measure of TAT activity, the ratio is comparing the rat activity to the human activity (animal metric divided by human metric). This is the inverse of the above equations using plasma tyrosine, as TAT activity can be interpreted as a measure of clearance, in that, as TAT activity increases (and HPPA is formed), tyrosine plasma decreases. As per the guidance, when using a measure of clearance to derive the AFAD, the inverse equation should be used (US EPA Citation2014). Using the hepatocyte data from either the Botham et al. (Citation2023) or the US EPA (Citation2020b) data gave similar ratios, 0.31 and 0.32 respectively, using EquationEquation (5)(5)
(5) (). Using the pooled cytosol data, the AFAD for rat to human was 0.32-fold, which is aligned with the hepatocyte data (). Similar available datasets align with the derived in vitro AFADs. The derived ratio of HPLA formation between rat and human hepatocytes dosed with the HPPD inhibitor tembotrione in the Liverbead™ assay was 0.21 [0.18 (μg/mg protein)/0.84 (μg/mg protein)] (data as reported in US EPA Citation2020b). Additional data from the same Liverbead™ assay but using the HPPD inhibitor nitisinone yielded the same ratio of 0.21 [0.23 (μg/mg protein)/1.08 (μg/mg protein)] (data as reported in US EPA Citation2020b). It should be noted that these values from the Liverbead assay were not scaled for IVIVE and reflect HPLA not HPPA, yet still demonstrate a similar range of AFAD.
Table 5. Derived rat AFADs across different HPPD inhibitors or TAT activators using in vitro data.
The values calculated for AFAD in are highly aligned with each other and again demonstrate the much lower sensitivity of the human compared to the rat. The TAT-based in vitro AFAD of 0.3 is also identical to the in vivo tyrosine AUC/Cmax AFAD for mesotrione (also 0.3) (). This supports the conclusion that both metrics (plasma tyrosine and TAT activity) are accurately reflecting the AOP and the differences across species, as the relationship between these parameters should be similar. Taken together, these data indicate that the in vitro data accurately reflect the available in vivo data, and vice versa. This, in turn, supports the hypothesis that the toxicodynamic species differences are in fact chemically agnostic under complete or near complete HPPD inhibition, as the mesotrione-specific AFAD based on plasma tyrosine ratios was identical to the AFADs derived for nitisinone and excess tyrosine. These data could thus support extrapolation and use of an AFAD of 0.3 across all HPPD inhibiting compounds; however, it is worth noting the non-linear TD response. The early linear response results from the build-up of HPPA causing elevated systemic tyrosine levels, which increase in a dose-dependent manner until the HPPD enzyme in completely inhibited. Once fully inhibited, tyrosine settles to a level governed by a balance between dietary intake and the species ability to clear HPPA. As such, tyrosine is no longer similarly dose-responsive, given that additional exposure to HPPD inhibitors does not appreciably increase plasma tyrosine. A good depiction of this nonlinear tyrosine response can be seen in US EPA (Citation2020b, in Figure 3.4.3.1), which shows a dose-response of plasma tyrosine against the herbicide mesotrione. Therefore, caution is recommended when extrapolating from sub-threshold exposures, that would not be expected to fully inhibit HPPD. Additional modeling to identify an internal dose associated with complete inhibition of HPPD in vivo would be supportive of the chemical agnostic AFAD hypothesis.
3. Discussion
The use of CSAFs/DDEFs has been scientifically justified and applied in many chemical risk assessments over the years by several State and Federal regulatory agencies [e.g. US EPA, Texas Commission for Environmental Quality (TCEQ), Health Canada, and Minnesota Department of Health (MDH)] (Bhat et al. Citation2017). A majority of these are hazard assessments conducted for well-studied legacy compounds to derive health-based risk values for programs such as the Toxic Substances Control Act (TSCA), the Integrated Risk Information System (IRIS), Priority Substances Lists, Health Criteria Documents, Drinking Water Health Advisories, or Water Quality Criteria/Limits, among others (Bhat et al. Citation2017). While these approaches are utilized less commonly in pesticide risk assessment, some examples do exist such as the Sulfoxaflor Registration Decision (PMRA Citation2016) and the Simazine Registration Review (Terry et al. Citation2016; Bhat et al. Citation2017; US EPA Citation2018). In many cases, it is superfluous to apply data-derived approaches in pesticide risk assessments, firstly due to the “fit-for-purpose” nature of pesticide risk assessment, which relies on a tiered screening level approach in which worst case default data are used until a more refined assessment is triggered, at which point default data are refined using empirical data. This aligns with the necessary level of precision needed to be protective of human health. However, utilizing data-derived approaches by the registrant and in consultation with the regulator could be valuable in the context of proactive approaches or cumulative risk assessments. Secondarily, difficulties in implementation, such as a lack of reliable data (especially TD data), lack of data in humans, and regulatory concerns for acceptance result in their use being less common (Bhat et al. Citation2017). Recently evolving risk assessment paradigms advocate for the use of more predictive technologies and modeling approaches. Thus several recent assessments have applied advanced risk assessment concepts in a proactive nature in order to provide a better understanding of the biology behind agrochemical activity as well as a better characterization of uncertainty and variability (PMRA Citation2016; US EPA Citation2018, Citation2020a).
The AOP for HPPD inhibitors is well understood. Based on clinical and experimental evidence of the effects of HPPD inhibition, humans are known to be less sensitive than toxicity test animal species, and in particular, less sensitive than rat. Therefore, we calculated a DDEF for rat to human extrapolation for the HPPD inhibitor mesotrione using available in vivo human toxicokinetic and toxicodynamic data and based on a defined AOP. The rat to human extrapolation AFA for kinetics (AFAK = 2.5) was based on comparative mesotrione kinetics and multiplied by the AFA for dynamics (AFAD = 0.3) based on comparative tyrosine dynamics, resulting in a composite DDEF of ∼1 (AFA = 0.75). This reflects the understanding and available scientific evidence that humans are much less sensitive than rats to the effects of HPPD inhibitors, on the basis of TD differences (Lock et al. Citation2000; Lewis and Botham Citation2013; JMPR Citation2014; US EPA Citation2015a, Citation2020b; Botham et al. Citation2023). Further analyses were conducted utilizing in vitro datasets measuring HPPA production from rat and human hepatocytes and liver cytosols dosed with either nitisinone or excess TAT substrates. Utilizing HPPA production as a surrogate for TAT-activity, and after conducting IVIVE, the comparative TD ratios between rat and human cells indicated that the dynamic difference between species results in a similar AFAD as derived for in vivo mesotrione data, regardless of method for HPPD inhibition (AFAD = 0.3). This generates the hypothesis that for any compounds causing complete inhibition of HPPD, an AFAD of 0.3 may apply. Under this logic, a majority of the chemical specific differences would be related to kinetics (uptake and bioavailability, persistence and half-life, and potency to act on HPPD). An AFAD derived based on the dynamic effect of increased tyrosine would prevent any downstream adverse outcomes resulting from increased tyrosine, be they ocular effects or developmental effects. Future work is needed to test this hypothesis with additional in vitro/in vivo datasets for other HPPD inhibitors and with supportive TD modeling approaches. Additionally, identification of a similar ratio between the in vitro datasets (complete HPPD inhibition) with the in vivo datasets at the selected doses (likely partial HPPD inhibition) supports the dose selection and the derived AFAD.
It should be noted that the kinetics (with a relationship to administered dose) of the specific HPPD inhibitors are important factors in determining the impact of exposure to the compound on plasma tyrosine (JMPR Citation2014). For example, studies in humans exposed to nitisinone at a dose of 1 mg/kg reported much higher plasma tyrosine concentrations than studies in which mesotrione was administered at similar doses, and this likely reflects the very large differences in half-life between these two compounds (54 h and 1 h, respectively) (Hall et al. Citation2001; JMPR Citation2014). Therefore, consideration of compound kinetics and dosing scheme are important when evaluating a chemical agnostic AFAD for human health risk assessment. It is generally understood, however, that human exposure to agrochemical HPPD inhibitors is low, and the kinetics of such compounds suggest neither accumulation potential, nor high enough exposure concentrations to induce this AOP (US EPA Citation2020b). Future development of toxicokinetic models will aid in derivation of a DDEF that includes AFAK for other HPPD inhibitors and will aid in deriving DDEF’s between additional species (mouse, dog). This may, in turn, support a refined composite DDEF for other species extrapolations which may be lower or higher. Future work might also derive a chemical specific relative potency AFAK based on the modeled ADME profile for HPPD inhibitors. This would be akin to deriving a relative potency factor as is commonly done for carcinogens or compounds acting via a similar mode of action, and similar to work already conducted to evaluate cumulative risk assessment for HPPD inhibitors as a class (US EPA Citation2021). This approach would require an integration of both kinetic and dynamic datasets in order to capture aspects related to half-life and AUC/Cmax.
Our results align with other approaches to utilize a CSAF/DDEF for other HPPD inhibitors. The JMPR conducted a risk assessment for the HPPD inhibitor bicyclopyrone, using a study conducted in rats as the basis for the acceptable daily intake (ADI) (JMPR Citation2017). While the approach was only semi-quantitative, JMPR justified the reduction of the default 10-fold AFA to 3.3-fold. They discuss the basis for this by citing data showing at least 3-fold higher activity in human TAT as compared to rat, and they use this to reduce the default AFAD of 2.5-fold by 3x, to get a factor 0.83 for dynamics. Multiplying the AFAD of 0.83 by the default AFAK of 4, results in a CSAF of 3.3 (JMPR Citation2017). The AFAD derived by JMPR of 0.83 for bicyclopyrone is higher than the derived chemical agnostic AFAD in this paper of 0.3. However, JMPR utilized a different method for CSAF derivation, and both values are less than 1, again reflecting the reduced sensitivity of humans compared to rats. Similarly, and as noted above, the US EPA has repeatedly evaluated the data for HPPD inhibiting agrochemicals and determined that the rat is less sensitive than humans on the basis of toxicodynamic differences (US EPA Citation2015a, Citation2020b, Citation2020c, Citation2022).
In general, these quantitative species differences support the large body of literature suggesting the biology driving species differences is related to TAT activity and tyrosine clearance mechanisms. However, explicit TAT activity may account for only some the identified species sensitivities, with other factors contributing as well. This is especially important at current human environmental exposure levels, which are not high enough to completely inhibit HPPD. It is probable that under incomplete HPPD inhibition, additional mechanisms, in addition to increased TAT activity, might contribute to reduced sensitivities for humans. Indeed, plasma tyrosine concentration should reflect not only the TAT activity level, but additionally the rate of formation/absorption of free tyrosine and the elimination rates of HPPA and related metabolic products (Lewis and Botham Citation2013). For example, higher excretion of HPPA or conversion to HPLA in the human compared to the rat could overcome any negative feedback loops or equilibrium effects that might exist between HPPA and tyrosine, supporting a reduction of plasma tyrosine in these species. Additionally, it is feasible that the rat has an increased ability to convert HPPA back into tyrosine as compared to the mouse or human, since the TAT reaction is reversible. It is also possible that increased reabsorption of urinary tyrosine in the rat could be a contributing factor to maintaining increased plasma levels (Hall et al. Citation2001). Increased sequestration of tyrosine in the liver or other target tissues in the rat (as opposed to blood or plasma concentrations) may allow for tissue-specific accumulation which can be released back into circulation following conversion to HPPA in the blood. This hypothesis is somewhat supported by observations made by Ward et al. (Citation2017) following development of a physiologically based pharmacokinetic (PBPK) model for nitisinone-induced tyrosinemia in rats. In their study, the authors hypothesized that tyrosine blood level might not fully reflect the total systemic tyrosine that would include liver and other tissue concentrations (Ward et al. Citation2017). Additionally, the buildup of HPPA prevents further conversion of tyrosine in the rat, but there may be higher accumulation or excretion rates in the human and mouse. This suggests that the conversion rate of HPPA to HPLA would also contribute to reduced tyrosine levels. These hypotheses could help to explain the different thresholds for plasma tyrosine build-up across species when excluding differences in TAT activity. In either case, the data as evaluated, in using plasma tyrosine levels (AUC or Cmax) or TAT activity (HPPA formation) show that humans are much less sensitive to the effects of HPPD inhibition as compared to rats due to toxicodynamic differences.
4. Conclusion
While the best science approaches to human health risk assessments advocate use of data in the place of default adjustment factors, this is not common practice in the context of agrochemical risk assessments. There are typically sufficient data to replace default adjustment factors given the extensive toxicological data packages, and assessments should aim to incorporate these data, where possible and appropriate. Specifically, case studies demonstrating application of data-derived approaches are critical to confidence building and to facilitate and advance regulatory adoption. To support this initiative, we present here a DDEF for rat to human extrapolation (AFA) for the HPPD inhibitor mesotrione, given that for this compound, human kinetic and dynamic data exist. The DDEF applies a derived AFAK of 2.5 for rat to human extrapolation based on in vivo comparative mesotrione kinetics multiplied by the AFAD of 0.3 based on in vivo comparative tyrosine dynamics, resulting in a DDEF of ∼1 (0.75). This reflects the understanding and available scientific evidence that humans are much less sensitive than rats to the effects of HPPD inhibitors on the basis of toxicodynamic differences (Lock et al. Citation2000; Lewis and Botham Citation2013; JMPR Citation2014; US EPA Citation2015a, Citation2020b; Botham et al. Citation2023). Additionally, utilizing comparative TAT-based in vitro datasets for mesotrione, the HPPD inhibitor nitisinone, or TAT activation via excess tyrosine, a chemical agnostic AFAD of 0.3 is hypothesized. These data demonstrate that humans are less sensitive than rats to the toxicodynamic effects (increased tyrosine plasma concentrations) leading to the adverse outcome (ocular effects) for HPPD inhibitors. Based on the concordance of the AFAD across compounds and datasets, and as supported by an understanding of the AOP by which the dynamics of complete HPPD inhibition would be expected to be the same regardless of the inhibitor, our analyses show potential for the development of a chemical agnostic AFAD. Future work can be conducted to further support the development of a chemically agnostic AFAD and can incorporate toxicokinetics on the basis of human to animal datasets (as exist for mesotrione) or the development of PBPK models. Other future work includes the development of DDEFs for mouse to human and dog to human extrapolation for HPPD inhibiting chemicals. Ultimately, integration of both TK and TD data could be used to develop a relative potency adjustment scalable to all HPPD inhibiting compounds. Furthermore, building chemically agnostic DDEFs for additional AOPs supports resource optimization and supports regulators who are often constricted by tight deadlines or resource limitations. This builds off of and advances the already existing industry-academic-regulatory partnerships and collaborative efforts on implementation of new approach methods into risk assessment. In this way, agrochemical human health risk assessments can incorporate the most robust scientific methods for the protection of human health.
Abbreviations | ||
ADME | = | absorption, distribution, metabolism, and excretion |
ADI | = | acceptable daily intake |
AR | = | accumulation ratio |
AF | = | adjustment factor |
AFH | = | adjustment factor for interhuman variability |
AFA | = | adjustment factor for interspecies extrapolation |
AFAK | = | adjustment factor for kinetic interspecies extrapolation |
AFAD | = | adjustment factor for dynamics interspecies extrapolation |
AOP | = | adverse outcome pathway |
AO | = | adverse outcome |
AUC | = | area under the time concentration curve |
CSAF | = | chemical specific adjustment factor |
Cmax | = | concentration maximum in the plasma |
DDEF | = | data derived extrapolation factor |
EFSA | = | European Food Safety Authority |
HEC | = | human equivalent concentration |
HED | = | human equivalent dose |
HPLA | = | 4-hydroxyphenyllactate |
HPPA | = | 4-hydroxyphenylpyruvic acid |
HHPD | = | 4-hydroxyphenol pyruvate dioxygenase |
IRIS | = | Integrated Risk Information System |
IPCS | = | International Programme for Chemical Safety |
IVIVE | = | in vitro to in vivo extrapolation |
JMPR | = | Joint FAO/WHO Meeting on Pesticide Residues |
MOA | = | mode of action |
MIE | = | molecular initiating event |
NTBC | = | nitisinone (2-(4-methylsulfonyl-2-nitrobenzoyl)-1,2-cyclohexanedione, brand name ORFADIN®) |
POD | = | point of departure |
PMRA | = | Pest Management Regulatory Agency |
PBPK | = | physiologically based pharmacokinetic |
RfD | = | reference dose |
TK | = | toxicokinetic |
TD | = | toxicodynamic |
TAT | = | tyrosine aminotransferase |
US FDA | = | United States Food and Drug Administration |
US EPA | = | US Environmental Protection Agency |
Supplemental Material
Download MS Word (44.1 KB)Acknowledgments
The authors would like to express our sincere gratitude to the anonymous referees selected by the Editor for the detailed comments and suggested revisions which have greatly enhanced the current manuscript.
Declaration of interest
The authors report there are no competing interests to declare. This research was funded by Syngenta AG which is a registrant of HPPD-inhibiting agrochemicals. The authors are all employees of Syngenta and have all contributed to various aspects of this project over several years. While Syngenta conducts internal reviews of employee manuscripts pre-submission, no substantive scientific changes were recommended internally during these reviews.
References
- [AOP] Adverse Outcome Pathway. 2024. HPPD inhibition leading to corneal papillomas and carcinomas (in rat); [accessed 2024 Jan 18]. https://aopwiki.org/aops/114.
- Bhat VS, Meek MEB, Valcke M, English C, Boobis A, Brown R. 2017. Evolution of chemical-specific adjustment factors (CSAF) based on recent international experience: increasing utility and facilitating regulatory acceptance. Crit Rev Toxicol. 47(9):729–749. doi: 10.1080/10408444.2017.1303818.
- Botham J, Lewis RW, Travis KZ, Baze A, Richert L, Codrea E, Semino Beninel G, Garcin JC, Strupp C. 2023. Species differences and human relevance of the toxicity of 4-hydroxyphenylpyruvate dioxygenase (HPPD) inhibitors and a new approach method in vitro for investigation. Arch Toxicol. 97(4):991–999. doi: 10.1007/s00204-023-03458-8.
- Burns RP, Gipson IK, Murray MJ. 1976. Keratopathy in tyrosinemia. Birth Defects Orig Artic Ser. 12(3):169–180 [as cited in Lewis and Botham, 2013].
- Calabrese EJ, Gilbert CE. 1993. Lack of total independence of uncertainty factors (UFs): implications for the size of the total uncertainty factor. Regul Toxicol Pharmacol. 17(1):44–51. doi: 10.1006/rtph.1993.1005.
- Dourson M, Becker RA, Haber LT, Pottenger LH, Bredfeldt T, Fenner-Crisp PA. 2013. Advancing human health risk assessment: integrating recent advisory committee recommendations. Crit Rev Toxicol. 43(6):467–492. doi: 10.3109/10408444.2013.807223.
- Dourson ML, Stara JF. 1983. Regulatory history and experimental support of uncertainty (safety) factors. Regul Toxicol Pharmacol. 3(3):224–238. doi: 10.1016/0273-2300(83)90030-2.
- [EFSA] European Food Safety Authority. EFSA Scientific Committee. 2012. Guidance on selected default values to be used by the EFSA Scientific Committee, Scientific Panels and Units in the absence of actual measured data. EFSA J. 10(3):2579.
- Gibbs JP, Yang JS, Slattery JT. 1998. Comparison of human liver and small intestinal glutathione S-transferase-catalyzed busulfan conjugation in vitro. Drug Metab Dispos. 26(1):52–55.
- Hall MG, Wilks MF, Provan WM, Eksborg S, Lumholtz B. 2001. Pharmacokinetics and pharmacodynamics of NTBC (2-(2-nitro-4-fluoromethylbenzoyl)-1,3-cyclohexanedione) and mesotrione, inhibitors of 4-hydroxyphenyl pyruvate dioxygenase (HPPD) following a single dose to healthy male volunteers. Br J Clin Pharmacol. 52(2):169–177. doi: 10.1046/j.0306-5251.2001.01421.x.
- [IPCS] International Programme for Chemical Safety. 2005. Chemical-specific adjustment factors for interspecies differences and human variability: guidance document for use of data in dose/concentration–response assessment. Harmonization Project Document No. 2; [accessed 2024 Jan 18]. https://www.who.int/publications/i/item/9241546786.
- [JMPR] Joint FAO/WHO Meeting on Pesticide Residues. 2014. Pesticide residues in food – 2014. Part II—toxicological evaluations. Mesotrione. Joint Meeting of the FAO Panel of Experts on Pesticide Residues in Food and the Environment and the WHO Core Assessment Group on Pesticide Residues; September 16–25; Rome, Italy; [accessed 2024 Jan 18]. https://inchem.org/documents/jmpr/jmpmono/v2014pr01.pdf.
- [JMPR] Joint FAO/WHO Meeting on Pesticide Residues. 2017. Pesticide residues in food - 2017. 5.3 Bicyclopyrone (295). Report of the Joint Meeting of the FAO Panel of Experts on Pesticide Residues in Food and the Environment and the WHO Core Assessment Group on Pesticide Residues; September 12–21; Geneva, Switzerland; [accessed 2024 Jan 18]. https://www.fao.org/fileadmin/templates/agphome/documents/Pests_Pesticides/JMPR/Report2017/5.3_BICYCLOPYRONE__295_.pdf.
- Lehman AJ, Fitzhugh OG. 1954. 100-fold margin of safety. Q Bull Assoc Food Drug Off. 18(1):33–35 [as cited in Dourson and Stara, 1983].
- Lewis RW, Botham JW. 2013. A review of the mode of toxicity and relevance to humans of the triketone herbicide 2-(4-methylsulfonyl-2-nitrobenzoyl)-1,3-cyclohexanedione. Crit Rev Toxicol. 43(3):185–199. doi: 10.3109/10408444.2013.764279.
- Lock EA, Gaskin P, Ellis MK, McLean Provan W, Robinson M, Smith LL. 2000. Tissue distribution of 2-(2-nitro-4-trifluoromethylbenzoyl)-cyclohexane-1,3-dione (NTBC) and its effect on enzymes involved in tyrosine catabolism in the mouse. Toxicology. 144(1-3):179–187. doi: 10.1016/s0300-483x(99)00205-x.
- Lock EA, Gaskin P, Ellis MK, Provan WM, Robinson M, Smith LL, Prisbylla MP, Mutter LC. 1996. Tissue distribution of 2-(2-nitro-4-trifluoromethylbenzoyl)cyclohexane-1-3-dione (NTBC): effect on enzymes involved in tyrosine catabolism and relevance to ocular toxicity in the rat. Toxicol Appl Pharmacol. 141(2):439–447. doi: 10.1006/taap.1996.0310.
- Martin OV, Martin S, Kortenkamp A. 2013. Dispelling urban myths about default uncertainty factors in chemical risk assessment–sufficient protection against mixture effects? Environ Health. 12(1):53. doi: 10.1186/1476-069X-12-53.
- Musther H, Harwood MD, Yang J, Turner DB, Rostami-Hodjegan A, Jamei M. 2017. The constraints, construction, and verification of a strain-specific physiologically based pharmacokinetic rat model. J Pharm Sci. 106(9):2826–2838. doi: 10.1016/j.xphs.2017.05.003.
- Pecquet AM, Haber LT. 2023. Noncancer risk assessment: principles and practice in environmental and occupational settings. In: Paustenbach D, Farland W, et al., editors. Patty’s toxicology. Hoboken (NJ): John Wiley and Sons; p. 1–45. doi: 10.1002/0471125474.
- Peters SA. 2021. Physiologically based pharmacokinetic (PBPK) modeling and simulations: principles, methods, and applications in the pharmaceutical industry. 2nd ed. Hoboken (NJ): Wiley; p. 1–624.
- [PMRA] Pest Management Regulatory Agency. 2008. The application of uncertainty factors and the pest control products act factor in the human health risk assessment of pesticides. Science Policy Note, SPN2008-01. Ottawa (ON): Health Canada.
- [PMRA] Pest Management Regulatory Agency. 2016. Sulfoxaflor registration decision RD2016-12, sulfoxaflor, April 1, 2016. Health Canada; [accessed 2024 Apr 22]. https://publications.gc.ca/collections/collection_2016/sc-hc/H113-25-2016-12-eng.pdf.
- [PMRA] Pest Management Regulatory Agency. 2021. A framework for risk assessment and risk management of pest control products. PMRA Guidance Document, Health Canada; [accessed 2024 Jan 18]. https://www.canada.ca/en/health-canada/services/consumer-product-safety/reports-publications/pesticides-pest-management/policies-guidelines/risk-management-pest-control-products.html.
- Reichard JF, Maier MA, Naumann BD, Pecquet AM, Pfister T, Sandhu R, Sargent EV, Streeter AJ, Weideman PA. 2016. Toxicokinetic and toxicodynamic considerations when deriving health-based exposure limits for pharmaceuticals. Regul Toxicol Pharmacol. 79 Suppl 1:s 67–s 78. doi: 10.1016/j.yrtph.2016.05.027.
- Renwick AG. 1991. Safety factors and establishment of acceptable daily intakes. Food Addit Contam. 8(2):135–149. doi: 10.1080/02652039109373964.
- Renwick AG. 1993. Data-derived safety factors for the evaluation of food additives and environmental contaminants. Food Addit Contam. 10(3):275–305. doi: 10.1080/02652039309374152.
- Renwick AG, Lazarus NR. 1998. Human variability and noncancer risk assessment- an analysis of the default uncertainty factor. Regul Toxicol Pharmacol. 27(1 Pt 2):3–20. doi: 10.1006/rtph.1997.1195.
- Rich LF, Beard ME, Burns RP. 1973. Excess dietary tyrosine and corneal lesions. Exp Eye Res. 17(1):87–97. doi: 10.1016/0014-4835(73)90170-x.[as cited in Lewis and Botham, 2013].
- Terry C, Hays S, McCoy AT, McFadden LG, Aggarwal M, Rasoulpour RJ, Juberg DR. 2016. Implementing a framework for integrating toxicokinetics into human health risk assessment for agrochemicals. Regul Toxicol Pharmacol. 75:89–104. doi: 10.1016/j.yrtph.2015.10.003.
- [US EPA] US Environmental Protection Agency. 2002. A review of the reference dose and reference concentration processes. Washington (DC): Risk Assessment Forum. EPA/630/P-02/002F.
- [US EPA] US Environmental Protection Agency. 2014. Guidance for applying quantitative data to develop data-derived extrapolation factors for interspecies and intraspecies extrapolation. Washington (DC): Office of the Science Advisor, Risk Assessment Forum. EPA/100/R-14/002F.
- [US EPA] US Environmental Protection Agency. 2015a. Mesotrione. Human health risk assessment for amended uses on corn. Health effects division. Washington (DC): US EPA; D427385.
- [US EPA] US Environmental Protection Agency. 2015b. Proposed approach to efficiently develop physiologically based pharmacokinetic (PBPK) & physiologically based pharmacokinetic-pharmacodynamic (PBPK-PD) models for pesticides. Office of Pesticide Programs; [updated 2023 Apr 10; accessed 2024 Jan 1]. https://www.epa.gov/pesticide-science-and-assessing-pesticide-risks/developing-tiered-framework-extrapolation-modeling.
- [US EPA] US Environmental Protection Agency. 2018. Simazine. Human health risk assessment for registration review and to support the registration of proposed uses on citrus fruit (crop group 10-10), pome fruit (crop group 11-10), stone fruit (crop group 12-12), tree nuts (crop group 14-12), and tolerance amendment for almond hulls. Health Effects Division, Office of Pesticide Programs. Washington (DC): US EPA; DP Nos. D402163, D428603.
- [US EPA] US Environmental Protection Agency. 2020a. Chlorpyrifos: third Revised Human Health Risk Assessment for Registration Review. Health Effects Division, Office of Pesticide Programs. Washington (DC): US EPA; D456427.
- [US EPA] US Environmental Protection Agency. 2020b. HPPD Inhibiting Herbicides: state of the Science. Health Effects Division, Office of Pesticide Programs. Washington (DC): US EPA; D439367.
- [US EPA] US Environmental Protection Agency. 2020c. Mesotrione: draft Human Health Risk Assessment to Support Registration Review. Health Effects Division, Office of Pesticide Programs. Washington (DC): US EPA; D453769.
- [US EPA] US Environmental Protection Agency. 2021. P-Hydroxyphenyl-Pyruvate Dioxygenase (HPPD) Inhibitors Cumulative Risk Assessment. Health Effects Division, Office of Pesticide Programs. Washington (DC): US EPA; EPA-HQ-OPP-2020-0391-0007.
- [US EPA] US Environmental Protection Agency. 2022. Bicyclopyrone: human Health Risk Assessment for the Establishment of Permanent Tolerances for Residues in/on Bananas, Broccoli, Dry Bulb Onions, Green Onion, Hops, Horseradish, Papaya, Strawberry, Sweet Potatoes, Timothy Forage, Timothy Hay, and Watermelon. Health Effects Division, Office of Pesticide Programs. Washington (DC): US EPA; D459563.
- [US FDA] US Food and Drug Administration. 2002. ORFADIN(R) capsules (nitisinone); [accessed 2024 Apr 18]. https://www.accessdata.fda.gov/drugsatfda_docs/label/2002/21232lbl.pdf.
- Ward JP, Dunster JL, Derks G, Mistry P, Salazar JD. 2017. Predicting tyrosinaemia: a mathematical model of 4-hydroxyphenylpyruvate dioxygenase inhibition by nitisinone in rats. Math Med Biol. 34(3):335–390. doi: 10.1093/imammb/dqw006.
- [WHO] World Health Organization. 1958. Procedures for the testing of intentional food additives to establish their safety for use. Second report of the joint FAO/WHO expert committee on food additives. Technical report series No 144. Geneva (Switzerland): WHO; [accessed 2024 Jan 18]. https://iris.who.int/bitstream/handle/10665/40403/WHO_TRS_144.pdf?sequence=1.