Abstract
A novel biocover with passive air diffusion system (PADS) was designed in this study. Its effect on landfill gas components in the macrocosms of simulated biocover systems was also investigated. The results show that O2 concentration increased in the whole profile of the macrocosms equipped with PADS. When simulated landfill gas (SLFG) flow rate was no more than 40 mL min−1, the methane oxidation rate was 100%. The highest CH4 oxidation capacity reached to 31.34 mol m−3 day−1. Molecular microbiology analysis of the soil samples taken from the above macrocosm showed that the growth of type I methanotrophs was enhanced, attributable to enhanced air diffusion and distribution, whereas the microbial diversity and population density of type II methanotrophs were not so affected, as evidenced by the absence of any difference between the biocover equipped with PADS and that of the control. According to a phylogenic analysis, Methylobacter, Methylosarcina for type I, and Methylocystis, Methylosinus for type II, were the most prevalent species in the macrocosm with PADS.
Semiaerobic landfill technology was reported to be an effective in situ CH4 emission reduction method. A semiaerobic status is achieved through a continuous supply of fresh air sucked passively through the open end of a leachate collection pipe, with a temperature gradient created by organic matter degradation. In this research, a novel passive air diffusion system (PADS) was designed, and O2 concentration increased in the whole profile of the microcosms equipped with PADS. The constant supply of fresh air reduced CH4 production and enhanced biodegradation. Through molecular microbiology analysis, it was found that growth of methanotrophs was enhanced, and species of prevalent methanotrophs were found.
Supplemental materials are available for this article. Go to the publisher's online edition of the Journal of the Air & Waste Management Association for a list of abbreviations and PCR protocol.
Introduction
Methane (CH4) is an important greenhouse gas with a global warming potential that is 25 times greater than that of carbon dioxide (CO2), according to Intergovernmental Panel on CitationClimate Change 2007 (IPCC). Its contribution to escalating global warming is estimated at 15% (CitationThemelis and Ulloa, 2007). Landfill sites are thought to contribute 20 to 70 Tg of CH4 annually to global CH4 emissions and are the fourth largest anthropogenic source of CH4 worldwide (CitationBoeckx et al., 1996). According to the Annual Report on the State of the Environment in China (S.E.P. CitationAdministration, 2009), CH4 production from landfill account for 11% of the total amount in anthropogenic CH4 production in 2005, and it is predicted that the ratio will exceed 14% in 2015. CH4 production and emission from landfill in Beijing accounts for 39.48% (in 2005) of the total CH4 emission in the city, which is significantly higher than other sources. That is because landfill is the primary way (>88%) for municipal solid waste (MSW) treatment in Beijing.
Together with CH4 emissions from the fossil fuel sector, landfill CH4 emission requires the most urgent mitigation to stabilize CH4 concentration in the atmosphere (CitationStern and Kaufman, 1996). The oxidation of CH4 by bacteria residing in soil constitutes an important terrestrial CH4 sink. These bacteria are particularly abundant in the topsoil of landfill caps due to the high CH4 concentration supply from the landfill below. CitationWhalen et al. (1990) first reported the high CH4-oxidizing capabilities of landfill cover soil, which is capable of oxidizing CH4 at a rate of 2.8 mol m−2 day−1. Since then, various studies have focused on the biotransformation of CH4 in the landfill cover soils. Documented laboratory and field studies showed that landfill cover soil exposed to CH4 can develop high capacities for CH4 oxidation by indigenous methanotrophic microorganisms (CitationBogner et al., 1997; CitationChanton et al., 1999; CitationChristophersen et al., 2000; CitationCzepiel et al., 1996; CitationKightley et al., 1995; CitationLiptay et al., 1998). In recent years, landfill biofilter designed to optimize CH4 oxidation have demonstrated the potential to mitigate landfill CH4 emissions as high as 1900 g m−3 day−1 (CitationScheutz et al., 2009).
Traditionally, methanotrophs are classified into two general groups (type I and II) based on several characteristics, such as cell morphology, membrane arrangement, carbon assimilation pathway, and predominant phospholipid fatty acids (CitationHanson and Hanson, 1996). Type I methanotrophs, which belong to γ-proteobacteria, are composed of Methlyomonas, Methylococcus, Methylomicrobium, Methylosarcina, Methylosphaera, Methylothermus, Methylosoma, Methylohalobius, Methylocaldum, and Methylobacter (CitationHanson and Hanson, 1996; CitationRahalkar et al., 2007). A recent study (CitationLee, 2008) divided type I methanotrophs into two different groups, i.e., type Ia and Ib; the latter is composed of Methylococcus, Methylocaldum, and Methylothermus, whereas the rest of previous type I belong to type Ia methanotrophs. Genera that are members of type II methanotrophs belong to α-proteobacteria, including Methylosinus, Methylocella, Methylocapsa, and Methylocystis.
Type I methanotrophs whose thriving is much influenced by O2 grow faster than type II and thus type I methanotrophs are more sensitive to the environment, such as O2 concentration and nitrogen; by contrast, higher CH4 concentrations favor the growth of type II methanotrophs. CitationCrossman et al. (2004) confirmed that type I methanotroph is responsible for CH4 oxidation for the top section of the landfill cap, whereas type II methanotroph population is responsible for CH4 oxidation in the deepest section of the landfill.
The application of landfill cover layers for the CH4 oxidation is mostly influenced by the available O2 concentration (CitationNikiema et al., 2007). In order to reduce problems related to O2 diffusion in landfill covers and open biofilters, many authors prefer the use of multilayer beds (CitationBerger et al., 2005; CitationScheutz et al., 2009), compost (CitationStreese and Stegmann, 2003), soil (CitationParker et al., 2002), a mixture of compost and soil (CitationHaubrichs and Widmann, 2006), etc. Indeed, these porous materials can improve O2 transfer and distribution. However, they cannot overcome the problem of insufficient O2 supply because diffusion of atmospheric O2 is limited and, generally, an oxygenated zone of only 0.60–0.80 m is observed (CitationCrossman et al., 2004; CitationKallistova et al., 2005; CitationPerera et al., 2002; CitationTagaris et al., 2003). In other words, improvement of CH4 oxidation in a landfill cover soil depends on continuous O2 supply rather than limited atmospheric diffusion.
Semiaerobic landfill technology was first developed more than 20 years ago in Japan (CitationMatsufuji and Sinha, 1990) and has since been reported to be an effective in situ CH4 emission reduction method. A semiaerobic status is achieved through a continuous supply of fresh air sucked passively through the open end of a leachate collecting pipe (diameter ≥0.6 m), with a temperature gradient created by biodegradation of organic matter. The constant supply of fresh air not only enhances biodegradation and reduces CH4 production but also simultaneously improves leachate quality. It is a proven technology that has been tested in many places in Japan and in a few developing countries, such as Malaysia, Iran, and China (CitationChong et al., 2005). Obviously, a semiaerobic landfill provides more favorable conditions for the placement of an in situ O2 supply into the cover layer than a conventional landfill, and the CH4 oxidation can be expected. Unfortunately, no research has been carried out on the effect of O2 supply to the landfill top cover, so the feasibility of CH4 oxidation improvement in the landfill cover layer by this method remains unknown.
In this paper, a novel passive air diffusion system (PADS) for biocover layer was developed. The CH4 oxidation efficiencies under different simulated landfill gas flow rate were reported. Methanotrophic population structure and activity were also investigated to reveal the effect of O2 on the microbial population. It aims to provide a feasible air supply system without energy consumption in the landfill biocover layer to promote the growth of methanotrophic bacteria, and finally to reduce the CH4 emission from landfill site.
Materials and Methods
Microcosms of simulated biocover
The experimental setups of the simulated biocover microcosms were each made of polyvinyl chloride (PVC) pipes with a size of 0.45 m × 0.38 m (height and inner diameter). The microcosm that was equipped with PADS to enhance the air supply in the column was labeled “macrocosm of simulated novel biocover system” (MSNBS), whereas the macrocosm that simulates conventional biocover system (MSCBS) without PADS was set as the control. A diagram of the macrocosms is shown in As can be seen, the PADS made from Unplasticized-PVC consists of three parts: the bottom drainage pipe (inner diameter = 0.057 m), the vertical venting pipe (inner diameter = 0.046 m, length = 1.000 m), and the air-distributing head wheel (inner diameter = 0.30 m). Whereas the air-distributing head wheel and part of the vertical venting pipe (0.15 m) was placed inside the macrocosm, the rest of the vertical venting pipe and the bottom drainage pipe were kept outside of the macrocosm. The vertical venting pipe outside the macrocosm was heated, and the air temperature in the pipe was kept at 50 ± 1 °C by a heating controller. This was carried out to simulate the microbial self-heating inside the landfill site during organic waste biodegradation. In this setup, the air was sucked in passively through the open ends of the bottom drainage pipe because of the temperature difference between the air inside the vertical pipe and the fresh air outside of the pipe. Subsequently, the drawn-in air was transported upwards through the vertical venting pipe and filled to the macrocosm through the head wheel.
Yard waste compost and topsoil from Beishenshu municipal solid waste (MSW) Landfill (Beiijing, China) were mixed homogeneously at a ratio of 7:3 (w/w). This mixture was used as the material for the simulated biocover macrocosms. The height of the filling material was 0.35 m. Both macrocosms have gas inlet ports at the bottom. Simulated landfill gas (SLFG; CH4 and CO2, 1:1 v/v) was pumped in through the gas inlets at flow rates of 0 (no gas pumped), 10, 20, 40, 60, and 80 mL min−1, respectively, to simulate landfill gas interference in the landfill body right below the biocover layer. At the bottom of the column, a 0.05-m layer of gravel was placed to ensure a homogenous distribution of the gas in the macrocosms. Each macrocosm had seven gas sampling ports with a 0.05-m interval down through the column and an outlet port on the tops.
The landfill gas in the macrocosms was sampled once a day. Each microcosm had eight gas sampling ports with a 0.05-m interval down through the column. The gas samples were collected from these sampling ports with syringes; CH4, O2, and CO2 were analyzed using gas chromatograph (Agilent 6890N; Agilent Technologies, Palo Alto, CA, USA) with a thermal conductivity detector. The detection program consisted of a carrier gas (H2) flow quantity of 30 mL min−1, oven temperature of 120 °C, injector temperature of 160 °C, and detector temperature of 160 °C.
Sampling
Two types of samples were collected from each macrocosm at the end of the experiment for molecular biology analysis of methanotrophs. Samples A1 and B1 represent the bottom layers (10 cm up the gravel of the SLFG-distributing layer) of MSCBS and MSBNS, respectively, and samples A2 and B2 represent the top layers of the MSCBS and MSBNS (10 cm down the top of the biocover material), respectively. Each sample had three replicates.
DNA extraction and PCR
First, DNA was extracted and purified directly from the fresh samples using the FastDNA SPIN Kit for Soil (MP Biotechnology, Solon, OH, USA) according to the manufacturer's instructions. To amplify type I methanotrophs present in the environmental DNA, the primers MethT1bR and MethT1dF () were used, and MethT2R and the bacterium-specific primer 27F were used to amplify type II methanotrophs. Polymerase chain reaction (PCR) was conducted in a thermocycler (TC-3000; High System, Barloworld Scientific, Staffordshire, United Kingdom). The presence and size of amplification products were determined by agarose gel electrophoresis and ethidium bromide staining. For PCR protocols see Supplementary Material.
Table 1. Methanotroph-specific primers and probes
DGGE
A nested-PCR approach was used to profile the type I and type II methanotrophic communities by denaturing gradient gel electrophoresis (DGGE). The PCR-amplification in the above-mentioned steps were used as templates for PCR with the primers GC358F (with a 40-bp GC clamp at the 5' end of 358F) (CitationYan et al., 2011) and 517R (), which span variable region 3 (V3 region) of the 16S rRNA gene. Hot-start and touchdown PCRs were performed to reduce primer-dimer complexes.
The PCR products were analyzed on agarose gels to confirm the presence of a single amplification of expected size. Denaturing gradient electrophoresis gels were 6.5% polyacrylamide with a denaturant gradient from 20% to 70% and 30% to 60% for type I sequences and type II sequences, respectively. Gels were run in 1× Tris‐acetate‐EDTA (TAE) buffer for 12 hr at 100 V at 60 °C and visualized by SYBR-Gold staining.
Specific PCR-DGGE bands were manually excised from the gel, suspended in 50 μL of sterile water, and incubated overnight at 4 °C. The PCR-DGGE protocol was repeated using the above solution as template, until only a single band was detected. A final PCR step was performed without the GC-clamp attached to the forward primer (358F). The resulting PCR products were sent to SinoGenoMax Co. Ltd. (Beijing, China) for purification and nucleotide sequencing.
Sequencing and phylogenetic analysis
Basic Local Alignment Search Tool (BLAST) program was used to search the National Center for Biotechnology Information (NCBI) sequence database (http://www.ncbi.nlm.nih.gov/BLAST/) for sequence similarity. All nucleotide sequences were aligned using the CLUSTAL X program (CitationAymerich et al., 2003). Phylogenetic trees were constructed with the neighbor-joining method using MEGA 5.0 software (http://www.megasoftware.net). Reference sequences were obtained from the GenBank database and were included in the phylogenetic trees for comparison. All nucleotide sequences were optimally aligned prior to tree construction.
Band patterns from PCR-DGGE fingerprints were analyzed using the unweighted pair group method (UPGMA), in which the arithmetic mean used DNAMAN v. 4.1 (Lynnon Biosoft, Vaudreuil, Quebec, Canada). Binary sequences were generated for individual fingerprints by determining band number (R) and position compared with the total number of band positions using Labworks 4.6 software (UVP Products, Upland, CA, USA). The scanned gel images were analyzed using Labworks 4.6.
To evaluate the microbial community in the different height of the macrocosms, richness (R), diversity (H), and dissimilarity (D) indices were calculated from the DGGE band profiles. The Shannon–Weaver index of general diversity (H) was calculated according to the following equation (CitationShannon and Weaver, 1963):
where Pi represents the relative probability of the bands in a gel lane. H is calculated on the basis of the bands on the gel lane that are applied for the generation of the dendrograms by using the intensities of the bands as judged by peak heights in the densitometric curves (CitationAmpe and Miambi, 2000; CitationNübel et al., 1999). Pi is calculated as
where ni refers to the height of a peak and N is the sum of all peak heights in the densitometric curve.
The dissimilarity index (D) is adopted to show the difference among the communities (CitationHiraishi et al., 1991; CitationLuxmy et al., 2000). This is given by
where =
= 1.00, and xik
and xjk
represent the ratio of relative band intensity for samples i and j in the location of band number k. Dissimilarity indices were expressed numerically within a range of 0 (perfectly similar) to 1.00 (completely dissimilar).
Accession numbers for nucleotide sequences
The partial sequences of the 16S rRNA gene of methanotrophs obtained in this study are available from the NCBI database under accession numbers JN166453–JN166465 and JN166487–JN1666496.
Results
CH4 oxidation capacity of biocover equipped with PADS
CH4 oxidation capacity and O2 concentration in MSNBS and MSCBS were compared. Results show that the introduction of PADS in MSNBS significantly increased O2 concentration and improved its distribution in the biocover material either with or without SLFG interference (). In the first stage without SLFG interference, O2 concentration was higher than 18% in different MSNBS layers, whereas those in MSCBS varied within a range of 10–20%. When SLFG interfered in the macrocosms and the flow rate increased from 10 to 80 mL min−1, the O2 content maintained a steady level in MSNBS, where the average O2 concentration detected from each layer was higher than 15%. Those in MSCBS decreased significantly as SLFG flow rate increased, and the average O2 concentration was lower than 10%. More importantly, compared with MSCBS, the O2 concentrations in MSNBS showed less fluctuation during the experimental duration. In other words, the introduction of PADS can significantly change the O2 distribution profile in the biocover matrix.
Figure 2. O2 content in different microcosm layers at different SLFG flow rates. (a) MSCBS; (b) MSNBS.
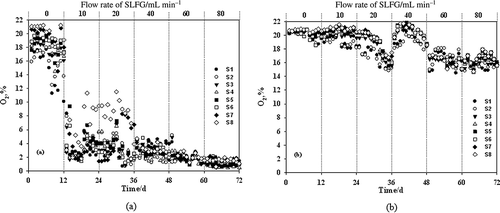
Correspondingly, the CH4 oxidation capacity was significantly higher in MSNBS than that in MSCBS (). In MSNBS, when SLFG flow rate was no more than 40 mL min−1, CH4 could not be detected at the outlet port, indicating a CH4 oxidation rate of 100%. The maximum CH4 oxidation capacity obtained at a flow rate of 40 mL min−1 was 31.34 mol m−3 day−1, which is the highest value generated. The CH4 concentration at the outlet increased slightly (<6%) as SLFG flow rate rose. Those at other layers were no more than 10% even at high SLFG flow rates. The MSCBS CH4 concentration changed drastically with increasing SLFG flow rate, with the highest concentration of 55% observed at the outlet when interfered by SLFG at a flow rate of 80 mL min−1. Those at the other layers were significantly higher than that of MSNBS as well. Similar trends were observed in the CO2 concentration distribution of the two macrocosms. Compared with MSCBS, the CO2 concentration in biocover layers of MSNBS was kept at a lower level with PADS performance. For instance, the highest CO2 concentration was less than 15% when SLFG flow rate reached its highest, whereas that in MSCBS was around 45% ().
Figure 3. CH4 content in different microcosm layers at different SLFG flow rates. (a) MSCBS; (b) MSNBS.
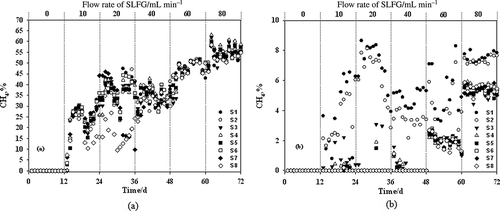
Figure 4. Fingerprints of type I (a) and type II (b) methanotrophic bacterial communities in the microcosms as generated by nested PCR-DGGE (denaturing gradient: 30–60%). Bands designated with an asterisk were excised and subjected to sequencing and identification.
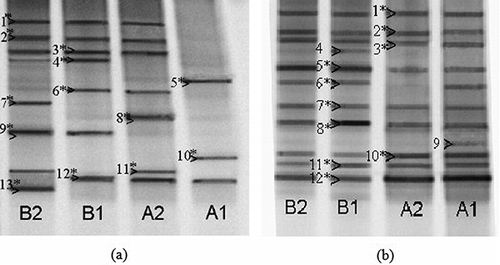
The above results prove that PADS promoted CH4 oxidation by providing sufficient O2 to the biocover system. This system can overcome the problem of limited O2 transportation in biocover layers, thus allowing methanotrophic populations to thrive in the macrocosm.
Numerical analysis of microbial community diversity of methanotrophs
The changes in the methanotrophs community structure in the two macrocosms are investigated and the result is shown in Since each band in the DGGE profiles is likely to be derived from one phylogenetically distinct population, R is equal to the total number of the species in the profile. Thirteen (i.e., R = 13) and 12 (i.e., R = 12) species were obtained for type I and type II methanotrophs, respectively, indicating that there were 13 dominate type I methanotrophs and 12 dominate type II methanotrophs in the samples. For type I methanotrophs, around 10 dominate species (R = 10) were generated with samples from MSNBS, whereas those generated from MSCBS were around 9 (R = 9). For type II methanotrophs, around 11 dominate species (R = 11) were generated with samples from MSNBS, whereas those generated from MSCBS were around 10 (R = 10).
Figure 5. Neighbor-joining tree depicting phylogenetic relationships of type I methanotrophic populations detected in microcosms by nested PCR-DGGE.
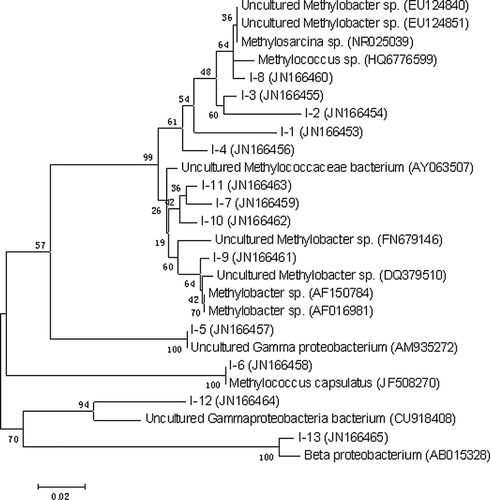
Moreover, the H and D indices, reflecting the structural diversity of the methanotrophic community and the banding-pattern dissimilarity, respectively, were calculated on the basis of the number and relative intensities of bands on the gel track (). Results show that there was a significant difference in microbial diversity between MSNBS and MSCBS for type I methanotrophic bacterial communities. In the MSCBS samples, a significant difference in H value between two layers of the sample (2.04 ≤ H ≤ 2.73) was found, reflecting O2 concentration effect on type I methanotroph diversity. Although there was no such phenomena in MSNBS, widespread occurrence of high populations of type I methanotrophs, as evidenced by a similar H value (2.78 ≤ H ≤ 2.80), indicated that there was sufficient O2 in the entire profile of the system. However, no significant difference of microbial diversity of type II methanotrophic bacterial communities were found among them, as the H value changed in a narrow range (2.84 ≤ H ≤ 2.89). This reflects that O2 concentration in the experiment conditions has little effect on type II methanotroph structure and population density.
Table 2. Comparison of the microbial diversity and dissimilarity of type I and type II methanotrophics between reactors A and B
The band patterns of type I methanotrophs show that there was a lower dissimilarity (D(B1, B2) = 0.26) between the upper and lower MSNBS layers, indicating even O2 distribution in the system as shown in the text above. The dissimilarity was higher (D(A1, A2) = 0.38) in MSCBS, however, reflecting the difference in O2 concentration between the two sampling layers. Low O2 concentration was clearly the limiting factor for type I methanotrophic growth. The band patterns of type II methanotrophs showed lower dissimilarity (0.31 ≤ D ≤ 0.35) in the two macrocosms, confirming that O2 concentration in the study has little influence on type II methanotrophs.
Phylogenetic relationships analysis
Thirteen bands were excised from denaturing gels containing 16S rRNA gene fragments of type I methanotrophic bacteria; these were then sequenced. The detected populations were related to several methanotrophic genera, including Methylobacter, Methylococcus, and Methylosarcina. Among them, Methylobacter were the most prevalent species. Species that were not closely related to any known methanotrophic bacteria were also found in our study, such as band I-5 (JN166457), band I-12 (JN166464), and band I-13 (JN166465) (). The community structure of type I methanotrophs in MSNBS was also found to be different from that in MSCBS. For example, Methylosarcina sp. (band 4, JN166456) and Methylobacter sp. (band 9, JN166461) substituted uncultured γ-proteobacterium (band 5, JN166457) and Methylococcus sp. (band 8, JN166460). Additionally, uncultured Methylococcaceae bacterium (band 7, JN166459) and β-proteobacterium bacterium (band 13, JN166465) were found almost exclusively in MSNBS.
Figure 6. Neighbor-joining tree depicting phylogenetic relationships of type II methanotrophic populations detected in microcosms by nested PCR-DGGE.
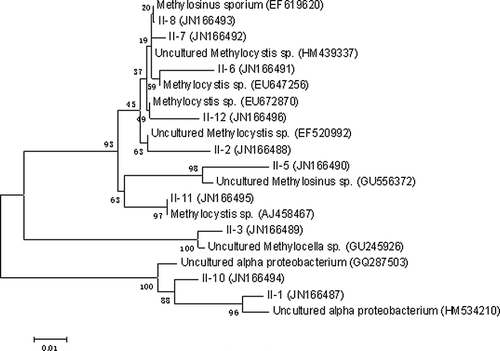
Ten different bands were excised from the denaturing gels containing 16S rRNA gene fragments of type II methanotrophic bacteria; these were then sequenced. The detected populations were related to Methylosinus sp., Methylocystis sp., and Methylocella sp. Among them, Methylocystis sp. was the most predominant species. Populations that were not closely related to any known methanotrophic population were also detected such as band 1(JN166487) and band 10 (JN166494).
Discussion
Since CH4 will continuously produce and release in landfills even at stabilized stage, development of in situ CH4 reduction technology is urgent to alleviate greenhouse effect origin from landfill site. Moreover, in situ oxidation is an optimum choice regardless of whether the landfill site is equipped with gas collection system or not. The above results show that passive air diffusion system has significantly effect on the greenhouse gas emission reduction. And it is technically feasible especially for semiaerobic landfill: the bottom drainage pipes that simultaneously used for air inlet and leachate drainage can be the air supply source, with the function of the air-distributing wheel that connected via the vertical venting pipe, fresh air can be transported and distributed up to the biocover layer thus to increase the O2 concentration nearby. The air-distributing wheel can be substituted by the gravel path during engineering application. An extra advantage of this novel biocover system is to reduce other nocuous gases in the LFG such as gaseous ammonia and sulfur alcohol. Thus alleviate the odors in the landfill site. Demonstration engineering with a size of 1 × 104 m3 has now been carried out by the authors to test the efficiency of PADS at an up-scaled level.
The use of PADS support higher O2 diffusion than the level of atmospheric diffusion reported in other studies (CitationCrossman et al., 2004; CitationKightley et al., 1995). Undoubtedly, PADS became a second O2 source for the biocover in addition to the natural atmospheric diffusion. In this study, methanotrophic populations thrived in MBNBS, since PADS provided sufficient O2 to the whole profiles of it. It is also well documented by another study that improved O2 condition stimulates the growth of aerobic microbes including methanotrophs (CitationLiptay et al., 1998).
The analysis of methanotroph-biased nested PCR-DGGE indicated that sufficient O2 promoted the growth and influenced structure of type I methanotrophs but had little effect on population density as well as community structure of type II methanotrophs, reflecting that O2 is a limiting factor for type I methanotrophs, which grow faster than type II. It also means that type I methanotrophs are more sensitive to the environment. Both type I and type II methanotrophs produce a membrane-bound (particulate) CH4 monooxygenase enzyme (pMMO), which is thought to proliferate under low CH4-to-O2 ratios (CitationAuman and Lidstrom, 2002; CitationCostello et al., 2002; CitationHanson and Hanson, 1996). On the other hand, most type II methanotrophs produce not only the pMMO enzyme but also a more reactive cytoplasmic (soluble) CH4 monooxygenase enzyme (sMMO), which explains the fast acclimation of type II methanotrophs to the environment, and their existence in a wide range of CH4-to-O2 ratios (CitationHanson and Hanson, 1996). CitationZhang et al. (2008) also found that there is a higher abundance of type II than that of type I methanotrophs in fertilization paddy soils.
In this study, the most abundant population among the isolates are Methylobacter (type I) and Methylocystis (type II), which confirmed the results of other studies that demonstrated these two species were extremely abundant in the top layer of landfill covers, as well as the topsoil where O2 was sufficient (CitationBogner et al., 1997; CitationMcdonald and Murrell, 1997; CitationStralis-Pavese et al., 2006; CitationVallaeys et al., 1997; CitationWise et al., 1999).
Studies showed that Methylomonas was an active species in the paddy soils (CitationZheng et al., 2008) besides Methylobacter, that's because this genus is adapted to the condition of high CH4 concentration and low O2, whereas Methylocystis is more frequently detected in a condition with high O2 and low CH4, such as in upland agricultural soil and forestry soil (CitationMei et al., 2011; CitationMohanty et al., 2006). In our system, however, the structure of the dominate species of methanotrophs were different; for instance, species such as Methylomonas and Methylocysitis were not detected, whereas some unknown methanotrophic population were present both for type I and II methanotrophs, indicating the unique condition in MSNBS that provides high concentration of both CH4 and O2.
Landfill is the primary way (>80%) for MSW treatment in China. There are totally more than 400 landfills in China, but only 13 are equipped with LFG extraction and utilization facilities (mostly gas to electricity generation) (Y. F. Nie, personal observation). Landfill gas pollution is thus the key issue of environmental impacts due to direct or fugitive emission. The passive in situ O2 supply that has been proved effective both by CH4 degradation kinetics and microbiological study provide an all-purpose CH4 emission reduction method for the landfills regardless of whether it is equipped with a gas collection system or not. We believe that could be a technically as well as economically feasible approach for the remediation of LFG pollution during MSW landfilling.
Conclusions
1. | The O2 concentration increased in the whole profile of the macrocosms equipped with PADS. The highest CH4 oxidation capacity at 31.34 mol m−3 day−1 was obtain by this system. | ||||
2. | Molecular microbiology analysis showed that the growth of type I methanotrophs was enhanced in the macrocosm because of enhanced air diffusion and distribution; Methylobacter and Methylosarcina were the most prevalent species of type I methanotrophs. | ||||
3. | The microbial diversity and population density of type II methanotrophs were not affected by O2, however, as evidenced by the absence of any difference between the biocover equipped with PADS and that of the control; Methylocystis and Methylosinus were the most prevalent species of type II methanotrophs. |
uawm_a_647236_sup_24065466.docx
Download MS Word (21 KB)Acknowledgments
This research project was funded by the National High Technology Research and Development Program of China (grant no. 2007AA06Z350) and the National Natural Science Foundation of China (grant no. 41101225).
References
- Ampe , F. and Miambi , E. 2000 . Cluster Analysis, Richness and Biodiversity Indexes Derived from Denaturing Gradient Gel Electrophoresis Fingerprints of Bacterial Communities Demonstrate that Traditional Maize Fermentations are Driven by the Transformation Process . International Journal of Food Microbiology , 60 : 91 – 97 .
- Auman , A. and Lidstrom , M. 2002 . Analysis of sMMO-Containing Type I Methanotrophs in Lake Washington Sediment . Environmental Microbiology , 4 : 517 – 524 .
- Aymerich , T. , Martin , B. , Garriga , M. and Hugas , M. 2003 . Microbial Quality and Direct PCR Identification of Lactic Acid Bacteria and Nonpathogenic Staphylococci from Artisanal Low-Acid Sausages . Applied and Environmental Microbiology , 69 : 4583 – 4594 .
- Berger , J. , Fornés , L.V. , Ott , C. , Jager , J. , Wawra , B. and Zanke , U. 2005 . Methane Oxidation in a Landfill Cover with Capillary Barrier . Waste Management , 25 : 369 – 373 .
- Boeckx , P. , Van Cleemput , O. and Villaralvo , I. 1996 . Methane Emission from a Landfill and the Methane Oxidising Capacity of Its Covering Soil . Soil Biology and Biochemistry , 28 : 1397 – 1405 .
- Bogner , J.E. , Spokas , K.A. and Burton , E.A. 1997 . Kinetics of Methane Oxidation in Landfill Cover Materials: Major Controls, a Whole Landfill Oxidation Experiment, and Modeling of Net Methane Emissions . Environmental Science and Technology , 31 : 2504 – 2514 .
- Chanton , J.P. , Rutkowski , C.M. and Mosher , B.M. 1999 . Quantifying Methane Oxidation from Landfills Using Stable Isotope Analysis of Downwind Plumes . Environmental Science and Technology , 33 : 3755 – 3760 .
- Chong , T.L. , Matsufuji , Y. and Hassan , M.N. 2005 . Implementation of the Semi-Aerobic Landfill System (Fukuoka Method) in Developing Countries: A Malaysia Cost Analysis . Waste Management , 25 : 702 – 711 .
- Christophersen , M. , Linderød , L. , Jensen , P.E. and Kjeldsen , P. 2000 . Methane Oxidation at Low Temperatures in Soil Exposed to Landfill Gas . Journal of Environmental Quality , 29 : 1989 – 1997 .
- Costello , A.M. , Auman , A.J. , Macalady , J.L. , Scow , K.M. and Lidstrom , M.E. 2002 . Estimation of Methanotroph Abundance in a Freshwater Lake Sediment . Environmental Microbiology , 4 : 433 – 450 .
- Crossman , Z.M. , Abraham , F. and Evershed , R.P. 2004 . Stable Isotope Pulse-Chasing and Compound Specific Stable Carbon Isotope Analysis of Phospholipid Fatty Acids to Assess Methane Oxidizing Bacterial Populations in Landfill Cover Soils . Environmental Science and Technology , 38 : 1359 – 1367 .
- Czepiel , P. , Mosher , B. , Crill , P.M. and Harriss , R. 1996 . Quantifying the Effect of Oxidation on Landfill CH4 Emissions . Journal of Geophysical Research , 101 : 16721 – 16729 .
- Hanson , R.S. and Hanson , T.E. 1996 . Methanotrophic Bacteria . Microbiology Reviews , 60 : 439 – 471 .
- Haubrichs , R. and Widmann , R. 2006 . Evaluation of Aerated Biofilter Systems for Microbial Methane Oxidation of Poor Landfill Gas . Waste Management , 26 : 408 – 416 .
- Hiraishi , A. , Morishima , Y. and Taeuchi , J. 1991 . Numerical Analysis of Lipoquinone Patterns in Monitoring Bacterial Community Dynamics in Wastewater Treatment Systems . Journal of General and Applied Microbiology , 37 : 57 – 70 .
- IPCC . “ Methane: Tapping the Untapped Potential. The Fourth Assessment Report (AR4) ” . In Climate Change 2007: Synthesis Report Core Writing Team , Edited by: Pachauri , R.K. and Reisinger , A. Cambridge , , UK : Cambridge University Press .
- Kallistova , A. , Kevbrina , M. , Nekrasova , V. , Glagolev , M. , Serebryanaya , M. and Nozhevnikova , A. 2005 . Methane Oxidation in Landfill Cover Soil . Microbiology , 74 : 608 – 614 .
- Kightley , D. , Nedwell , D.B. and Cooper , M. 1995 . Capacity for Methane Oxidation in Landfill Cover Soils Measured in Laboratory-Scale Soil Microcosms . Applied and Environmental Microbiology , 61 : 592 – 610 .
- Lee , S.W. 2008 . Microbial Mitigation of Greenhouse Gas Emissions from Landfill Cover Soils. Ph.D. Dissertation , Ann Arbor , MI : University of Michigan .
- Liptay , K. , Chanton , J. , Czepiel , P. and Mosher , B. 1998 . Use of Stable Isotopes to Determine Methane Oxidation in Landfill Cover Soils . Journal of Geophysical Research , 10 ( 3 ) : 8243 – 8250 .
- Luxmy , B.S. , Nakajima , F. and Yamamoto , K. 2000 . Analysis of Bacterial Community in Membrane-Separation Bioreactors by Fluorescent in Situ Hybridization (FISH) and Denaturing Gradient Gel Electrophoresis (DGGE) Techniques . Water Science and Technology , 41 : 259 – 268 .
- Matsufuji , Y. and Sinha , K. 1990 . “ Landfill Site Improvement in Design for Sanitary Landfill in Malaysia ” . In Hazardous Waste Regulations and Management , Edited by: Tong , S.L. , Hamid , A.A. and Lee , K. 175 – 200 . Kuala Lumpur : ENSEARCH .
- Mcdonald , I.R. and Murrell , J.C. 1997 . The Methanol Dehydrogenase Structural Gene MxaF and Its Use as a Functional Gene Probe for Methanotrophs and Methylotrophs . Applied and Environmental Microbiology , 63 : 3218 – 3224 .
- Mei , J. , Wang , L. , Han , D. and Zhao , Y.C. 2011 . Methanotrophic Community Structure of Aged Refuse and Its Capability for Methane Bio-Oxidation . Journal of Environmental Science , 23 : 868 – 874 .
- Mohanty , S.R. , Bodelier , P.L.E. , Floris , V. and Conrad , R. 2006 . Differential Effects of Nitrogenous Fertilizers on Methane-Consuming Microbes in Rice Field and Forest Soils . Applied and Environmental Microbiology , 72 : 1346 – 1354 .
- Nikiema , J. , Brzezinski , R. and Heitz , M. 2007 . Elimination of Methane Generated from Landfills by Biofiltration: A Review . Reviews in Environmental Science and Biotechnology , 6 : 261 – 284 .
- Nübel , U. , Garcia-Pichel , F. , ühl , M. K and Muyzer , G. 1999 . Quantifying Microbial Diversity: Morphotypes, 16S rRNA Genes, and Carotenoids of Oxygenic Phototrophs in Microbial Mats . Applied and Environmental Microbiology , 65 : 422 – 430 .
- Parker , T. , Dottridge , J. and Kelly , S. 2002 . Investigation of the Composition and Emissions of Trace Components in Landfill Gas , 145 Bristol , , UK : Environment Agency. p . R&D Technical Report P1–438/TR
- Perera , L. , Achari , G. and Hettiaratchi , J. 2002 . Determination of Source Strength of Landfill Gas: A Numerical Modeling Approach . Journal of Environmental Engineering , 128 : 461 – 471 .
- Rahalkar , M. , Bussmann , I. and Schink , B. 2007 . Methylosoma Difficile Gen. Nov., sp nov., A Novel Methanotroph Enriched by Gradient Cultivation from Littoral 137 Sediment of Lake Constance . International Journal of Systematic and Evolutionary Microbiology , 57 : 1073 – 1080 .
- State Environmental Protection Administration of China . 2009 . 2006 Report on the State of the Environment in China
- Scheutz , C. , Kjeldsen , P. , Bogner , J. , DeVisscher , A. , Gebert , J. , Hilger , H. , Huber-Humer , M. and Spokas , K.A. 2009 . Microbial Methane Oxidation Processes and Technologies for Mitigation of Landfill Gas Emissions . Waste Management and Research , 27 : 409 – 455 .
- Shannon , C.E. and Weaver , W. 1963 . The Mathematical Theory of Communication , Urbana and Chicago , IL : University of Illinois Press .
- Stern , D.I. and Kaufman , R.K. 1996 . Estimates of Global Anthropogenic Methane Emission 1860–1993 . Chemosphere , 33 : 159 – 176 .
- Stralis-Pavese , N. , Bodrossy , L. , Reichenauer , T.G. , Weilharter , A. and Sessitsch , A. 2006 . 16S rRNA Based T-RFLP Analysis of Methane Oxidizing Bacteria: Assessment, Critical Evaluation of Methodology Performance and Application for Landfill Site Cover Soils . Applied Soil Ecology , 31 : 251 – 266 .
- Streese , J. and Stegmann , R. 2003 . Microbial Oxidation of Methane from Old Landfills in Biofilters . Waste Management , 23 : 573 – 580 .
- Tagaris , E. , Sotiropoulou , R.E. , Pilinis , C. and Halvadakis , C. 2003 . A Methodology to Estimate Odors Around Landfill Sites: The Use of Methane as an Odor Index and Its Utility in Landfill Sitting . Journal of the Air and Waste Management , 53 : 629 – 634 .
- Themelis , N.J. and Ulloa , P.A. 2007 . Methane Generation in Landfills . Renewable Energy , 32 : 1243 – 1257 .
- Vallaeys , T. , Topp , E. , Muyzer , G. , Macheret , V. , Laguerre , G. , Rigaud , A. and Soulas , G. 1997 . Evaluation of Denaturing Gradient Gel Electrophoresis in the Detection of 16S rDNA Sequence Variation in Rhizobia and Methanotrophs . FEMS Microbiology Ecology , 24 : 279 – 285 .
- Whalen , S.C. , Reeburgh , W.S. and Sandbeck , K.A. 1990 . Rapid Methane Oxidation in a Landfill Cover Soil . Applied and Environmental Microbiology , 56 : 3405 – 3411 .
- Wise , M.G. , McArthur , J.V. and Shimkets , L.J. 1999 . Methanotroph Diversity in Landfill Soil: Isolation of Novel Type I and Type II Methanotrophs whose Presence was Suggested by Culture-Independent 16S Ribosomal DNA Analysis . Applied and Environmental Microbiology , 65 : 4887 – 4897 .
- Yan , Q.M. , Zhang , X.X. , Zhang , T. and Fang , H.H.P. 2011 . Seasonal Microbial Community Shift in a Saline Sewage Treatment Plant . Frontiers of Environmental Science and Engineering in China , 5 : 40 – 47 .
- Zheng , Y. , Zhang , L.M. , Zheng , Y.M. , Di , H.J. and He , J.Z. 2008 . Abundance and Community Composition of Methanotrophs in a Chinese Paddy Soil Under Long-Term Fertilization Practices . Journal of Soil, Sediment , 8 : 406 – 414 .