ABSTRACT
The coronavirus helicase is an essential enzyme required for viral replication/transcription pathways. Structural studies revealed a sulphate moiety that interacts with key residues within the nucleotide-binding site of the helicase. Compounds with a sulphoxide or a sulphone moiety could interfere with these interactions and consequently inhibit the enzyme. The molecular operating environment (MOE) was used to dock 189 sulphoxide and sulphone-containing FDA-approved compounds to the nucleotide-binding site. Zafirlukast, a leukotriene receptor antagonist used to treat chronic asthma, achieved the lowest docking score at −8.75 kcals/mol. The inhibitory effect of the compounds on the SARS-CoV-2 helicase dsDNA unwinding activity was tested by a FRET-based assay. Zafirlukast was the only compound to inhibit the enzyme (IC50 = 16.3 µM). The treatment of Vero E6 cells with 25 µM zafirlukast prior to SARS-CoV-2 infection decreased the cytopathic effects of SARS-CoV-2 significantly. These results suggest that zafirlukast alleviates SARS-CoV-2 pathogenicity by inhibiting the viral helicase and impairing the viral replication/transcription pathway. Zafirlukast could be clinically developed as a new antiviral treatment for SARS-CoV-2 and other coronavirus diseases. This discovery is based on molecular modelling, in vitro inhibition of the SARS-CoV helicase activity and cell-based SARS-CoV-2 viral replication.
Introduction
In the last two decades, coronaviruses have caused three major global outbreaks: the Severe Acute Respiratory Syndrome Coronavirus (SARS-CoV) in (2003) [Citation1,Citation2], the Middle East Respiratory Syndrome Coronavirus (MERS-CoV) in (2012) [Citation3,Citation4], and the Severe Acute Respiratory Syndrome Coronavirus 2 (SARS-CoV-2) in 2019 [Citation5]. The symptoms of SARS-CoV-2 include shortness of breath, cough, and fever. In severe cases, the infection could lead to dyspnoea, pneumonia, kidney failure, and even death [Citation6]. SARS-CoV-2 is a positive RNA virus that belongs to the Betacoronavirus genus of the Coronaviridae family in the order Nidovirales [Citation7]. The genome of SARS-CoV-2 is composed of 14 open reading frames that encode 4 structural proteins, 16 nonstructural proteins (nsps) and several accessory proteins [Citation8]. The entry of SARS-CoV-2 in the host cell is initiated by binding the viral spike protein (S1) to the cellular receptor angiotensin-converting enzyme 2 (ACE2). Viral fusion to the host cell endosome is promoted by the cellular surface serine protease (TMPRSS2). After entry and release of the viral genomic RNA, two large open reading frames, ORF1a and ORF1b are translated producing viral polyproteins, pp1a and pp1ab, which in turn are processed by viral proteases into several non-structural proteins essential for the replication/transcription complex formation. Finally, new virions form by the assembly of translated structural proteins with the newly synthesized viral genomic RNA and secreted out of the infected cell by exocytosis [Citation9].
Since the outbreak in Wuhan province, China, drug discovery efforts were focused on finding new antiviral drugs that prevent the spread of SARS-CoV-2 and alleviate the severity of its symptoms in infected individuals [Citation10,Citation11]. Novel drug development processes can take many years, due to the stages of clinical investigation before final approval as a therapeutic agent [Citation12,Citation13]. Due the urgency of the current COVID-19 epidemic, a significant share of drug discovery research was geared towards drug repurposing [Citation14,Citation15]. Finding new anti-SARS-CoV-2 indications of a previously developed therapeutic agent that has passed the long processes of preclinical experiments and safety assessments can significantly speed up the efforts of finding an effective treatment for SARS-CoV-2 [Citation11,Citation16]. Remdesivir, the only antiviral drug approved by the Food and Drug Administration (FDA) to treat SARS-CoV-2 [Citation10], is an example of a repurposed drug which was initially developed through a collaboration between Gilead Sciences, the U.S. Army Medical Research Institute of Infectious Diseases (USAMRIID) and the U.S. Centres for Disease Control and Prevention (CDC), as an antiviral agent against RNA viruses that could potentially cause a global epidemic, including Ebola virus (EBOV), MERS-CoV and SARS and lately repositioned to treat SARS-CoV-2 [Citation17]. Drug discovery strategies, de novo drug development and drug repurposing, relied on integrating two screening methods, including high throughput screening and structure based virtual screening [Citation11,Citation18]. Many of the assay methods used to measure the SARS-CoV-2 proteins and enzymes functionalities are based on previously developed methods used to measure the activities in other pathogenic viruses, particularly coronaviruses [Citation19]. The high sequence identity between SARS-CoV-2 and other human pathogenic coronaviruses, 82% in the case of SARS-CoV, and the identification of several highly conserved regions coding for proteins and enzymes essential for virus replication in all pathogenic coronaviruses, including SARS-CoV and MERS-CoV [Citation20–22] combined with comparative homology modelling of high resolution crystal structures of viral proteins enabled the use of structure based virtual screening to predict new ligands that can bind to different SARS-CoV-2 proteins [Citation23]. The importance of the structure based virtual screening approach significantly increased with the direct of use rapidly growing SARS-CoV-2 structural data and the continuous release of three dimensional structures of SARS-CoV-2 structural, nonstructural and accessory proteins [Citation24–26].
Helicases are essential enzymes that hydrolyse ATP to separate double-stranded (ds) nucleic acid into two single-stranded nucleic acids (ss) [Citation27]. Helicases are found in all life kingdoms and many viruses [Citation28]. DNA helicases are essential for DNA replication, recombination, and repair [Citation29]. RNA helicases, on the other hand, are essential for RNA transcription, mRNA splicing, mRNA export, and translation [Citation30]. Both DNA and RNA helicases are found in the human genome [Citation31]. On a molecular level, helicases play a central role in many processes such as protein–nucleic acid complex dissociation [Citation32], Holliday junction displacement [Citation33], and chromatin remodelling [Citation34]. The SARS-CoV-2 genome is composed of several open reading frames (ORFs). Located at 5’ end of the genome, the ORF1ab is translated in the host cell directly after infection. During translation, a ribosomal frame shift of −1 results in the synthesis of two large polypeptides: pp1a and pp1b. These two polypeptides undergo proteolytic cleavage by viral papain-like protease (PLpro) and 3 C-like protease (3CLpro) producing 16 nonstructural proteins (nsps) [Citation35]. The nsps assemble into membrane-associated replication-transcription complexes (RTCs). These complexes are responsible for SARS-CoV-2 genome replication. The coronavirus helicase (nsp13) is a pivotal component of the RTCs [Citation36].
The helicase can be considered as a possible target for antiviral agents since it is one of the most conserved nonstructural proteins in all the pathogenic coronaviruses [Citation37]. The helicases of SARS-CoV-2, MERS-CoV and SARS-CoV share 84% to 99% sequence similarity [Citation38]. The coronavirus helicase (nsp13) is a 5′–3′ direction ATP-dependent helicase that separates double-stranded nucleic acids (ds) into two single-stranded nucleic acids (ss) [Citation39,Citation40]. Crystal structures of the helicases of SARS-CoV, MERS-CoV and SARS-CoV-2 show a similar structural organization. The coronavirus helicase is a multi-domain protein, consisting of the unwinding activity associated domain 1A, the 2A domain, the 1B domain, the stalk domain, and the zinc-binding domain [Citation41–43]. Virtual screening of homology-based models of the SARS-CoV-2 helicase predicted the binding of several approved human drugs to the nucleotide-binding pocket [Citation44]. Cepharanthine was shown to possibly interact with the residues G285, G287, S289, A313, A316, K320, E375, E540, R442, R443, Q537, G538 within the SARS-CoV-2 helicase-binding pocket [Citation45]. In another study, simeprevir, paritaprevir, and grazoprevir possibly interacted with several common residues in the SARS-CoV-2 helicase nucleotide binding site, including T286, G287, K288, S289, Q404, R443, G538, E540, R567 [Citation40]. In addition, three compounds possibly interacted with residues K288, S289, D374, E375, Q404 and R567 of SARS-CoV-2 helicase [Citation46]. The RNA-binding of SARS-CoV-2 helicase interface is also a possible binding site for several inhibitors, including imatinib [Citation47]. Ivermectin also possibly interacts with residues R178, N179, K202, and T532 of SARS-CoV-2 helicase [Citation48]. Finally, the N-terminal zinc-binding domain of SARS-CoV-2 helicase is also identified as a possible binding site for inhibitors [Citation49].
In addition to virtual screening, several SARS-CoV inhibitors were detected through a Fluorescence Resonance Energy Transfer (FRET)-based high throughput assay, including myricetin and scutellarein [Citation50]. The compounds, SSYA10-001 and SSYA10-002, inhibit the SARS-CoV helicase [Citation51], and the compound SSYA10-001 inhibits the MERS-CoV helicase [Citation52]. Triazole derivatives were reported to inhibit the MERS-CoV helicase. Molecular docking attributed the inhibitory action of triazole derivatives to their interaction with residues Y7, Y159, R 163, and Y174 in the active site [Citation53]. Using a FRET-based high throughput assay, our laboratory identified several MERS-CoV helicase inhibitors of different potency levels. Docking of these inhibitors in the nucleotide-binding site revealed possible interactions with several residues including G258, G285, K288, E319, K323, E375, R443, G538, E540 and R567 [Citation54]. In a previous structural study, a sulphate ion was located within the nucleotide-binding site of MERS-CoV helicase. Although it is difficult to conclude whether this sulphate ion is an intrinsic part of the protein’s atomic structure or a solution component, it appears to interact specifically and is stabilized through hydrogen bonding with the key amino acids Q404, R443 and R567, in the vicinity of the nucleotide-binding site. It has been suggested that this ion is essential for NTP hydrolysis [Citation49]. Compounds with a sulphoxide or sulphone moiety could establish similar interactions with these amino acids and eventually affect the enzyme activity. The aim of the current study was to virtually screen the binding of sulphoxide and sulphone-containing FDA-approved compounds to the nucleotide-binding site of the SARS-CoV-2 helicase, as well as to test the effects of the compounds on the SARS-CoV-2 helicase unwinding activity of dsDNA, using an FRET-based assay.
Materials and methods
SARS-CoV-2 helicase nucleotide-binding site modelling
The recently published crystal structures of the SARS-CoV-2 helicase are fragmented atomic structures. The fragmentation pattern of these structures makes it difficult to locate a binding site suitable for in-silico docking simulations [Citation45]. The MERS-CoV atomic structure (PDB_ID: 5WWP), has a well-identified nucleotide-binding site [Citation49]. Since the nucleotide-binding domains of the three pathogenic coronaviruses are highly conserved, as shown by the UniProt alignment program (http://www.uniprot.org/align/), it is assumed that the three-dimensional structures of these binding sites are also similar. The nucleotide-binding site in the SARS-CoV-2 helicase was located by structural superimposition of the fragmented SARS-CoV-2 helicase (PDB_ID: 5RL9) atomic structure with the MERS-CoV full atomic structure (PDB_ID: 5WWP). MOE (MOE, 2019.0102; Chemical Computing Group ULC, Canada). It was used to perform the docking of 189 compounds with the sulphoxide or sulphone moiety containing FDA molecules into the nucleotide binding pocket of SARS-CoV-2 helicase at pH 7.4. The docking scores and the ligand–amino acid interactions are used to obtain a reasonable subset of molecules for enzyme assay testing. The sulphoxide or sulphone-containing compounds docked into the nucleotide-binding pocket of the SARS-CoV-2 helicase, while allowing a 9 Å radius around the binding pocket to flex during the docking process. The molecules were allowed 10 final poses, which interacted with the essential amino acids. The docked poses with one or more interactions with one of the essential amino acids: Q404, R442, R443, and R567 were included in the final set. The final set of molecules containing an interaction with one or more essential amino acids has been categorized as potentially good inhibitors.
SARS-CoV-2 helicase expression and purification
The SARS-CoV-2 helicase bacterial expression plasmid was synthesized and cloned by GeneScript. The nsp13-cDNA was cloned in the pET-52b(+) vector with a Strep-tag at its N-terminus in the cloning site SmaI/SacI. The DNA substrate fork was made of black hole quencher (BHQ) modified oligonucleotides: 5′TCACCACCACGTATCTGAGCCTGGGCGA(BHQ)-3′ and fluorescein modified oligonucleotide: 5′(FLUORESCEIN)TCGCCCAGGCTCAGATACGACCACCACT-3′. The DNA substrate fork was made of 5′-UCACCACCACGUAUCUGAGCCUGGGCGA(BHQ)-3′ and 5′(FLUORESCEIN)UCGCCCAGGCUCAGAUACGACCACCACU-3′oligonucleotides. All oligonucleotides were purchased from Integrated DNA Technologies (Coralville, IA). The SARS-CoV-2 nsp3 was expressed and purified as described previously [Citation51]. In brief,: pET-52b-nsp13 plasmids were transformed into BL21 (DE3) cells. The cells were grown overnight at 37°C in starter non-inducing media: 50 mM Na2HPO4, 50 mM NH4Cl, 5 mM Na2SO4, 2 mM MgSO4.7H2O, 10 μM FeCl3.6H3O, 4 μM CaCl2, 2 μM Mn3.4H3O, 2 μM ZnSO4.7H3O, 0.4 μM CoCl2.6H3O, 0.4 μM CuCl2.2H3O, 0.4 μM NiCl2.6H3O, 0.4 μM Na2MoO4.5H3O, Na2SeO3.5H3O, 0.4 μM H3BO3, 0.5% glucose, 0.25% aspartate, and 100 μg/ml ampicillin. The overnight grown cells are transferred at a 1:1000 dilution into expression ZYM-5052 auto-inducing media: 10 g/L N-Z amine, 5 g/L yeast extract, 50 mM Na2HPO4, 50 mM NH4Cl, 5 mM Na2SO4, 2 mM MgSO4.7H2O, 10 μM FeCl3.6H3O, 4 μM CaCl2, 2 μM Mn3.4H3O, 2 μM ZnSO4.7H3O, 0.4 μM CoCl2.6H3O, 0.4 μM CuCl2.2H3O, 0.4 μM NiCl2.6H3O, 0.4 μM Na2MoO4.5H3O, Na2SeO3.5H3O, 0.4 μM H3BO3, 0.5% glycerol, 0.05% glucose, 0.2% alpha-lactose, and 100 μg/ml ampicillin. The cells are left to grow at 37°C while shaking at 210 rpm for 5 h until the solution is turbid. The temperature was lowered to 20°C and shaken overnight until the OD600 plateau. The cells were harvested by centrifugation at 4000 × g for 10 min. The cells were washed once with a lysis buffer: 100 mM Tris-HCl, pH 8.0, 150 mM NaCl, 5% glycerol, 0.1% Triton X, 1 M DTT, 0.15 mg/ml lysozyme, and 1 mM phenylmethylsulfonyl fluoride (PMSF). The pellet was resuspended in a 100 ml lysis buffer. The cell suspension was sonicated on ice at 75% amplitude for 15:15 s pulse for 2 min. The cell lysate was centrifuged at 17,500 × g for 45 min. The supernatant fluid was applied to a Strep-Tactin column previously equilibrated with two volumes of washing buffer: 100 mM Tris-HCl, pH 8.0, 150 mM NaCl, 5% glycerol, 0.1% Triton X, and 1 M DTT. After all the cell lysate passed through the column, the column was washed 5 times with 1 column volume of washing buffer and eluted with elution buffer: 100 mM Tris-HCl, pH 8.0, 150 mM NaCl, 5% glycerol, 0.1% Triton X, and 1 M DTT, and 10 mM desthiobiotin. The eluate was dialysed overnight in the washing buffer. Helicase containing fractions were concentrated and stored at −80°C.
Assay of SARS-CoV-2 helicase ATPase activity
Helicase activity was monitored throughout the purification procedure. Enzyme activity was detected by measuring ATPase activity in the samples using the E. coli DNA Helicase ATPase Assay Kit (ProFoldin, Hudson, MA). Briefly,: 20 nM helicase is incubated with 250 nM dsDNA and 0.25 mM ATP in a reaction buffer containing 20 mM HEPES, pH 7.5, 20 mM potassium glutamate, 1 mM DTT, 0.005% Triton X-100, 10 mM MgCl2 at room temperature for 60 min in total volume, 30 µl. The released inorganic phosphate was detected by the addition of 45 µl detection dye to the reaction mixture in a 1:1.5 ratio. After 5 min incubation, the absorbance of the solution was measured at 650 nm. The positive control (E. coli helicase) is provided with the kit. The protein concentration was measured with Bradford’s assay reagent, and the SDS-PAGE was used to determine the purity of the sample.
Assay of SARS-CoV-2 helicase activity
The FRET-based screening assay was conducted in a 96-well plate platform as described previously [Citation54]. Briefly, the helicase dsDNA substrate was prepared by mixing a fluorescein-labelled oligonucleotide with a black hole quencher (BHQ)-labelled oligonucleotide at a 1:1.2 ratio in 50 mM Tris, NaCl 50 mM, pH 8.0 in a total volume 150 µl. The mixture was first heated to 95°C for 5 min and then cooled slowly to room temperature to allow annealing. The compounds of the FDA-approved library of compounds (Tocris Biosciences, Bristol, United Kingdom) were lyophilized and added to the 96-well plates. The source plates were prepared by dissolving the compounds in 100% v/v dimethyl sulphoxide (DMSO) to a final concentration of 10 mM. Each compound was assayed at a 100 µM final concentration by adding 1 µl of each compound from the source plate (10 mM) to the final assay volume of 100 µl. For subsequent assays, the sub-plates of several factor dilutions (in 100% DMSO v/v) were prepared from the source plates. All of the plates were stored at – 80°C. The change in fluorescence due to helicase activity was measured in black 96-well flat-bottom corning plates. The final concentration of the assay solution in 100 µl volume: 20 mM HEPES, 20 mM NaCl, 5 mM MgCl2, 0.01% bovine serum albumin (BSA), 2 mM DTT, 5% glycerol, 50 nM helicase, 0.5 mM ATP, 75 nM fluorescein- and black hole quencher-labelled dsDNA, 2 µM trap, unlabelled single-stranded DNA complementary to the BHQ-labelled DNA strand, and different final concentrations of inhibitors. A volume of 1 µl of different concentrations of the inhibitors was preincubated with 50 µl enzyme solution of the assay buffer: 100 nM helicase, 1 mM ATP, and 4 µM trap at room temperature for 5 min, and the reactions were initiated by adding 50 µl of assay buffer containing 150 nM labelled dsDNA. The reactions were allowed to continue for 10 min at 30°C. The samples were excited at a wavelength of 495 nm and fluorescence emission was measured in microfluor 2 black U-bottom 96-well plates (Fisher Scientific) at a wavelength of 520 nm using a plate-reader (SpectraMax M5, Molecular Devices, USA).
SARS-CoV-2 helicase kinetics and IC50 determinations
To determine the Km values for the DNA and ATP, the helicase activity was measured by the FRET-based assay, as previously mentioned, with various concentrations of dsDNA: 0 nM, 5 nM, 10 nM, 20 nM, 40 nM, 80 nM and 160 nM. The dsDNA substrate was prepared by annealing a fluorescein-labelled oligonucleotide with a black hole quencher as previously mentioned. To determine the Km values for the ATP, the helicase activity was measured with the FRET-based assay as previously mentioned, with various concentrations of ATP (0 mM, 0.25 mM, 0.5 mM, 1.5 mM, 3 mM, and 6 mM) using dsDNA as the second substrate. Data were fitted to the Michaelis–Menten curve using GraphPad Prism. IC50 determinations for the inhibition of helicase activity were performed using varying concentrations of each inhibitor: 0 µM, 1 × 10−3 µM, 1.7 × 10−3 µM, 5.1 × 10−3 µM, 15.2 × 10−3 µM, 45.7 × 10−3 µM, 0.1372 µM, 0.4115 µM, 1.234 µM, 3.704 µM, 11.11 µM, 33.33 µM, and 100 µM. The data were fitted to a 4-parameter logistic fit for the dose–response curves using GraphPad Prism.
Vero cell viability and infection with SARS-CoV-2
Vero E6 cells at 10,000 cells/µL density in phenol-free Dulbecco’s modified Eagle’s medium (DMEM) supplemented with 10% foetal bovine serum (FBS) were plated in a white flat bottom 96-well plate (Co-Star Cat#3917) containing 1 µL compound in DMSO at concentrations: 0 µM, 1 µM, 10 µM, 25 µM, 50 µM, 100 µM. The cells were then incubated for 24 h at 37°C and 5% CO2. The next day, the cells in each well were lysed by the addition of 50 µL of CellTiter‐Glo luminescent cell viability assay reagent (Promega, Durham, NC, USA) and incubation for 15 min in the dark. The luminescence was then read using the Envision plate reader (Perkin Elmer, Waltham, MA, USA). The infection assay of Vero E6 cells using the SAR-CoV-2 named SARS-CoV2/human/SAU/85791 C/(2020) (Gene accession number MT630432.1) was conducted as previously described [Citation55]. Briefly, the Vero E6 cells in 6-well clusters were infected with serial dilutions of SARS-CoV-2 in PBS containing DEAE (0.005% w/v) and 2% FCS and were incubated at 37°C for 1 h. Subsequently, the inoculations were replaced with 2 ml of a 1.2% suspension of Avicel (RC-581; FMC Biopolymer) in DMEM containing 2% FBS, 25 mM HEPES, penicillin (100 IU/ml) and streptomycin (100 IU/ml). The cells were incubated at 37°C for 48 hours and fixed with formaldehyde, the infected cells were assessed by microscopy. For compound testing, the compounds were added to the virus aliquot to give the indicated final concentration. The final DMSO concentration was less than 0.1% in each assay and in the control experiment, DMSO alone was added. All experiments involved live SARS-CoV-2 were performed following the international recommended safety measures and precautions in Biosafety Level 3 Facility at the Special Infectious Agent Unit, King Fahd Medical Research Centre, King Abdulaziz University, Saudi Arabia.
Quantifying SARS-CoV-2 mRNA within infected Vero E6 cells by qPCR
The amount of SARS-CoV-2 mRNA in the infected Vero E6 cells, in the presence or absence of compounds, was determined by Real-time RT-using PowerChek™ 2019-nCoV Real-time PCR Kit (Cat No. R6900TD). The kit was utilized according to the manufacturer’s instructions to target the RdRp gene of 2019-nCoV as previously described [Citation55]. The ExiPrep™ 96 Viral DNA/RNA Kit (BioNEER Corp.) was used to extract the SARS-CoV-2 RNA from the infected Vero E6 cells and used in qPCR. The level of the SARV-CoV-2 mRNA was converted to cycle threshold (CT) values.
Results
Virtual screening of inhibitors in the SARS-CoV-2 helicase nucleotide binding site
The nucleotide-binding site in the SARS-CoV-2 helicase was located by the structural superimposition of the SARS-CoV-2 helicase (PDB_5RL9) with the MERS-CoV helicase (PDB_5WWP). The spatial position of amino acid R443 in MERS-CoV, an essential residue for sulphate ion stabilization, matched the position of the corresponding amino acids in the SARS-CoV-2 helicase – see . The effect of the sulphate moiety on the docking of the molecules to the SARS-CoV-2 helicase was investigated. The ligands and the binding pocket are kept fully flexible during this procedure. Of the 1953 FDA-approved compounds, 189 compounds containing a sulphoxide or sulphone moiety were selected for virtual screening – see Table S1. Sulphoxide and sulphone-containing molecules were conformationally docked into the nucleotide-binding pocket of the SARS-CoV-2 helicase at pH 7.4, using the molecular operating environment (MOE). Ten final poses were allowed for each molecule while maintaining the active site flexible at 9 Å from the ligand. The molecules were prioritized according to the number of poses showing one or more interactions with the one or more essential amino acids Q404, R442, R443, and R567 – see . The lowest docking score was measured for zafirlukast. Zafirlukast’s sulphate moiety showed multiple interactions with two essential amino acids in the nucleotide-binding site – see ). The sulphoxide moiety of lapatinib showed multiple interactions with three essential amino acids in the nucleotide-binding site; however, its docking score is relatively high compared to other molecules. The compounds mezlocillin sodium, bosentan hydrate, sivelestat sodium tetrahydrate and omipalisib (GSK458) established one interaction between their sulphoxide or sulphone moieties and essential residues at the binding site. The sulphoxide moieties of darunavir, tianeptine sodium and pazopanib HCl did not establish any interaction with any essential residue. Montelukast, a leukotriene receptor antagonist used as an alternative to zafirlukast to treat asthma [Citation56] was docked at the nucleotide-binding site even though it lacks a sulphoxide or sulphone moiety, to determine if its action is achieved by binding to the helicase nucleotide-binding site. Montelukast established several stable interactions at the binding site. One interaction was with an essential residue – see and Figure S1.
Table 1. Docking scores and interactions of high priority molecules with SARS-CoV-2 nucleotide-binding site
Figure 1. Superimposition of the atomic structure of SARS-CoV-2 helicase (yellow; PDB_ID:5RL9) and atomic structure of MERS-CoV helicase (magenta; PDB_ID:5WWP). Nucleotide binding site of both structures was identified by residue R443 (blue) of MERS-CoV helicase
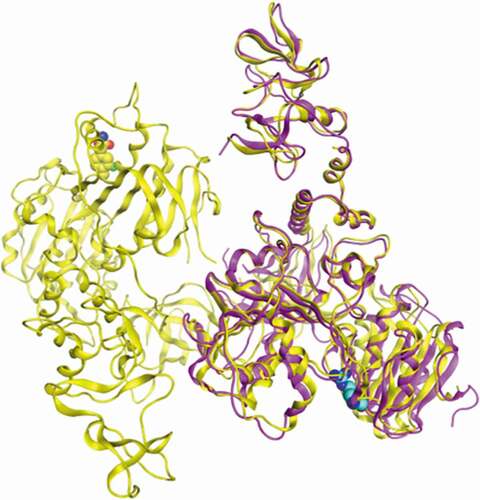
Figure 2. Zafirlukast docking to SARS-CoV-2 helicase: (a) A dock pose of zafirlukast (ball-and–stick model) to SARS-CoV-2 helicase nucleotide binding site (surface view) using MOE. Protein was allowed 9 Å flexibility away from ligand. Zafirlukast is presented (b) Interaction map of zafirlukast with SARS-CoV-2 helicase. The basic, acidic and the polar amino acids are represented by the pink spheres with blue, red and black outlines respectively. Hydrophobic residues are presented in green. Blue and green arrows indicate hydrogen bonding to backbone and side chain atoms respectively. The fuzzy blue spheres represent the ligand atoms exposed to solvent as determined by the docking process. Light-blue shadows around residues indicate the degree of interaction with ligand atoms. The dotted contour reflects steric. The steric room for methyl substitution is presented by dotted contours
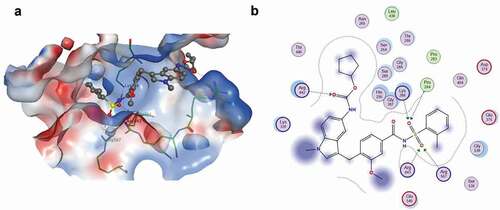
SARS-CoV-2 helicase purification and activity
After expression in BL21 (DE3) cells and purification by a Strep-Tactin column, 1 L media yielded about 1 mg of SARS-CoV-2 helicase. The purity of the enzyme was checked by SDS-PAGE – see . To validate the purified enzyme activity, both the helicase and ATPase activities were measured. The ATPase activity was determined by measuring the total amount of released inorganic phosphate at 650 nm – see ) and the helicase activity by measuring the emission at 520 nm after excitation at 495 nm – see . The assay conditions and the standard curve of the single-stranded DNA labelled with fluorescein were established to convert RFUs to change in double-strand concentration – see Figure S2. The kinetic parameters of the SARS-CoV-2 helicase unwinding activity were determined by using the FRET-based assay at different concentrations for dsDNA and a constant concentration of ATP as a second substrate and at different concentrations of ATP and a fixed concentration of dsDNA as a second substrate. The results were fitted by the Michaelis–Menten equation to obtain the Michaelis–Menten constant and maximum velocities – see and Figure S3.
Table 2. Kinetic parameters of SARS-CoV-2 helicase
Figure 3. SARS-CoV-2 helicase expression and activity: (a) Helicase purification analysed by SDS-PAGE and detected by Coomassie staining. Lane 1, prestained molecular standards (BioRad); lane 2, eluted SARS-CoV-2 helicase from Strep-Tactin column. (b) SARS-CoV-2 helicase ATPase activity incubated measured by monitoring the change in absorbance of the released inorganic phosphate at 650 nm after the addition of detection dye after incubation of 250 nM dsDNA and 0.25 mM ATP for 60 min at no enzyme or with 20 nM SARS-CoV-2 helicase. (c) SARS-CoV-2 helicase activity measured by monitoring the change in fluorescence (excitation 495 nm, emission 520 nm) for 10 min incubation of 75 nM fluorescein- and black hole quencher-labelled dsDNA and 0.5 mM ATP at no enzyme or 50 nM SARS-CoV-2 helicase
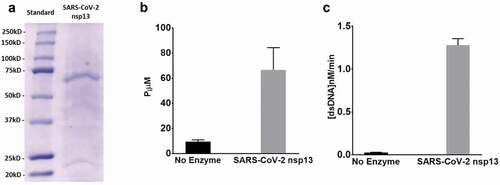
SARS-CoV-2 helicase inhibition
The inhibitory effect of the nine compounds selected through virtual screening on the SARS-CoV-2 helicase activity was directly measured with a FRET-based assay in 96-well plates. Of the nine compounds, zafirlukast was the only compound inhibiting the SARS-CoV-2 helicase at 100 µM final concentration – see . The dsDNA was used to mimic the natural substrate. The assays were performed in 100 µM final concentration conditions. The IC50 of zafirlukast was determined – see . The same assay conditions were used to measure the inhibitory effect of the previously reported MERS-CoV helicase inhibitors. Doxorubicin HCl, epirubicin HCl, mitoxantrone 2HCl, daunorubicin HCl and idarubicin HCl completely abolished the SARS-CoV-2 helicase activity at IC50’s lower than 3 µM final concentrations. Ethacridine lactate monohydrate, sunitinib malate, otilonium bromide, masitinib, and diminazene aceturate inhibited the SARS-CoV-2 helicase IC50’s between 20 and 500 µM. Ruxolitinib, tolcapone, raloxifene HCl, and bazedoxifene HCl did not inhibit the SARS-CoV-2 helicase activity even at 100 µM final concentrations – see and Figure S4. Montelukast did not inhibit SARS-CoV-2 helicase activity at 25 µM final concentration – see .
Table 3. IC50 values of SARS-CoV-2 inhibitors
Figure 4. SARS-CoV-2 helicase inhibition by compounds identified by virtual screening: (a) SARS-CoV-2 helicase activity measured in the presence of 75 nM fluorescein- and black hole quencher-labelled dsDNA, 0.5 mM ATP and 100 mM of zafirlukast, bosentan hydrate, rigosertib, chondroitin sulphate, encorafenib, darunavir, mezlocillin sodium, tianeptine sodium, lapatinib, or DMSO. (b) IC50 value of zafirlukast was measured using the FRET-based assay. The SARS-CoV-2 helicase was incubated with 75 nM fluorescein- and black hole quencher-labelled dsDNA, 0.5 mM ATP and various concentrations: 0 µM, 1 × 10–3 µM, 1.7 × 10–3 µM, 5.1 × 10–3 µM, 15.2 × 10–3 µM, 45.7 × 10–3 µM, 0.1372 µM, 0.4115 µM, 1.234 µM, 3.704 µM, 11.11 µM, 33.33 µM, and 100 µM of zafirlukast. (c) The SARS-CoV-2 helicase activity measured in the presence of 75 nM fluorescein- and black hole quencher-labelled dsDNA, 0.5 mM ATP and 25 µM of zafirlukast or montelukast
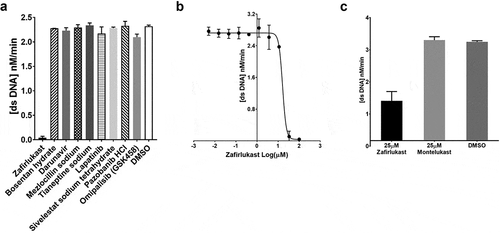
Vero E6 cells viability and infection by SARS-CoV-2
Vero E6 cell viability was monitored after the addition of different compounds to their growth and maintenance media. Uninfected Vero E6 cells grow similarly in the presence of 0.1% DMSO, 25 µM zafirlukast or 25 µM montelukast – see . Titre Gloassay of uninfected Vero E6 cells indicated that in comparison to 1% DMSO treatment (control), both zafirlukast and montelukast killed Vero E6 cells at 50 and 100 µM, however, montelukast was significantly more potent than zafirlukast. There was no significant difference between the control group and both compound treatments at 25 µM or less concentrations – see . Before the SARS-CoV-2 infection, the Vero E6 cells grew as a monolayer in the presence of 0.1% DMSO. Monolayers of Vero E6 cells infected with SARS-CoV-2 previously treated with either 0.1% DMSO or 25 µM montelukast were largely disturbed. However, the monolayers of the Vero E6 cells infected with SARS-CoV-2 previously treated with 25 µM zafirlukast retained 60–70% of their integrity – see . The qPCR of the Vero E6 cells infected with SARS-CoV-2 and treated with 0.1% DMSO or 25 µM montelukast resulted in a CT value of 13, relatively lower than the qPCR of cells infected with SARS-CoV-2 and treated with 25 µM zafirlukast, which was 17.
Figure 5. The effect of zafirlukast and montelukast on Vero E6 cells infection by SARS-CoV-2:(a) Integrity of Vero E6 cells inoculated in the presence of 1% DMSO, 25 µM zafirlukast or 25µ montelukast. (b) Vero E6 cells viability measured by TitreGlo assay after overnight incubation at 37°C and 5% CO2 in the presence of 1%DMSO (white), zafirlukast (black), or montelukast (grey). (c) The integrity of Vero E6 cells treated with 1% DMSO and not infected (control) or treated with 1% DMSO, 25 µM montelukast, or 25 µM zafirlukast and then infected with SARS-CoV-2
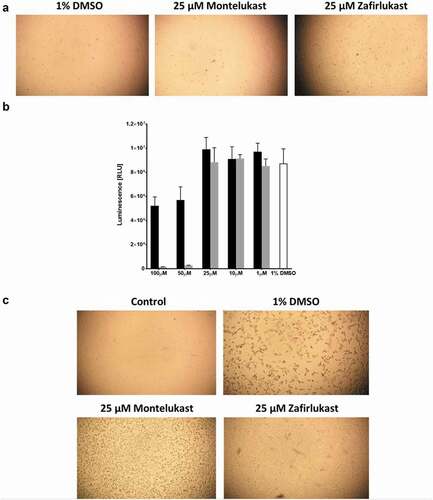
Discussion
The central role of the SARS-CoV-2 helicase in the formation of the replication/transcription centres (RTCs) makes it a potential antiviral target [Citation45]. Previous studies indicated that a sulphate moiety located at the nucleotide binding site of the MERS-CoV helicase is essential for enzyme activity, since it is stabilized by several key residues in the binding site [Citation49]. Compounds with sulphoxide moieties could possibly bind to and inhibit the SARS-CoV-2 helicase through these moieties. To investigate this possible role of sulphoxide moieties, it was first necessary to locate the nucleotide-binding domain within the fragmented atomic structure of the SARS-CoV-2 helicase. Since the SARS-CoV-2 and MERS-CoV helicases share a reasonable identity (72.4%) and a highly conserved sequence of the nucleotide-binding site, except the tyrosine residue 442, which is replaced by an arginine in the SARS-CoV-2 helicase – see Figure S5, it is reasonable to assume that the binding sites of the two enzymes will have a similar three-dimensional structure. Superimposition of the fragmented atomic structure of the SARS-CoV-2 helicase with the full MERS-CoV helicase atomic structure enabled the identification of the nucleotide-binding pocket within the SARS-CoV-2. An induced docking protocol was used to dock the compounds with a sulphoxide or sulphone moiety. Since the interactions were based on the sulphate ion, which interacts by long-range electrostatic interactions, all the binding site residues within a 9 Å distance radius from the ligand atoms were allowed to move/flex during the docking of the ligand. The binding site atoms outside the 9 Å were held rigid. The binding pocket of the enzyme is comprised of flexible loops – see . To incorporate the dynamics of the loop movements as much as possible, the induced docking protocol was expanded to a radius of 9 Å. Zafirlukast has the best docking score of the nine compounds containing a sulphoxide or sulphone moiety and was the only compound to inhibit the SARS-CoV-2 helicase unwinding activity.
The IC50 of zafirlukast in the current study is in agreement with the recently published inhibitory effect of zafirlukast on the SARS-CoV-2 helicase in the absence of non-ionic detergent (IC50 = 3.6 µM) [Citation57]. The same study showed that the inhibitory effect of zafirlukast diminished in the presence of 0.02% tween 20. The authors concluded that zafirlukast’s inhibitory effect in the absence of the detergent is an apparently a false-positive due to colloid formation. However, the effect of the detergent on the enzyme itself was not investigated in this study. Previous studies showed that some non-ionic detergents including tween 20 could significantly affect enzyme activities like West Nile Virus protease, and they are potentially unsuitable to discriminate true versus false inhibitors in high throughput assays [Citation58]. In addition, no evidence of zafirlukast-induced colloid formation was presented in that study [Citation57]. Colloid formation under different assay conditions can be experimentally measured by dynamic light scattering, centrifugation, or several other methods [Citation59]. For these reasons, zafirlukast cannot be dismissed as a possible inhibitor for the SARS-CoV-2 helicase, especially when there is evidence of tight binding to the nucleotide-binding site shown by docking experiment and confirmed by the inhibitory effect of zafirlukast on infected Vero E6 cells. Several in silico studies have emphasized the potential use of zafilrukast as an antiviral agent. Molecular docking studies predicted the potential inhibitory effect of zafirlukast on SARS-CoV-2 RdRp and 3CLpro [Citation60]. An artificial intelligence (AI) screening technique using a supervised machine learning model previously trained on the in vitro data of the effect of FDA-approved drugs on SARS-CoV-2 3CLpro activity selected zafilukast as the best candidate inhibitor [Citation61]. Moreover, the interactive online platform (https://shennongproject.ai/) used to report the data of systemic in silico screening of the FDA-approved drugs against SARS-CoV-2 proteins, indicated that zafirlukast could potentially bind to nsp13; however, no target-binding site was identified within the nsp13 structure [Citation62]. The current study identifies the nucleotide-binding site as the target-binding site, locating specific interactions between the essential binding residues and zafirlukast. Compared to the other eight sulphate moiety-containing compounds, zafirlukast was the only molecule to interact with all four residues. Three residues: Q404, R443 and R567 interact directly with the sulphate moiety of zafirlukast. Additional interaction is observed between residue P284 and zafirlukast sulphate moiety. Residue R442 interacts with a carbonyl amide moiety of zafirlukast. Similar to zafirlukast, lapatinib binds in the same vicinity and has several interactions between its sulphate moiety and three of the essential residues in the binding site; however, it did not inhibit the enzyme. This difference in the inhibitory effect could be explained by the less tight binding of lapatinib to the active site compared to zafirlukast, as indicated by the higher docking scores of lapatinib. All the other compounds did interact with the essential residues in the binding sites, but through different moieties than the sulphate moiety. The docking scores of these compounds were less than the zafirlukast docking scores. This emphasizes the role of the sulphate moiety in the compound binding to the active site.
Pranlukast and montelukast are leukotriene receptor antagonists used as alternatives of zafirlukast to treat asthma [Citation63,Citation64]. Several reviews emphasized on the possible role of montelukast to alleviate some of pulmonary manifestations triggered by SARS-CoV-2 infection [Citation65–67]. A recent report found that treating COVID-19 confirmed patients with montelukast, a leukotriene receptor antagonist similar to zafirlukast, was associated with less clinical deterioration signs [Citation68]. Montelukast docking to SARS-CoV-2 helicase nucleotide-binding site and its effect on SARS-CoV-2 helicase unwinding activity were investigated. Despite its lack of a sulphoxide or sulphone moiety, montelukast docked to the active site with a docking score comparable to zafirlukast, however, it did not inhibit the helicase unwinding activity. This suggests that docking with an appropriate orientation and establishing essential interactions are equally required to achieve enzyme inhibition. The residues, other than the designated four residues, could also be essential for the inhibition mechanism. P284 interaction with the sulphate moiety is observed in zafirlukast. P284 is a conserved residue located at domain I of the helicase nucleotide-binding site and seems to be central for the zafirlukast binding and inhibition mechanism. None of the sulphoxide or sulphone containing molecules or montelukast establishes this interaction. The effects of pranlukast on SARS-CoV-2 helicase activity were not tested since it has been recently shown that pranlukast treatment failed to reduce Vero E6 cells infection with SARS-CoV-2 [Citation69].
To measure the possible inhibitory effect of the compounds detected by virtual docking on the SARS-CoV-2 helicase activity, the enzyme was expressed in the BL21 (DE3) bacterial system. SARS-CoV-2 has a similar size and activities to what has been reported for MERS-CoV and SARS-CoV [Citation41,Citation54]. Recombinant SARS-CoV-2 helicase catalyses two activities: helicase activity and ATPase activity. The unwinding helicase activity was measured by a FRET-based assay, the dsDNA substrate has a length of 24 bp and contained a fork confirmation to ensure better enzyme binding and allow the complete separation under assay conditions [Citation29]. The enzyme assay conditions did not interfere with the FRET-based measurements. The SARS-CoV-2 activity properties were in good agreement with what is reported for MERS-CoV and SARS-CoV. The Km values for the dsDNA and ATP agree with the Km values of MERS-CoV [Citation54]. In addition, SARS-CoV-2 was inhibited in a similar pattern and potency by previously reported MERS-CoV helicase inhibitors [Citation54]. Similar to the MERS-CoV helicase, strong inhibitors completely inhibited the SARS-CoV-2 helicase unwinding activity at concentrations less than 1 µM, and the weak inhibitors affected the unwinding activity of SARS-CoV-2 helicase at concentrations higher than 10 µM. The values of the IC50’s of idarubicin HCl, doxorubicin HCl, epirubicin HCl, mitoxantrone 2HCl, and daunorubicin HCl for SARS-CoV-2 are in good agreement with those reported for the MERS-CoV helicase. This indicates that these compounds potentially inhibit the SARS-CoV-2 helicase with a similar mechanism that inhibited the MERS-CoV helicase. The mechanism of inhibition of the strong inhibitors could be by competing with the dsDNA or the ATP on their binding sites, since these compounds previously docked well in the nucleotide-binding site of the MERS-CoV helicase. Intercalating dsDNA is also possible since they did previously bind to DNA with binding constants close to their reported IC50’s [Citation54]. The SARS-CoV-2 helicase response to the weak inhibitors was relatively different from the MERS-CoV helicase response. Unlike the MERS-CoV helicase, ruxolitinib, tolcapone, raloxifene HCl, and bazedoxifene HCl did not inhibit the SARS-CoV-2 helicase unwinding activity. Sunitinib malate, otilonium bromide, masitinib, and diminazene aceturate inhibited both helicases with some variation as indicated by the IC50’s values. A previous docking study showed that many of these compounds do not dock well in the helicase nucleotide binding site, and they are more likely to excrete their inhibitory effect by binding to less conserved sites in the helicase. Variations in the non-conserved binding site sequences could explain the variations of the inhibitory effects of these inhibitors on SARS-CoV-2 and MERS-CoV helicases. Zafirlukast has no known DNA binding properties; therefore, intercalating dsDNA is a less likely mechanism of action.
Vero E6 cells pretreated with zafirlukast and infected with the SARS-CoV-2 virus retained most of their integrity. This suggests that zafirlukast is effective in alleviating the pathogenic effects of SARS-CoV-2 infection. Zafirlukast anti-pathogenic effects were observed at micromolar concentrations, similar to the IC50’s of zafirlukast in vitro inhibition of the SARS-CoV-2 helicase. Zafirlukast apparently impaired the viral replication/transcription pathway as indicated by the low qPCR CT values. Zafirlukast showed no toxicity effects on the uninfected Vero E6 cells at these concentrations. Montelukast did not alleviate the SARS-CoV-2 pathogenic effects on Vero E6 cells or affected the replication/transcription pathway. This agrees with the inability of montelukast to inhibit SARS-CoV-2 helicase. Montelukast could be a good leukotriene receptor antagonist alternative in asthma treatment; however, it is not a good alternative inhibiting the helicase of SARS-CoV-2. This study is the first study to use Vero 6 cell integrity in combination with in silico modelling and a FRET-based assay screening to investigate the zafirlukast inhibitory effects on the SARS-CoV-2 helicase. The tested concentrations of zafirlukast in FRET-based assay and Vero E6 assay are in close agreement with the pharmacokinetics and pharmacodynamic properties of zafirlukast which indicate that zafirlukast reaches maximal plasma concentrations (>1 µM) after 3 h of oral consumption depending on the initial oral dosage [Citation70]. The current study underlines the potential use of zafirlukast as an antiviral agent to SARS-CoV-2 and other coronavirus infections, however, further clinical studies are required to determine its efficacy. Lately, a Phase 2 clinical trial of zafrilukast treatment of COVID-19 patients has been approved by the Saudi Food and Drug Authority (SFDA).
Availability of data and materials
All the data used to support the findings of this study are included in the article, and supplementary information is provided in the supplementary section. In addition, the data used to support the findings of this study are available from the corresponding author on request.
Supplemental Material
Download MS Word (2.2 MB)Acknowledgements
We thank KAIMRC for funding this research. We thank all KAIMRC researchers and administrative staff for assisting directly and indirectly in this research.
Disclosure statement
No potential conflict of interest was reported by the author(s).
Supplementary material
Supplemental data for this article can be accessed at: https://doi.org/10.1080/1062936X.2021.1993995
Additional information
Funding
References
- V.C. Cheng, S.K. Lau, P.C. Woo, and K.Y. Yuen, Severe acute respiratory syndrome coronavirus as an agent of emerging and reemerging infection, Clin. Microbiol. Rev. 20 (2007), pp. 660–694.
- N. Lee, D. Hui, A. Wu, P. Chan, P. Cameron, G.M. Joynt, A. Ahuja, M.Y. Yung, C.B. Leung, K.F. To, S.F. Lui, C.C. Szeto, S. Chung, and J.J. Sung, A major outbreak of severe acute respiratory syndrome in Hong Kong, N. Engl. J. Med. 348 (2003), pp. 1986–1994. doi:https://doi.org/10.1056/NEJMoa030685.
- A.M. Zaki, S. Van Boheemen, T.M. Bestebroer, A.D. Osterhaus, and R.A. Fouchier, Isolation of a novel coronavirus from a man with pneumonia in Saudi Arabia, N. Engl. J. Med. 367 (2012), pp. 1814–1820. doi:https://doi.org/10.1056/NEJMoa1211721.
- R.J. de Groot, S.C. Baker, R.S. Baric, C.S. Brown, C. Drosten, L. Enjuanes, R.A. Fouchier, M. Galiano, A.E. Gorbalenya, Z.A. Memish, S. Perlman, L.L. Poon, E.J. Snijder, G.M. Stephens, P.C. Woo, A.M. Zaki, M. Zambon, and J. Ziebuhr, Middle East respiratory syndrome coronavirus (MERS-CoV): Announcement of the Coronavirus Study Group, J. Virol. 87 (2013), pp. 7790–7792. doi:https://doi.org/10.1128/JVI.01244-13.
- P. Zhou, X.L. Yang, X.G. Wang, B. Hu, L. Zhang, W. Zhang, H.R. Si, Y. Zhu, B. Li, C.L. Huang, H.D. Chen, J. Chen, Y. Luo, H. Guo, R.D. Jiang, M.Q. Liu, Y. Chen, X.R. Shen, X. Wang, X.S. Zheng, K. Zhao, Q.J. Chen, F. Deng, L.L. Liu, B. Yan, F.X. Zhan, Y.Y. Wang, G.F. Xiao, and Z.L. Shi, A pneumonia outbreak associated with a new coronavirus of probable bat origin, Nature 579 (2020), pp. 270–273. doi:https://doi.org/10.1038/s41586-020-2012-7.
- C. Huang, Y. Wang, X. Li, L. Ren, J. Zhao, Y. Hu, L. Zhang, G. Fan, J. Xu, X. Gu, Z. Cheng, T. Yu, J. Xia, Y. Wei, W. Wu, X. Xie, W. Yin, H. Li, M. Liu, Y. Xiao, H. Gao, L. Guo, J. Xie, G. Wang, R. Jiang, Z. Gao, Q. Jin, J. Wang, and B. Cao, Clinical features of patients infected with 2019 novel coronavirus in Wuhan, China, Lancet 395 (2020), pp. 497–506. doi:https://doi.org/10.1016/S0140-6736(20)30183-5.
- Y.A. Helmy, M. Fawzy, A. Elaswad, A. Sobieh, S.P. Kenney, and A.A. Shehata, The COVID-19 pandemic: A comprehensive review of taxonomy, genetics, epidemiology, diagnosis, treatment, and control, J. Clin. Med. 9 (2020), pp. 1225. doi:https://doi.org/10.3390/jcm9041225.
- I. Astuti, Severe acute respiratory syndrome coronavirus 2 (SARS-CoV-2): An overview of viral structure and host response, Diabetes Metab. Syndr. 14 (2020), pp. 407–412. doi:https://doi.org/10.1016/j.dsx.2020.04.020.
- P. V’kovski, A. Kratzel, S. Steiner, H. Stalder, and V. Thiel, Coronavirus biology and replication: Implications for SARS-CoV-2, Nat. Rev. Microbiol. 19 (2021), pp. 155–170. doi:https://doi.org/10.1038/s41579-020-00468-6.
- J.D. Twomey, S. Luo, A.Q. Dean, W.P. Bozza, A. Nalli, and B. Zhang, COVID-19 update: The race to therapeutic development, Drug Resist. Updates 53 (2020), pp. 100733. doi:https://doi.org/10.1016/j.drup.2020.100733.
- A. Saxena, Drug targets for COVID-19 therapeutics: Ongoing global efforts, J. Biosci. 45 (2020), pp. 87.
- A. Zumla, J.F. Chan, E.I. Azhar, D.S. Hui, and K.Y. Yuen, Coronaviruses - drug discovery and therapeutic options, Nat. Rev. Drug Discov. 15 (2016), pp. 327–347.
- S.J. Macalino, V. Gosu, S. Hong, and S. Choi, Role of computer-aided drug design in modern drug discovery, Arch. Pharm. Res. 38 (2015), pp. 1686–1701. doi:https://doi.org/10.1007/s12272-015-0640-5.
- G. Li and E. De Clercq, Therapeutic options for the 2019 novel coronavirus (2019-nCoV), Nat. Rev. Drug Discov. 19 (2020), pp. 149–150. doi:https://doi.org/10.1038/d41573-020-00016-0.
- L. Hage-Melim, L.B. Federico, N. de Oliveira, V. Francisco, L.C. Correia, H.B. De Lima, S.Q. Gomes, M.P. Barcelos, I. Francischini, and C. Da Silva, Virtual screening, ADME/Tox predictions and the drug repurposing concept for future use of old drugs against the COVID-19, Life Sci. 256 (2020), pp. 117963. doi:https://doi.org/10.1016/j.lfs.2020.117963.
- S. Pushpakom, F. Iorio, P.A. Eyers, K.J. Escott, S. Hopper, A. Wells, A. Doig, T. Guilliams, J. Latimer, C. McNamee, A. Norris, P. Sanseau, D. Cavalla, and M. Pirmohamed, Drug repurposing: Progress, challenges and recommendations, Nat. Rev. Drug. Discov. 18 (2019), pp. 41–58. doi:https://doi.org/10.1038/nrd.2018.168.
- R.T. Eastman, J.S. Roth, K.R. Brimacombe, A. Simeonov, M. Shen, S. Patnaik, and M.D. Hall, Remdesivir: A review of its discovery and development leading to emergency use authorization for treatment of COVID-19, ACS Cent. Sci. 6 (2020), pp. 672–683. doi:https://doi.org/10.1021/acscentsci.0c00489.
- T. Polgár and G.M. Keseru, Integration of virtual and high throughput screening in lead discovery settings, Comb. Chem. High Throughput Screen. 14 (2011), pp. 889–897. doi:https://doi.org/10.2174/138620711797537148.
- M. Rumlová and T. Ruml, In vitro methods for testing antiviral drugs, Biotechnol. Adv. 36 (2018), pp. 557–576.
- K.G. Andersen, A. Rambaut, W.I. Lipkin, E.C. Holmes, and R.F. Garry, The proximal origin of SARS-CoV-2, Nat. Med. 26 (2020), pp. 450–452. doi:https://doi.org/10.1038/s41591-020-0820-9.
- N. Kaur, R. Singh, Z. Dar, R.K. Bijarnia, N. Dhingra, and T. Kaur, Genetic comparison among various coronavirus strains for the identification of potential vaccine targets of SARS-CoV2, Infect. Genet. Evol. 89 (2021), pp. 104490. doi:https://doi.org/10.1016/j.meegid.2020.104490.
- J.S. Morse, T. Lalonde, S. Xu, and W.R. Liu, Learning from the past: Possible urgent prevention and treatment options for severe acute respiratory infections caused by 2019-nCoV, Chembiochem 21 (2020), pp. 730–738. doi:https://doi.org/10.1002/cbic.202000047.
- D.C. Hall Jr and H.F. Ji, A search for medications to treat COVID-19 via in silico molecular docking models of the SARS-CoV-2 spike glycoprotein and 3CL protease, Travel. Med. Infect. Dis. 35 (2020), pp. 101646. doi:https://doi.org/10.1016/j.tmaid.2020.101646.
- R. Arya, S. Kumari, B. Pandey, H. Mistry, S.C. Bihani, A. Das, V. Prashar, G.D. Gupta, L. Panicker, and M. Kumar, Structural insights into SARS-CoV-2 proteins, J. Mol. Biol. 433 (2021), pp. 166725. doi:https://doi.org/10.1016/j.jmb.2020.11.024.
- R. Gowthaman, J.D. Guest, R. Yin, J. Adolf-Bryfogle, W.R. Schief, and B.G. Pierce, CoV3D: A database of high resolution coronavirus protein structures, Nucleic Acids Res. 49 (2021), pp. D282–D287. doi:https://doi.org/10.1093/nar/gkaa731.
- M.I. Ionescu, An overview of the crystallized structures of the SARS-CoV-2, Protein J. 39 (2020), pp. 600–618. doi:https://doi.org/10.1007/s10930-020-09933-w.
- T.M. Lohman and K.P. Bjornson, Mechanisms of helicase-catalyzed DNA unwinding, Annu. Rev. Biochem. 65 (1996), pp. 169–214. doi:https://doi.org/10.1146/annurev.bi.65.070196.001125.
- G.W. Owttrim, RNA helicases: Diverse roles in prokaryotic response to abiotic stress, RNA Biol. 10 (2013), pp. 96–110. doi:https://doi.org/10.4161/rna.22638.
- K. Geider and H. Hoffmann-Berling, Proteins controlling the helical structure of DNA, Annu. Rev. Biochem. 50 (1981), pp. 233–260. doi:https://doi.org/10.1146/annurev.bi.50.070181.001313.
- Z. Xing, W.K. Ma, and E.J. Tran, The DDX5/Dbp2 subfamily of DEAD-box RNA helicases, Wiley Interdiscip Rev RNA 10 (2019), pp. e1519. doi:https://doi.org/10.1002/wrna.1519.
- R.M. Brosh Jr. and S.W. Matson, History of DNA helicases, Genes (Basel) 11 (2020), pp. 255. doi:https://doi.org/10.3390/genes11030255.
- S. Aarattuthodiyil, A.K. Byrd, and K.D. Raney, Simultaneous binding to the tracking strand, displaced strand and the duplex of a DNA fork enhances unwinding by Dda helicase, Nucleic Acids Res. 42 (2014), pp. 11707–11720. doi:https://doi.org/10.1093/nar/gku845.
- J. Xia, L.T. Chen, Q. Mei, C.H. Ma, J.A. Halliday, H.Y. Lin, D. Magnan, J.P. Pribis, D.M. Fitzgerald, H.M. Hamilton, M. Richters, R.B. Nehring, X. Shen, L. Li, D. Bates, P.J. Hastings, C. Herman, M. Jayaram, and S.M. Rosenberg, Holliday junction trap shows how cells use recombination and a junction-guardian role of RecQ helicase, Sci. Adv. 2 (2016), pp. e1601605. doi:https://doi.org/10.1126/sciadv.1601605.
- B. Bartholomew, ISWI chromatin remodeling: One primary actor or a coordinated effort?, Curr. Opin. Struct. Biol. 24 (2014), pp. 150–155. doi:https://doi.org/10.1016/j.sbi.2014.01.010.
- L. Subissi, I. Imbert, F. Ferron, A. Collet, B. Coutard, E. Decroly, and B. Canard, SARS-CoV ORF1b-encoded nonstructural proteins 12-16: Replicative enzymes as antiviral targets, Antiviral Res. 101 (2014), pp. 122–130. doi:https://doi.org/10.1016/j.antiviral.2013.11.006.
- E. Prentice, J. McAuliffe, X. Lu, K. Subbarao, and M.R. Denison, Identification and characterization of severe acute respiratory syndrome coronavirus replicase proteins, J. Virol. 78 (2004), pp. 9977–9986. doi:https://doi.org/10.1128/JVI.78.18.9977-9986.2004.
- S. Habtemariam, S.F. Nabavi, M. Banach, I. Berindan-Neagoe, K. Sarkar, P.C. Sil, and S.M. Nabavi, Should we try SARS-CoV-2 helicase inhibitors for COVID-19 therapy?, Arch. Med. Res. 51 (2020), pp. 733–735. doi:https://doi.org/10.1016/j.arcmed.2020.05.024.
- X. Hu, W. Hao., B. Qin, Z. Tian, Z. Li, P. Hou, R. Zhao, S. Cui, and J. Diao, Mechanism of duplex unwinding by coronavirus nsp13 helicases, bioRxiv (2020). 08.02.233510.
- A.O. Adedeji, B. Marchand, A.J. Te Velthuis, E.J. Snijder, S. Weiss, R.L. Eoff, K. Singh, and S.G. Sarafianos, Mechanism of nucleic acid unwinding by SARS-CoV helicase, PloS One 7 (2012), pp. e36521. doi:https://doi.org/10.1371/journal.pone.0036521.
- A.B. Gurung, In silico structure modelling of SARS-CoV-2 Nsp13 helicase and Nsp14 and repurposing of FDA approved antiviral drugs as dual inhibitors, Gene Rep. 21 (2020), pp. 100860. doi:https://doi.org/10.1016/j.genrep.2020.100860.
- W. Hao, J.A. Wojdyla, R. Zhao, R. Han, R. Das, I. Zlatev, M. Manoharan, M. Wang, and S. Cui, Crystal structure of Middle East respiratory syndrome coronavirus helicase, PLoS Pathog. 13 (2017), pp. e1006474. doi:https://doi.org/10.1371/journal.ppat.1006474.
- Z. Jia, L. Yan, Z. Ren, L. Wu, J. Wang, J. Guo, L. Zheng, Z. Ming, L. Zhang, Z. Lou, and Z. Rao, Delicate structural coordination of the Severe Acute Respiratory Syndrome coronavirus Nsp13 upon ATP hydrolysis, Nucleic Acids Res. 47 (2019), pp. 6538–6550. doi:https://doi.org/10.1093/nar/gkz409.
- J.A. Newman, Y. Yosaatmadja, A. Douangamath, C.H. Arrowsmith, F. von Delft, A. Edwards, C. Bountra, and O. Gileadi, Crystal structure of the SARS-CoV-2 helicase at 1.94 Angstrom resolution, Protein Data Bank (2020). doi:https://doi.org/10.2210/pdb6ZSL/pdb.
- O.M. Ugurel, O. Mutlu, E. Sariyer, S. Kocer, E. Ugurel, T.G. Inci, O. Ata, and D. Turgut-Balik, Evaluation of the potency of FDA-approved drugs on wild type and mutant SARS-CoV-2 helicase (Nsp13), Int. J. Biol. Macromol. 163 (2020), pp. 1687–1696. doi:https://doi.org/10.1016/j.ijbiomac.2020.09.138.
- M.A. White, W. Lin, and X. Cheng, Discovery of COVID-19 inhibitors targeting the SARS-CoV-2 Nsp13 helicase, J. Phys. Chem. Lett. 11 (2020), pp. 9144–9151. doi:https://doi.org/10.1021/acs.jpclett.0c02421.
- M.U. Mirza and M. Froeyen, Structural elucidation of SARS-CoV-2 vital proteins: Computational methods reveal potential drug candidates against main protease, Nsp12 polymerase and Nsp13 helicase, J. Pharm. Anal. 10 (2020), pp. 320–328. doi:https://doi.org/10.1016/j.jpha.2020.04.008.
- C. Gorgulla, K.M. Padmanabha Das, K.E. Leigh, M. Cespugli, P.D. Fischer, Z.F. Wang, G. Tesseyre, S. Pandita, A. Shnapir, A. Calderaio, M. Gechev, A. Rose, N. Lewis, C. Hutcheson, E. Yaffe, R. Luxenburg, H.D. Herce, V. Durmaz, T.D. Halazonetis, K. Fackeldey, J.J. Patten, A. Chuprina, I. Dziuba, A. Plekhova, Y. Moroz, D. Radchenko, O. Tarkhanova, I. Yavnyuk, C. Gruber, R. Yust, D. Payne, A.M. Näär, M.N. Namchuk, R.A. Davey, G. Wagner, J. Kinney, and H. Arthanari, A multi-pronged approach targeting SARS-CoV-2 proteins using ultra-large virtual screening, iScience 24 (2021), pp. 102021. doi:https://doi.org/10.1016/j.isci.2020.102021.
- S. Khater and G. Das, Repurposing Ivermectin to inhibit the activity of SARS CoV2 helicase: Possible implications for COVID 19 therapeutics, OSF Preprints (2020), pp. 1–17.
- M.R. Freidel and R.S. Armen, Mapping major SARS-CoV-2 drug targets and assessment of druggability using computational fragment screening: Identification of an allosteric small-molecule binding site on the Nsp13 helicase, PloS One 16 (2021), pp. e0246181. doi:https://doi.org/10.1371/journal.pone.0246181.
- M.S. Yu, J. Lee, J.M. Lee, Y. Kim, Y.W. Chin, J.G. Jee, Y.S. Keum, and Y.J. Jeong, Identification of myricetin and scutellarein as novel chemical inhibitors of the SARS coronavirus helicase, nsP13, Bioorg. Med. Chem. Lett. 22 (2012), pp. 4049–4054. doi:https://doi.org/10.1016/j.bmcl.2012.04.081.
- A.O. Adedeji, K. Singh, N.E. Calcaterra, M.L. DeDiego, L. Enjuanes, S. Weiss, and S.G. Sarafianos, Severe acute respiratory syndrome coronavirus replication inhibitor that interferes with the nucleic acid unwinding of the viral helicase, Antimicrob. Agents Chemother. 56 (2012), pp. 4718–4728. doi:https://doi.org/10.1128/AAC.00957-12.
- A.O. Adedeji, K. Singh, A. Kassim, C.M. Coleman, R. Elliott, S.R. Weiss, M.B. Frieman, and S.G. Sarafianos, Evaluation of SSYA10-001 as a replication inhibitor of severe acute respiratory syndrome, mouse hepatitis, and Middle East respiratory syndrome coronaviruses, Antimicrob. Agents Chemother. 58 (2014), pp. 4894–4898. doi:https://doi.org/10.1128/AAC.02994-14.
- N.H. Zaher, M.I. Mostafa, and A.Y. Altaher, Design, synthesis and molecular docking of novel triazole derivatives as potential CoV helicase inhibitors, Acta Pharma. 70 (2020), pp. 145–159. doi:https://doi.org/10.2478/acph-2020-0024.
- N. Mehyar, A. Mashhour, I. Islam, S. Gul, A.O. Adedeji, A.S. Askar, and M. Boudjelal, Using in silico modelling and FRET-based assays in the discovery of novel FDA-approved drugs as inhibitors of MERS-CoV helicase, SAR QSAR Environ. Res. 32 (2020), pp. 51–70. doi:https://doi.org/10.1080/1062936X.2020.1857437.
- H.A. Alhadrami, A.M. Hassan, R. Chinnappan, H. Al-Hadrami, W.H. Abdulaal, E.I. Azhar, and M. Zourob, Peptide substrate screening for the diagnosis of SARS-CoV-2 using fluorescence resonance energy transfer (FRET) assay, Mikrochim. Acta 188 (2021), pp. 137. doi:https://doi.org/10.1007/s00604-021-04766-5.
- LiverTox: Clinical and Research Information on Drug-Induced Liver Injury [Internet], National Institute of Diabetes and Digestive and Kidney Diseases, Bethesda (MD), (2012)-. Zafirlukast. [Updated 2019 June 4]. Available at: https://www.ncbi.nlm.nih.gov/books/NBK547915/
- J. Zeng, F. Weissmann, A.P. Bertolin, V. Posse, B. Canal, R. Ulferts, M. Wu, R. Harvey, S. Hussain, J.C. Milligan, C. Roustan, A. Borg, L. McCoy, L.S. Drury, S. Kjaer, J. McCauley, M. Howell, R. Beale, and J.F.X. Diffley, Identifying SARS-CoV-2 antiviral compounds by screening for small molecule inhibitors of nsp13 helicase, Biochem. J. 478 (2021), pp. 2405–2423. doi:https://doi.org/10.1042/BCJ20210201.
- M.D. Ezgimen, N.H. Mueller, T. Teramoto, and R. Padmanabhan, Effects of detergents on the West Nile virus protease activity, Bioorg. Med. Chem. 17 (2009), pp. 3278–3282. doi:https://doi.org/10.1016/j.bmc.2009.03.050.
- D. Reker, G.J.L. Bernardes, and T. Rodrigues, Computational advances in combating colloidal aggregation in drug discovery, Nat. Chem. 11 (2019), pp. 402–418. doi:https://doi.org/10.1038/s41557-019-0234-9.
- Z. Molavi, S. Razi, S.A. Mirmotalebisohi, A. Adibi, S. Mameni, F. Karami, V. Niazi, Z. Niknam, M. Aliashrafi, M. Taheri, S. Ghafouri-Fard, S. Jeibouei, S. Mahdian, H. Zali, M.M. Ranjbar, and M. Yazdani, Identification of FDA approved drugs against SARS-CoV-2 RNA dependent RNA polymerase (RdRp) and 3-chymotrypsin-like protease (3CLpro), drug repurposing approach, Biomed. Pharmacother. 138 (2021), pp. 111544. doi:https://doi.org/10.1016/j.biopha.2021.111544.
- M. Delijewski and J. Haneczok, AI drug discovery screening for COVID-19 reveals zafirlukast as a repurposing candidate, Med. Drug Discov. 9 (2021), pp. 100077. doi:https://doi.org/10.1016/j.medidd.2020.100077.
- C. Xu, Z. Ke, C. Liu, Z. Wang, D. Liu, L. Zhang, J. Wang, W. He, Z. Xu, Y. Li, Y. Yang, Z. Huang, P. Lv, X. Wang, D. Han, Y. Li, N. Qiao, and B. Liu, Systemic in silico screening in drug discovery for coronavirus disease (COVID-19) with an online interactive web server, J. Chem. Inf. Model. 60 (2020), pp. 5735–5745. doi:https://doi.org/10.1021/acs.jcim.0c00821.
- E. Di Salvo, V. Patella, M. Casciaro, and S. Gangemi, The leukotriene receptor antagonist Montelukast can induce adverse skin reactions in asthmatic patients, Pulm. Pharmacol. Ther. 60 (2020), pp. 101875. doi:https://doi.org/10.1016/j.pupt.2019.101875.
- S.J. Keam, K.A. Lyseng-Williamson, and K.L. Goa, Pranlukast: A review of its use in the management of asthma, Drugs 63 (2003), pp. 991–1019. doi:https://doi.org/10.2165/00003495-200363100-00005.
- H.M. Al-Kuraishy, A.I. Al-Gareeb, Y.Q. Almulaiky, N. Cruz-Martins, and G. El-Saber Batiha, Role of leukotriene pathway and montelukast in pulmonary and extrapulmonary manifestations of Covid-19: The enigmatic entity, Eur. J. Pharmacol. 904 (2021), pp. 174196. doi:https://doi.org/10.1016/j.ejphar.2021.174196.
- J. Barré, J.M. Sabatier, and C. Annweiler Cédric, Montelukast drug may improve COVID-19 prognosis: A review of evidence, Front. Pharmacol. 11 (2020), pp. 1344. doi:https://doi.org/10.3389/fphar.2020.01344.
- M. Dey and R.K. Singh, Possible therapeutic potential of cysteinyl leukotriene receptor antagonist montelukast in treatment of SARS-CoV-2-induced COVID-19, Pharmacology 106 (2021), pp. 469–476. doi:https://doi.org/10.1159/000518359.
- A.R. Khan, C. Misdary, N. Yegya-Raman, S. Kim, N. Narayanan, S. Siddiqui, P. Salgame, J. Radbel, F. Groote, C. Michel, J. Mehnert, C. Hernandez, T. Braciale, J. Malhotra, M.A. Gentile, and S.K. Jabbour, Montelukast in hospitalized patients diagnosed with COVID-19, J. Asthma (2021), PP. 1–7, Advance online publication. doi:https://doi.org/10.1080/02770903.2021.1881967.
- K. Imamura, Y. Sakurai, T. Enami, R. Shibukawa, Y. Nishi, A. Ohta, T. Shu, J. Kawaguchi, S. Okada, T. Hoenen, J. Yasuda, and H. Inoue, iPSC screening for drug repurposing identifies anti-RNA virus agents modulating host cell susceptibility, FEBS Open Bio. 11 (2021), pp. 1452–1464. doi:https://doi.org/10.1002/2211-5463.13153.
- W.J. Calhoun, Summary of clinical trials with zafirlukast, Am. J. Respir Crit. Care Med. 157 (1998), pp. S238–S246. doi:https://doi.org/10.1164/ajrccm.157.6.mar6.