ABSTRACT
The objective of this study was to determine whether ultraviolet-light-emitting diodes (UV-LEDs) could serve as an efficient photon source for heterogeneous photocatalytic oxidation (PCO). An LED module consisting of 12 high-power UV-A (λmax = 365 nm) LEDs was designed to be interchangeable with a UV-A fluorescent black light blue (BLB) lamp for a bench scale annular reactor packed with silica-titania composite (STC) pellets. Lighting and thermal properties of the module were characterized to assess its uniformity and total irradiance. A forward current (I F) of 100 mA delivered an average irradiance of 4.0 mW cm−2 at a distance of 8 mm, which is equivalent to the maximum output of the BLB, but the irradiance of the LED module was less uniform than that of the BLB. The LED and BLB reactors were tested for the oxidization of ethanol (50 ppmv) in a continuous-flow-through mode with 0.94 sec residence time. At the same average irradiance, the UV-A LED reactor resulted in a lower CO2 production rate (19.8 vs. 28.6 nmol L−1 s−1), lower ethanol removal (80% vs. 91%), and lower mineralization efficiency (28% vs. 44%) than the UV-A BLB reactor. Ethanol mineralization was enhanced with the increase of the irradiance at the catalyst surface. This result suggests that reduced ethanol mineralization in the LED reactor relative to the BLB reactor at the same average irradiance could be attributed to the nonuniform irradiance over the photocatalyst, that is, a portion of the catalyst was exposed to less than the average irradiance. The potential of UV-A LEDs may be fully realized by optimizing the light distribution over the catalyst and utilizing their instantaneous “on” and “off” feature for periodic irradiation. Nevertheless, our results also showed that the current UV-A LED module had the same wall plug efficiency (WPE) of 13% as that of the UV-A BLB, demonstrating that UV-A LEDs are a viable photon source both in terms of WPE and PCO efficiency.
Mercury (Hg)-vapor lamps are common UV sources for photocatalysis but create safety and environmental concerns because they contain Hg; furthermore, they have a relatively short life span. This paper demonstrated that the UV-A LED is a viable alternative to the Hg-vapor lamps without sacrificing PCO efficiency if the design of the LED arrays is improved to increase the lighting uniformity. The use of LEDs could eliminate hazardous Hg wastes and extend the application of photocatalysis in places requiring more compact and robust air purification solutions.
INTRODUCTION
The ability of titanium dioxide (TiO2)-assisted photocatalytic oxidation (PCO) to mineralize a broad range of organic contaminants into CO2 and H2O at room temperature has attracted attention for various environmental applications. This technique has been investigated as an alternative or complimentary method for air contaminant control as well as a means for treating water and wastewater.Citation1–8 The TiO2-catalyzed PCO process typically requires a light source with a wavelength below 388 nm. Mercury vapor lamps such as ultraviolet (UV)-A black lights and UV-C germicidal lamps have been widely used in laboratory and commercial PCO systems (e.g., Genesis Air Photocatalyst Gap, Ultra Sun Technologies, and Mazyck Technologies). However, these lamps contain trace amounts of toxic mercury, are fragile, and have a relatively short life span (<12,000 hr). Mercury is a highly toxic and controlled substance, and it is becoming banned by government safety and environmental regulators. Although there are nonmercury lamps, for example, microwave UV sources, they are driven by magnetrons that must withstand long duty cycles and the microwaves must be contained for safety purposes.
On the other hand, light-emitting diodes (LEDs; semiconductor-based lighting devices) are compact, reliable, and long lasting devices. LEDs are driven by direct current, can accommodate rapid “on” and “off” power toggling, and do not contain toxic mercury (Hg). They are entering the market of various illumination applications at an unprecedented speed. Since the development of the first commercial visible LEDs in 1968, the light output from a single device has increased by a factor of 20 per decade, while the price in US dollars per lumen has declined by a factor of 10 per decade.Citation9 White-light LEDs are now surpassing the efficiency of linear fluorescent and compact fluorescent lamps.Citation9 Ultraviolet-light-emitting diodes (UV-LEDs) have been commercially available since 2003. Currently, UV-A LEDs have a life expectancy of 50,000 hr at L 50 (life expectancy to 50% of the original light output), about 5 times that of Hg-vapor lamps. Naturally, they have been considered as an alternative UV source for photocatalysis for both gaseous and aqueous applications.Citation10–13 However, most of the PCO studies to date were conducted with low-power LEDs of varied wavelengths, including those outside of the TiO2 action spectrum (e.g., 395 and 405 nmCitation12,Citation13) in a variety of designs. Chen et al. studied photocatalytic degradation of percholoroethylene (PCE) in a rectangular steel gas-phase reactor irradiated by 16 Nichia 375-nm UV-LEDs (1.0 mW each) and found only 43% degradation of PCE in the LED reactor, whereas there was 90% degradation in a UV-A black-light reactor.Citation10 This result seemed to imply that LEDs are less effective than the black fluorescent light. Ciambelli et al. investigated the photocatalytic breakdown of benzene in a laboratory scale fluidized bed reactor irradiated by two or four Nichia 365 nm UV-LED modules and showed a 27% conversion of benzene into CO2 at 80 °C.Citation11 Although these studies proved that UV-LEDs were promising as a light source, no data were provided regarding their PCO performance relative to Hg-vapor lamps at the same irradiance, their actual power use efficiency, or issues related to the integration of LEDs into PCO reactors. The objective of this study was to design an LED PCO reactor for directly comparing the performance of state-of-the-art UV-A LEDs and an Hg-vapor UV-A BLB in oxidation of a model pollutant (ethanol), and ultimately address the central question whether UV-LEDs could serve as an effective photon source in heterogeneous PCO.
EXPERIMENTAL METHODS
UV Sources and LED Module Design
An 8-W UV-A (F8T5) fluorescent black light blue (BLB) lamp from Philips was used as the control light source. The BLB lamp dimensions were 15.6 mm depth × 304.8 mm length and the irradiance was 2.5 mW cm−2 at a distance of 25.4 mm. The LEDs used for the study were high-power chip-type UV-A LEDs (model NCSU033B) from Nichia Corporation (Tokushima, Japan), having a central wavelength of 365 nm, a spectrum half-width of 9 nm, and irradiance angle of 110°.Citation14 LEDs are directional light sources and their lighting intensity varies with the angular displacement (i.e., a Lambertian distribution of radiation). The total optical output of a single LED was 325 mW at a forward current of 500 mA and voltage of 3.8 V (i.e., 1.9 W).
In order to directly compare the effectiveness and efficiency of UV-A LEDs with the UV-A BLB in PCO, the LED module must meet three criteria: (1) interchangeability with the BLB in the same PCO reactor; (2) similarity of the irradiance profile between the LED module and the BLB (i.e., nearly isotropic); and (3) a wide range of irradiances, including one equivalent to that of the 8-W BLB. Accordingly, an LED assembly was designed to simulate the geometry of the linear fluorescent lamp. The spacing and arrangement of individual LEDs were determined by mathematical modeling (). Uniformity of the irradiance increased as the distance between LEDs decreased (). An assembly with densely populated LEDs (e.g., 15 mm vs. 30 mm spacing between LEDs) would provide a more uniform irradiance, but the power consumption and initial investment (number of the LEDs) would increase. Furthermore, greater numbers of LEDs would create a thermal management challenge, where lower operating temperatures are preferred to maintain a long operating life of the LEDs. The coefficient of temperature increase per unit electric power input is dependent upon the thermal resistance of the LED system (e.g., R ja = 35 °C/W with Nichia's standard circuit board), density of the LEDs on a circuit board, and factors associated with the operation environment. The radial irradiance profile of the LED assembly was modeled based on four linear LED arrays evenly placed every 90° in a 360° arrangement. The model examined the effect of viewing angle and distance between the light source and the object to be irradiated (). Because the object to be irradiated was the photocatalyst packed in an annular reactor (see details in PCO reactor design), the diameter of the quartz sleeve separating the light source from the catalyst bed was subsequently optimized by this modeling exercise. Although increasing the diameter enhanced the uniformity of light distribution, the power per unit area (E) decreased approximately following an inverse-square law (). suggests that 20 mm spaces between LEDs, and a 25-mm-diameter quartz sleeve would give satisfactory uniformity and sufficient intensity. Consequently, 12 LEDs were mounted on a 15.6-mm-diameter aluminum rod in four linear arrays, three LEDs in each array with a 20 mm spacing between the LEDs as shown in
Photocatalytic Oxidation (PCO) Reactor
Silica-titania composite (STC) pellets from Sol-gel Solutions, LLC (Gainesville, FL) served as the photocatalyst. The STC has the same porosity (30–40 Å) and TiO2 loading (4 g Degussa P-25 in 100 mL silica precursor, tetraethyl orthosilicate) as that used by Stokke and Mazyck.Citation15 An annular reactor, shown in , was used to carry out this study because of its simplicity and efficiency in utilizing traditional linear fluorescent lamps. It consisted of two concentric cylinders, with an annulus formed between an aluminum housing and a quartz sleeve. The light source was coaxially located inside the quartz sleeve, and STC pellets were packed in the annulus. Two reactor design parameters were optimized: (1) the diameter of the quartz sleeve that determines the distance between the photocatalyst and light sources and (2) the annulus size. The former was especially critical with LEDs as the light source. The effect of the quartz sleeve's diameter was illustrated in The annulus space that determines the thickness of the catalyst bed should be small enough to ensure a minimal gradient of UV irradiance across the catalyst bed and large enough to allow reproducible packing of STC pellets. Results of a preliminary light transmittance measurement showed that STC pellets in an annulus of 8 mm used by Stokke and MazyckCitation15 attenuated 97% of a UV-A BLB light, leaving the backside of the catalyst bed insufficiently illuminated. This prompted us to reduce the annulus size of the reactor, to 5.05 mm. Key parameters of the bench-scale test reactor used in this study and that used by Stokke and MazyckCitation15 are listed in for comparison. Because of the reduced annulus, smaller pellets than those used in Stokke and MazyckCitation15 were employed. As shown in , the STC pellets are truncated pyramids, most of them (>95%) are 1.5 ± 0.1 mm wide and 6.1 ± 0.1 mm long. The reactor was filled with 14.6 g of the STC pellets. A uniform catalyst bed was achieved by gently tapping the reactor wall until reaching a consistent height of 60 mm. This translated into a packing density of 0.46 g STC cm−3 and a void volume fraction of 74% as determined by fluid displacement.
Table 1. Comparison of test reactors
Light Source Characterization
Spectral quality and quantity of the light sources were assessed outside the PCO reactor in a dark room using a spectroradiometer (OL754C; Optronics Laboratories, Orlando, FL). The light source (either BLB or LED assembly) was centered inside a quartz sleeve of the same dimensions as that used in the reactor () and placed directly above the integrating sphere of the spectroradiometer. A 12.7-mm-diameter light attenuation aperture was placed before the integrating sphere entrance of 31.8 mm diameter. This setup () simulating the irradiance received at the surface of the catalyst bed (i.e., approximately 8 mm away from the light source) determined the incident photon flux. For the LED module, measurements were taken every 5 mm along the lateral direction both directly facing one of four LED arrays (designated as angle 0°) as shown in and between two linear LED arrays (designated as angle 45°). The irradiant output of the LED assembly and LED die's temperature were measured over a range of forward currents from 30 to 500 mA. The BLB was measured at three positions along the axis of the lamp.
PCO Tests
Performance of the UV-A BLB- and the LED-irradiated annular reactors was evaluated for the oxidation of ethanol. Ethanol (EtOH) was selected as a model pollutant in this study because (1) it is the most prevalent volatile organic compound (VOC) in the International Space Station air (accounting for 72% of alcohols, 57% of total nonmethane VOCs)Citation16 and represents a large class of polar volatile contaminants, alcohols; and (2) one of the proposed applications of the PCO technology is to remove polar VOCs from cabin air prior to a condensing heat exchanger in order to minimize VOC mass transfer into humidity condensate.Citation17 PCO tests were performed in an experimental setup as shown in that was capable of precisely controlling the experimental variables and of continuously monitoring the PCO reaction. The setup consisted of (1) a Kin-Tek trace gas generator (model 491M; La Marque, TX) for supplying a simulated contaminant air containing 50 ppmv ethanol (EtOH) and 72% relative humidity at 25 °C; (2) a PCO reactor packed with 14.6 g of STC pellets to a bed height of 60 mm; (3) two mass flow controllers for controlling the flows to the PCO reactor and CO2 analyzer; (4) temperature sensors for the reactor's inlet and outlet as well as for the determination of the temperature of the room; (5) humidity sensors for the reactor's influent and effluent air; (6) a CO2 analyzer for the reactor effluent; (7) a sample stream selecting valve; and (8) a gas chromatograph (ThermoFinnigan, Austin, TX) equipped with a flame ionization detector (GC/FID) and a HP Plot Q capillary column (30 m × 0.32 mm, 20 μm depth of film).
Figure 4. Illustration of the bench-scale PCO test bed employed to control the experimental parameters and monitoring the PCO process, objects are not to scale.
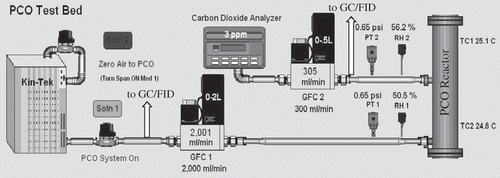
Tests were carried out in a flow-through mode with an uninterrupted 2 L min−1 air containing 50 ppmv EtOH under continuous illumination. Variation of the influent EtOH concentration was within 1%. Although ethanol concentrations in indoor air seldom exceeded 2 ppmv and had an average of 2.13 ppmv in the International Space Station,Citation16 such a high test concentration was chosen to hasten the deterioration of the catalyst within a reasonable experimental time period and test the robustness of the catalyst. Each test was repeated three times. Both influent and effluent streams were sampled alternately every 8.45 min and analyzed for ethanol as well as its oxidation intermediates by GC/FID. The effluent was also directed to a CO2 analyzer to determine the production rate of CO2, a complete mineralization product. CO2 concentration was recorded every minute. The reactor was maintained at 25 °C via forced air convection over heat sinks attached to the PCO reactor. The STC pellets were preconditioned with 72% RH, VOC-free air under continuous illumination. Each test began with the addition of ethanol to the air stream and continued for 21 hr, followed by regeneration with humidified, VOC-free air and continuous illumination. Completion of the regeneration was indicated by the absence of detectable organic species and only a baseline-level of CO2 in the effluent. The same batch of STC catalyst was repeatedly used for all tests reported in this paper. Consistent STC catalytic activity was ensured by periodical verification tests under the same experimental parameters.
Data Analysis
PCO performance was quantified in terms of EtOH removal and mineralization efficiency (X A). The former is a measure of the total removal of the test VOC, whether it is removed by adsorption or oxidation, whereas the latter is a measure of the complete oxidation of EtOH to CO2. These values were calculated using Equationeqs 1 and Equation2, respectively. C in and C out are the influent and effluent EtOH concentrations, and ∆C carbon dioxide is the CO2 generated from EtOH (a two-carbon molecule) degradation in the PCO process. The rate of photocatalytic oxidation of ethanol was determined based on the formation of CO2 instead of the disappearance of ethanol to prevent overestimation due to the adsorption of EtOH to the photocatalyst. Cumulative CO2 concentration was plotted against time; a linear relationship between the concentration and time suggested zero-order kinetics. The slope gave rise to the PCO rate (r). PCO photonic efficiency (ξ) was calculated as the ratio of the photocatalytic degradation rate to the incident photon flux ( Equationeq 3).
RESULTS AND DISCUSSION
Spectral Quality and Quantity of the Light Sources
Photon flux (molar photons per unit area) or irradiance (power per unit area) on the catalyst surface is one of the most important factors affecting photocatalytic oxidation efficiency. The LED assembly was extensively characterized in order to assess its irradiance uniformity and the required driving current for the LED module to provide an optical output similar to that of an 8-W BLB. Relative to the UV-A LED, the UV-A BLB had a broader peak (354–388 nm) with a central wavelength of 365 nm and low intensity peak at 405 nm that is out of the TiO2 action spectrum (<388 nm) (). The LED spectrum peak was narrower (357–378 nm) and all of the radiation fell within the TiO2's action spectrum. Furthermore, the spectra of the LED linear arrays (e.g., LED array 1 and array 2) were identical ().
The lateral irradiance profile of the LED assembly was measured every 5 mm from the first LED along the lateral axis directly facing one of the 4 arrays (angle 0°) as well as facing the space between two arrays (angle 45°) at a forward current of 100 mA. Evidently, the irradiance from the LED assembly was less uniform than desired (). The lowest intensity was about 55% of the peak intensity, occurring between two LEDs in a linear array. At a distance of 8 mm away from the source, the average irradiance (E) was 6.02 and 2.49 mW cm−2 for viewing angle 0° and 45°, respectively, which resulted in a mean of 4.25 mW cm−2 for the assembly. The overall mean irradiance for the LED module was 70% of the predicted value (). The discrepancy could be explained by the directionality (110°) of the LED radiation and how the light was measured (). The combination of the small sensor aperture (12.7 mm) and the close distance (approximately 8 mm) between the sensor and the light source prevented some of the photons from adjacent LEDs from entering the integrating sphere (). As a result, the measured value was somewhat underestimated compared to that obtained in the absence of the attenuation aperture (). However, because the STC pellets packed around the quartz sleeve in the PCO reactor block nearly all light transmittance, they would prevent photons outside of the 12.7-mm-diameter aperture from traveling further. We believed that the measured value was a more accurate representation of the light level the catalyst would intercept rather than the predicted value. In contrast, the 8-W UV-A BLB lamp from Philips measured in the same way showed a uniform irradiance of 4.0 ± 0.2 mW cm−2 along both the radial and lateral axes.
Figure 6. Irradiance profiles of the LED assembly determined at I F = 100 mA: (a) lateral and radial profiles on the outer surface of the quartz sleeve (OD 28 mm) where the photocatalyst is located; (b) comparison between measured average and model-predicted irradiance.
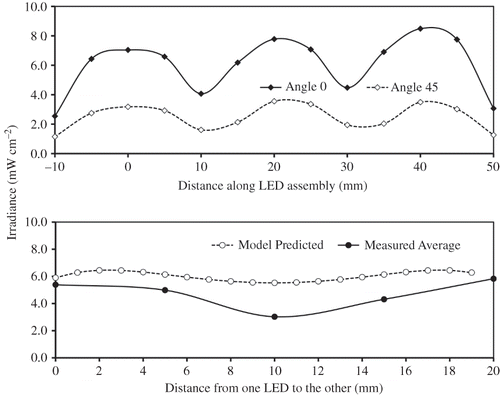
The light output of the LED assembly was also measured at I F between 30 to 500 mA. As with the individual LEDs, the irradiance of the LED assembly was directly proportional to the driving current in the range of 30 to 500 mA (E = 0.0449I F − 0.2235, R Citation2 = 0.9999, RSD < 8%). This relationship provided a simple means to vary the incident irradiance and study its effect on PCO. It also allowed for the determination of the required driving current (100 mA) to deliver the same irradiance of the 8-W fluorescent BLB.
Thermal Characteristics of the LED Assembly
LED life span can vary according to environmental and design factors. Although it is largely determined during the engineering phase of an LED module design, overdriving an LED assembly will decrease its life span if thermal management is inadequate. In order to assess the effectiveness of our heat management strategy, and to determine the upper limit of driving current (and hence the light output) for the assembly, the solder temperature (T s) of each LED in the assembly was measured at three driving currents (). At any given I F, T s was higher for the LED farther away from the heat sink (). Differences in T s among the LEDs along the lateral axis increased with the increase in driving current as indicated by the increasing relative standard deviation (), but was less than 3% even at 300 mA. The LED junction temperatures (T j) were calculated based on the thermal resistance 7 °C W−1 from the LED die to the measuring point. Results showed that a linear relationship between the driving current and measured solder temperature (T s) or calculated junction temperature (T j), is T j = 0.0857I F + 25.4 (R Citation2 = 0.9999). From this, the maximal allowable driving current was determined to be 870 mA for the LEDs to be operated below the manufacturer's recommended maximum T j of 100 °C. Because the LED used in this study was rated for a maximum forward current 700 mA, the assembly consisting of 12 LEDs electrically strung in two parallel series should allow for a maximum of 1400 mA and result in a light output of 62.6 mW cm−2 at a distance of 8 mm according to the established relationship between the irradiance and forward current (E = 0.0449I F − 0.2235). These findings suggest that the LED assembly had a greater light output potential (62.6 mW cm−2) than the current thermal management strategy could deliver (38.9 mW cm−2). In other words, the assembly can only fulfill 62% of its light output potential from the thermal perspective. This is primarily due to one of the design constraints for this first-generation LED module, where the LED module is required to be interchangeable with linear fluorescent lamps in the same reactor. Given such constraints, the four linear LED arrays were mounted linearly on a small aluminum rod; therefore thermal energy (e.g., 12 W at 500 mA driving current) had to be conducted to the ends for convective dissipation.
Table 2. LED solder temperature (T s) and dice temperature (T j) as a function of I F
PCO Efficiency of the BLB and LED-Irradiated Reactors
The PCO reactor effluent was found to consist of ethanol (influent pollutant), acetaldehyde (oxidation intermediate), and carbon dioxide (final oxidation product). Acetaldehyde (ACD) was the only quantifiable intermediate in the effluent as indicated by the lack of any other peaks in the GC chromatograms (data not shown). The effluent ethanol and acetaldehyde profiles resulting from the UV-A BLB-irradiated reactor () were similar to those reported for methanol oxidation.Citation18 Upon the initiation of ethanol-contaminated air flow, effluent ethanol concentration remained very low (2% of the feed) for the first 3 hr, increased at an accelerated rate between 3 and 10 hr, and continuously crept upwards even after 10 hr. This initial lag time for ethanol was attributed to the adsorption of ethanol by the STC pellets. In contrast, there was a very short (less than 30 min) for ACD and essentially no lag time for CO2. The concentration of ACD and CO2 in the effluent increased steeply upon the addition of feed contaminant, suggesting low and/or no affinity of ACD and CO2 to the STC. The concentration of CO2 approached a plateau or steady state after 5 hr, but that of ACD and ethanol reached somewhat steady state until 10 hr. Therefore, the time period between 10 and 20 hr was considered as the “pseudo-steady state” (PSS). The time course profiles of effluent ethanol, ACD, and CO2 from the UV-A LED reactor () resembled those of the BLB reactor in general shape, but differed in slope and concentration at the PSS.
Figure 7. Time-course of the effluent composition during STC-catalyzed oxidation of ethanol in the (a) UV-A LED and (b) UV-A BLB reactors at the same irradiance of 4 mW cm−2. CO2 concentration was recorded every minute and was affected by the sample stream valve position.
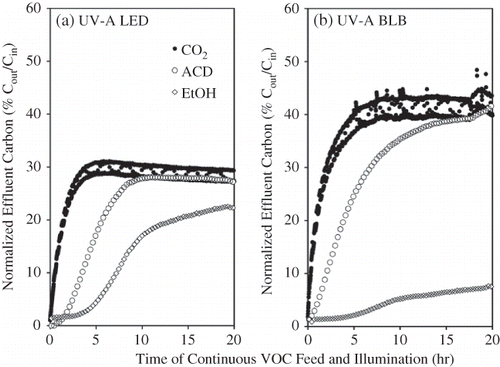
showed the relationship between the cumulative CO2 concentration and time in both LED- and BLB-irradiated reactors. Linear regressions suggest that the PCO of ethanol in both reactors followed a zero-order reaction kinetics. The slope of the regression gave rise to the CO2 evolution rate, or PCO rate. At an equivalent average irradiance, the LED- and BLB-irradiated reactors had a PCO rate of 19.8 and 28.6 nmol L−1 s−1, respectively. The average concentrations of effluent components at the PSS were used to assess the PCO efficiency in terms of ethanol removal and mineralization (). Compared with the UV-A BLB reactor, the LED reactor had a lower effluent ACD and CO2 but higher EtOH, which translated into lower EtOH removal, mineralization, POC rate, and photonic efficiency than the UV-A BLB reactor ( through e). The results do not necessarily imply that the LEDs were a less effective light source. Because of the fact that the LED module's irradiance was not as uniform as the BLB, some of the catalyst was irradiated by less than the average irradiance, which may have accounted for the reduced CO2 and ACD in the effluent.
Figure 8. A linear relationship between CO2 production and time during STC-catalyzed PCO of ethanol in both UV-A LED and BLB reactors, suggesting an zero-order reaction kinetics.
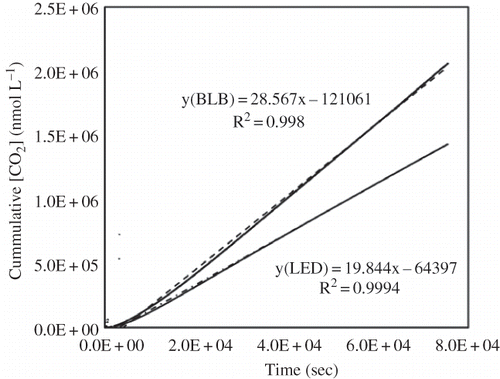
Table 3. Effluent composition and PCO efficiency at the pseudo-steady state from the BLB and LED reactors at the same irradiance
Figure 9. Effect of the LED average irradiance at the catalyst surface on: (a) reactor effluent composition, (b) ethanol removal, (c) ethanol mineralization, (d) PCO rate, and (e) photonic efficiency.
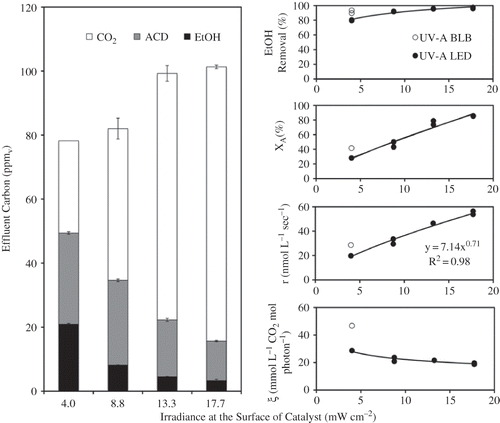
The effect of irradiance on PCO efficiency was subsequently examined in the LED reactor. Increasing the irradiance at the catalyst surface reduced effluent ACD and EtOH and increased CO2 production (). Both the mineralization efficiency and rate constant increased as the irradiance increased at less than first order (0.7) of the irradiance ( and d). The influence of increasing irradiance on the ethanol removal () was not as pronounced as for the mineralization. This is not surprising because EtOH removal is the result of both physical adsorption and PCO; the adsorption by the STCs should not be affected by incident irradiance. However, increasing the irradiance from 4.0 to 17.7 mW cm−2 decreased the photonic efficiency (ξ) by 33%. The relationship between mineralization and irradiance allowed us to predict that an irradiance of 7.6 mW cm−2 from the LED module would reach the same mineralization as that of the BLB at its full intensity. In other words, the LED reactor used in this study could achieve the same PCO efficiency for ethanol as an 8-W UV-A FL when it is operated at a forward current (I F) of 170 mA (i.e., a power input of 3.4 W). These values could be lowered by a LED module that provides more uniform irradiance over the entire surface of the photocatalyst.
Power Use Efficiency
The power use efficiency of a light source for PCO encompasses both the electric-quantum efficiency, or wall plug efficiency (WPE), and the reaction quantum efficiency. The WPE (defined as the percentage of irradiant output per electrical input) of the light sources used in this study is shown in . It is apparent that the WPE of the UV-A LEDs and UV-A FL were comparable, 17% as the manufacturer specified and 13% as measured in this study. It is interesting to note that the WPE efficiency is higher for LEDs of longer wavelength. For example, the same type of LED with a central wavelength of 385 nm has a WPE of 21.6%, that is, a 25% increase from that of the 365 nm LED. However, it is not known whether the gain in WPE would be offset by the potential loss in reaction quantum efficiency, because 385-nm LEDs approach the upper limit of the TiO2 action spectrum. In terms of reaction quantum efficiency, the LEDs in the current design were lower when compared to the UV-A FL (), but the gap could be closed if a more uniform irradiance is achieved. In addition, PCO efficiency may be enhanced by the exploitation of the instantaneous “on” and “off' feature of LEDs for periodic irradiation. It was previously demonstrated that photonic efficiencies for the decomposition of o-cresol by a UV/TiO2 process in a slurry reactor, under controlled periodic illumination of LEDs, was higher than that under continuous illumination.Citation13 As a result, the electric energy required for the same extent of degradation of contaminants decreased significantly by using periodic irradiation.
Table 4. Wall plug efficiency (WPE) of the light sources
CONCLUSIONS
This is the first report of a direct comparison between UV-A LEDs and a UV-A BLB as PCO light sources under similar irradiance. Challenges were encountered in achieving uniform LED irradiance over the photocatalyst while maintaining the power use efficiency. Increasing the density of LEDs could, no doubt, enhance the uniformity of the irradiance, but it would also increase the initial cost of a PCO reactor and the heat management requirement if high-power LEDs were used. The results from our LED reactor suggest that the number of LEDs per unit area may actually be reduced because the LED assembly could deliver up to 38.9 mW cm−2; an irradiance of 17.7 mW cm−2 resulted in a 97% EtOH removal and an 86% mineralization. It became clear that different design strategies should be considered depending upon the type of UV-A LEDsCitation19 to be used, e.g. a higher density of low-power LEDs (<10 mW) or the use of high-power LEDs (>100 mW) coupled with light dispersion. Typical approaches for light dispersion in laboratory-scale reactors include (a) coupling LEDs to light-transmitting optical fibers coated with a thin film of a photocatalyst and (b) using a waveguide through which light travels and emits from its sides into the surrounding catalyst. Although these approaches have the advantage of transferring the small area of an LED's illumination to a much greater surface area, each has its own drawbacks. The former has limited applications with respect to the thin films of catalysts because of TiO2’s opacity, whereas the latter creates a gradient of irradiance along the waveguide. A balance between side emission and transmission must be maintained to achieve uniform side-emission intensity over a reasonable length. We are currently investigating various light conduit materials and LED–light conduit coupling techniques for effective dispersion of high-power LED radiation.
The PCO efficiency, in terms of ethanol removal and mineralization, was greater in the UV-A BLB reactor than in the UV-A LED reactor at the same average irradiance (4.0 ± 0.2 mW cm−2). Irradiance and uniformity over the catalyst had a great impact on the PCO efficiency. The PCO efficiency increased as the irradiance over the surface of catalyst increased in the range tested (4–18 mW cm−2). We estimated that the LED reactor used in this study could achieve the same ethanol mineralization as an 8-W UV-A BLB when it is operated at a forward current (I F) of 170 mA, which corresponds to a power input of 3.4 W and an irradiance of 7.6 mW cm−2. These values are expected to be lower as uniform irradiance and/or periodic irradiation are implemented. The results proved that LEDs are a viable photon source both in terms of reaction quantum efficiency and wall plug efficiency. Continuing efforts in the following areas will strengthen this conclusion: (1) improvements in the design of the LED-PCO reactor for a higher power use efficiency; (2) investigation of the trade-off between the PCO reaction quantum efficiency and electric-quantum efficiency by using longer wavelength LEDs (e.g., 385 nm instead of 365 nm); and (3) using visible-light-responsive catalysts to take advantage of the higher electric quantum efficiency of longer-wavelength LEDs.
ACKNOWLEDGMENTS
The work was conducted under the auspices of the Life Science Support Contract and supported by a Kennedy Space Center Innovative Partnership Program (IPP) grant. The authors are extremely grateful to Dr. David Mazyck of the University of Florida for donating the photocatalyst. The authors would also like to thank Mr. Lawrence L. Koss for his invaluable assistance with the PCO test bed construction by making customized parts and Opto 22 data logging. The authors would also like to extend their appreciations to Mr. J. Schellack and Mr. D. Johnson of Lighting Science Groups Corporation for constructing the LED assembly and the KSC prototype shop personnel for fabricating the bench scale PCO reactor.
REFERENCES
- Hoffmann , M.R. , Martin , S.T. , Choi , W. and Bahnemann , D.W. 1995 . Environmental Applications of Semiconductor Photocatalysis . Chem. Rev. , 95 : 69 – 96 .
- Mo , J. , Zhang , Y. , Xu , Q. , Lamson , J.J. and Zhao , R. 2009 . Photocatalytic Purification of Volatile Organic Compounds in Indoor Air: A Literature Review . Atmos. Environ. , 43 : 2229 – 2246 .
- Hodgson , A.T. , Sullivan , D.P. and Fisk , W.J. Evaluation of Ultra-Violet Photocatalytic Oxidation (UV-PCO) for Indoor Air Applications: Conversion of Volatile Organic Compounds at Low Part-Per-Billion Concentrations; LBNL-58936 , 2005 Berkeley, CA : Lawrence Berkeley National Laboratory .
- Zhao , J. and Yang , X.D. 2003 . Photocatalytical Oxidation for Indoor Air Purification: A Literature Review . Build. Environ. , 38 : 645 – 654 .
- Tompkins , D.T. , Lawnicki , B.J. , Zeltner , W.A. and Anderson , M.A. 2005 . Evaluation of Photocatalysis for Gas-Phase Air Cleaning—Part 1: Process, Technical and Sizing Considerations . ASHRAE Trans. , 111 : 60 – 84 .
- Tompkins , D.T. , Lawnicki , B.J. , Zeltner , W.A. and Anderson , M.A. 2005 . Evaluation of Photocatalysis for Gas-Phase Air Cleaning—Part 2: Economics and Utilization . ASHRAE Trans. , 111 : 85 – 95 .
- Kwon , S. , Fanb , M. , Cooper , A.T. and Yang , H. 2008 . Photocatalytic Applications of Micro- and Nano-TiO2 in Environmental Engineering . Crit. Rev. Environ. Sci. Technol. , 38 : 197 – 226 .
- Yu , K.P. , Lee , W.M. and Huang , G.H. 2010 . The Effect of Ozone on the Removal Effectiveness of Photocatalysis on Indoor Gaseous Biogenic Volatile Organic Compound . J. Air Waste Manage. Assoc. , 60 : 820 – 829 . doi: doi: 10.3155/1047-3289.60.7.820
- Steele , R.V. 2007 . The Story of a New Light Source . Nat. Photonics , 1 : 25 – 26 .
- Chen , D.H. , Ye , X. and Li , K. 2005 . Oxidation of PCE with a UV LED Photocatalytic Reactor . Chem. Eng. Technol. , 28 : 95 – 97 .
- Ciambelli , P. , Sannino , D. , Palma , V. , Vaiano , V. and Mazzei , R.S. 2009 . A Step Forward in Ethanol Selective Photo-oxidation . Photochem. Photobiol. Sci. , 8 : 699 – 704 .
- Wang , W.Y. and Ku , Y. 2006 . Photocatalytic Degradation of Reactive Red 22 in Aqueous Solution by UV-LED Radiation . Water Res. , 40 : 2249 – 2258 .
- Chen , H.W. , Ku , Y. and Irawan , A. 2007 . Photodecomposition of o-Cresol by UV-LED/TiO2 Process with Controlled Periodic Illumination . Chemosphere , 69 : 184 – 190 .
- Nichia Corp. Specifications for Nichia Chip Type UV LED http://www.nichia.co.jp/specification/en/product/led/NCSU033B-E.pdf (http://www.nichia.co.jp/specification/en/product/led/NCSU033B-E.pdf) (Accessed: 27 July 2011 ).
- Stokke , J.M. and Mazyck , D.W. 2007 . Effect of Catalyst Support on the Photocatalytic Destruction of VOCs in a Packed-Bed Reactor . Proceeding of 37th International Conference on Environmental Systems . July 9–12 2007 , Chicago, IL. Warrendale, PA : Technical paper 2007-01-3138. Digital Media, SAE International .
- Perry , J.L. 2009 . A Design Basis for Spacecraft Cabin Trace Contaminant Control . Proceeding of 39th International Conference on Environmental Systems . July 12–16 2009 , Savannah, GA. Warrendale, PA : Technical paper 2009-01–2592. Digital Media, SAE International .
- O'Rourke , M.J.E. , Perry , J.L. and Carter , D.L. 2006 . A Water Recovery System Evolved for Exploration . Proceeding of 35th International Conference on Environmental Systems . July 17–20 2006 , Norfolk, VA. Warrendale, PA : Technical paper 2006-01–2274. Digital Media, SAE International .
- Stokke , J.M. and Mazyck , D.W. 2008 . Photocatalytic Degradation of Methanol Using Silica-Titania Pellets: Effect of Pore Size on Mass Transfer and Reaction Kinetics . Environ. Sci. Technol. , 42 : 3803 – 3813 .
- Sandhu , A. 2007 . The Future of Ultraviolet LEDs . Nat. Photonics , 1 : 38