ABSTRACT
Preeclampsia is a severe complication of pregnancy, affecting an estimated 4 million women annually. It is one of the leading causes of maternal and fetal mortality worldwide, and it has life-long consequences. The maternal multisystemic symptoms are driven by poor placentation, which causes syncytiotrophoblastic stress and the release of factors into the maternal bloodstream. Amongst them, the soluble fms-like tyrosine kinase-1 (sFLT-1) triggers extensive endothelial dysfunction by acting as a decoy receptor for the vascular endothelial growth factor (VEGF) and the placental growth factor (PGF). Current interventions aim to mitigate hypertension and seizures, but the only definite treatment remains induced delivery. Thus, there is a pressing need for novel therapies to remedy this situation. Notably, CBP-4888, a siRNA drug delivered subcutaneously to knock down sFLT1 expression in the placenta, has recently obtained Fast Track approval from the Food and Drug Administration (FDA) and is undergoing a phase 1 clinical trial. Such advance highlights a growing interest and significant potential in gene therapy to manage preeclampsia. This review summarizes the advances and prospects of gene therapy in treating placental dysfunction and illustrates crucial challenges and considerations for these emerging treatments.
Introduction
Preeclampsia is a severe pregnancy-related syndrome affecting approximately 3–5% of pregnancies worldwide (Citation1). The disease presents with sudden-onset hypertension and proteinuria or other end-organ damage. It is the second cause of maternal death in developing countries, killing approximately 50,000 pregnant women (Citation2). The affected mothers will have a higher risk of long-term adverse health outcomes, including stroke, cardiovascular disease, kidney disease, and metabolic disorder (Citation3–5). On the fetal side, preeclampsia is often associated with fetal growth restriction (FGR), perinatal death, and preterm delivery, as well as a higher susceptibility to developing hypertension, diabetes, and neurological impairment in adulthood (Citation4,Citation6,Citation7). Although preeclampsia has long been described in the medical literature, our understanding of its pathogenesis remains uncertain, and clinical interventions are limited. Clinical management mainly mitigates hypertension and seizures, while aspirin is used for prevention with limited efficiency (Citation8). The only definite treatment remains delivery, which is often associated with induced prematurity and its own complications. Although the disease poses a significant social and economic burden, effective therapeutics are still missing.
During the first trimester of pregnancy, the progenitor cytotrophoblasts (CTB) localized within the anchoring villi differentiate into extravillous trophoblasts (EVT) and acquire an invasive phenotype. The EVTs migrate into the maternal decidua and replace the smooth muscle cells of the spiral and radial arteries to ensure adequate perfusion of the fetoplacental unit throughout gestation (Citation9,Citation10). Defective vascular remodeling is thought to cause inadequate oxygen supply to the placenta and induce subsequent syncytiotrophoblast stress (Citation11,Citation12). Cellular responses are various and include unfolded protein response, metabolic adaptations, apoptosis, and the release of different factors into the maternal circulation, such as antiangiogenic factors (soluble fms-like tyrosine kinase-1 (sFLT-1), soluble endoglin (sENG)), proinflammatory cytokines, exosomes, and extracellular vesicles (Citation8,Citation13,Citation14). These factors provoke systemic endothelial dysfunction, causing hypertension and maternal multi-organ dysfunction.
Significant advances in gene therapy have been approved and translated into clinical settings in recent years, but none for preeclampsia. Gene therapy involves transferring genetic materials into the target cells to achieve a therapeutic effect. Thus, it implies two things to generate appropriate vectors: a complete understanding of the disease pathophysiology and the assumption that the disease is not multifactorial but caused by only one dysfunctional pathway. Although placental dysfunction is a multifaceted syndrome, several targets have been recently identified. Amongst them, silencing sFLT-1 is a promising strategy, and the CBP-4888 was last July approved by the Food and Drug Administration (FDA) for phase 1 clinical trials (Citation15).
This review discusses the basics of gene therapy, including its history, recent advances, and applications in placenta dysfunction. We included findings from in vitro models and data from animals. Finally, we discussed the advances in vector design and gene delivery, especially placenta-specific delivery systems, and the future blueprint of gene therapy for mitigating placental dysfunction.
An overview of the gene therapy
History
Both successes and failures marked the last three decades of gene therapy. In 1990, the first approved and successful treatment constited in direct insertion of the adenosine deaminase (ADA) gene into the T cells of a girl with severe combined immunodeficiency through a viral vector (Citation16). This clinical case validated the basis of gene therapy, but its development was slowed by severe side effects, such as insertional mutagenesis and immune reactions to adenovirus (Citation17,Citation18). To date, 59 gene therapies have been approved for clinical use, covering diseases in the retina, liver, muscle, and hematopoietic system. However, these therapies are still limited by their poor delivery and specificity of cellular targeting (Citation19,Citation20). Approximately 2000 gene therapies are currently being developed for clinical application, mainly for cancer, infections, and orphan diseases (Citation19). We have undoubtedly entered the modern era of gene therapy, and much progress is expected.
Strategies
Gene therapy consists of using genetic materials (DNA or RNA) to target a specific gene to prevent or treat disease by increasing or decreasing its expression or editing its sequence (Citation21). Increasing expression aims to use a standard copy of an allele under the control of a constitutive promoter in a plasmid, for instance, while gene suppression refers to reducing the expression by inhibitory tools such as antisense oligonucleotides and RNA interference by small interfering RNA (siRNA) or micro RNA (miRNA). Small interfering RNA can trigger more efficient and specific gene silencing, while miRNA may influence the expression of several target genes at the same time (Citation22).
For gene editing, the strategy seeks to modify the DNA sequence to correct the produced dysfunctional protein. This process can be achieved by using the CRISPR/Cas-9 system, a valuable and effective tool for gene editing, as well as a milestone in the development of gene therapy (Citation21,Citation23). The CRISPR system was initially discovered in prokaryotes as a defense mechanism for adaptive immune response. In this system, single guide RNAs (sgRNA) guide the Cas-9 nuclease to a specific location in the genome, thereby inducing double-strand breaks (DSBs). In the absence of exogenous DNA, the cell will repair the DSBs by non-homologous end joining, which results in random insertion or deletion at the cleavage site. This error-prone mechanism mostly leads to a frameshift mutation or premature stop codon, thus gene knock-out. If exogenous DNA is present, the DSBs are repaired by homology-directed insertion of a specific sequence. This system allows a precise gene edition or activation (Citation24).
Delivery
The critical step for efficient gene therapy is using vectors to deliver the genetic cargo to the chosen organ. According to vector type, transfer systems can be divided between viral-mediated and non-viral-mediated systems.
Viral vectors include adenovirus, adeno-associated virus (AAV), and lentivirus. Adenovirus is an icosahedral capsid containing internal double-stranded DNA, able to infect both quiescent and proliferating cells (Citation25). After endocytosis of the viral particle, the genetic material remains in the nucleus without integration into the host genome. The advantages of adenoviral vectors include high transduction efficiency, epichromosomal persistence, and the ability to transfect almost all cell types. However, the potent immunogenicity of these adenoviral particles hampers their use in humans (Citation26,Citation27). Discovered in the 1960s during adenovirus production, AAV is the smallest vector used in gene therapy. Thanks to its reduced immunogenicity and toxicity, recombinant AAV (rAAV) has emerged as a promising and prominent vector to enable stable long-term transgene expression (Citation27). Lastly, the lentiviral system was derived from the human immunodeficiency virus (HIV)-1 to incorporate the transgene into the human genome. Hence, lentiviral vectors can transduce postmitotic and quiescent cells and permit long-term transgene expression. This vector type is now mainly employed in manufacturing modified T cells in cancer therapy (Citation27).
Although viral vectors were initially preferred because of their higher transduction efficiency and ease of engineering, concerns were raised about their immunogenicity and oncogenic capacity (Citation28). Therefore, developing non-viral vectors has recently gained momentum as they offer low manufacturing costs and modifiable structural components. The non-viral vectors include five main types: lipid nanoparticles (LNP), polymeric nanoparticles (such as PAMAM, PDMAEMA, and PAEs), inorganic nanoparticles (such as quantum dots, gold nanoparticles, silica nanoparticles, and carbon nanotubes), virus-like particles, and extracellular vesicles. These vectors are less common but have shown a safer immunogenicity profile and higher cargo-packing capacity than viral systems (Citation29). Although transfer efficiency, target specificity, and lasting gene expression remain challenging, non-viral vectors have already been used in clinical trials and constitute the future of precision medicine. Notably, non-viral vectors predominate over viral vectors for CRISPR/Cas-9 delivery according to the results of preclinical and clinical trials (Citation30).
Administration
Administration roads can be divided into in vivo and ex vivo methods. Many in vivo methods have been tested to optimize gene therapy efficiency. Published and ongoing trials include subcutaneous, intravenous, intradermal, intratumoral, and intravitreal injections and eye drops. In the last five years, subcutaneous and intravenous administration accounted for the majority (Citation31). In ex vivo methods, the cells are taken from patients, cultured, and treated in the lab with gene therapy and then put back into the patient (Citation32). This method is mainly used to manage patients with blood disorders and hematologic malignancies (Citation33).
Applications in human diseases
Gene therapy will undoubtedly become essential in human medicine. It has already been proven during the SARS-CoV-2 pandemic, and a growing number of treatments are being approved by regulatory agencies such as the FDA and EMA. Since these methods can edit genome sequences, they have found unlimited applications in genetic disorders. For instance, siRNA-based therapeutics are already approved for treating hereditary transthyretin amyloidosis, acute intermittent porphyria, primary hyperoxaluria type 1, and hypercholesterolemia, and many clinical trials are still ongoing (Citation31).
Regarding CRISPR/Cas-9, the technology promises to cure important genetic diseases such as sickle cell disease, β-thalassemia, cystic fibrosis, and muscular dystrophy. Notably, the first-ever CRISPR-based therapeutic (Casgevy and Lyfgenia) was approved for treating β-thalassemia and sickle cell anemia in the USA and the UK (Citation34,Citation35). Interestingly, gene therapy also finds applications in cell therapy, as illustrated by engineered T lymphocytes use in refractory cancer (CAR T cells) (Citation36).
Gene therapy in placental dysfunction
Targeting the placenta
Effective therapies for placental dysfunction remain limited compared to other major health problems because of the inherent complexity of pregnancy and the risk of unknown long-term consequences for the fetus (Citation37). The only accepted intervention for preeclampsia is the prophylactic administration of aspirin before the end of the first trimester. Other potential therapies included statins or phosphodiesterase inhibitors, but these small molecules easily cross the placenta and reach the fetus, eventually leading to unexpected adverse effects (Citation38). Thus, current research aims to develop placenta-specific drug delivery to reduce fetal exposure while maximizing efficiency ().
Figure 1. Placenta-specific drug delivery systems. Targeting the trophoblasts is a crucial point in the treatment of placental dysfunction. Though adenovirus was prevalent as a vector in gene therapy for fetal growth restriction, nanoparticles are the emerging method in gene therapy nowadays, especially for placenta-originated diseases. Nanoparticles are economical, accessible, and have lower immunogenicity and larger capacity. However, there is a long way to go in the research in the molecular size and shape, surface charge, targeting ligands, and material composition for the perfect placenta-specific drug delivery systems. Figure created with Biorender.
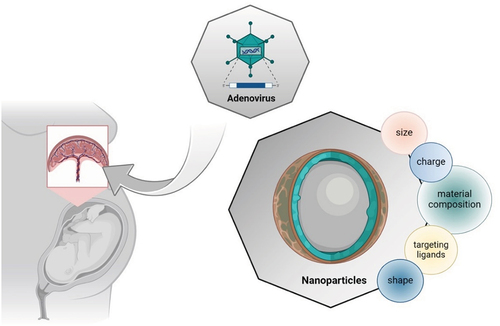
Viral vectors
Viral vectors have received widespread attention in research on placenta-targeted drug delivery systems in recent years. Amongst them, adenovirus-mediated placental therapy has proven effective in in vitro and in vivo models, including trophoblast cell lines, rodents, sheep, and primates (Citation39). These studies have consistently demonstrated that adenoviral delivery of vectors expressing the insulin-like growth factor (IGF-1) and the vascular endothelial growth factor (VEGF) could improve fetal growth and prevent FGR-induced cardiac dysfunction (Citation40–42). Although adenovirus administration has been improved with larger capacity, reduced immunogenicity, and prolonged transduction, concerns remain about their long-term safety in exposed fetuses.
Non-viral vectors
Because of safety concerns regarding viral vectors, the non-viral systems have also been investigated for placenta-targeted drug delivery. They also present substantial advantages, such as immunological safety and spontaneous accumulation in the placenta, following the physiological adaptations of the maternal cardiovascular system during pregnancy (Citation43). Nanoparticles, including lipid nanoparticles, polymeric nanoparticles, and inorganic nanoparticles, can target desired cells or tissues in four ways: paracellular pathway, endocytosis, exocytosis, and transporter-mediated uptake (Citation43). The application of nanotechnology to placenta-targeted delivery has been subjected to intense research during the last several years (Citation44). In 2017, Cureton et al. designed a peptide CNKGLRNK-decorated liposome located almost exclusively in the intima of the decidual spiral arteries and the vasculature of the mouse placental labyrinth zone. Via tail vein injection, they obtained a significant therapeutic effect, showing the potential of their engineered liposomal placenta-targeted vectors (Citation45). In the same idea, Zhang et al. developed a placental chondroitin sulfate A binding peptide (plCSA-BP)-guided nanoparticles able to specifically bind with trophoblast without apparent effect on maternal tissues in mice (Citation46). The contrast-enhanced ultrasound (CEU)-mediated lipid nanoparticle encapsulated microbubble (MB) showed the ability to carry VEGF DNA and estradiol into the basal plate in baboons, achieving uterine artery remodeling, providing a novel strategy in gene therapy for placental dysfunction (Citation47).
At last, it is possible to modify the substrate (nanoplatforms), adjust the placental microenvironment (pH, for instance), or construct nanoplatforms with a lysosomal escape to improve accumulation and retention, which are both pivotal to enhance efficacy and reduce the side effects (Citation48–50). For example, Fein et al. reported that larger polymers had lower permeability and polyethylene glycol permeating the placenta to a greater extent than dextrans with the same weight in BeWo cell line and pregnant CD1 mice, suggesting that molecular size, chemistry, and conformation are essential variables in placental drug delivery (Citation51). Unfortunately, numerous characteristics of non-viral vectors, including their toxicity and capability to penetrate the placental barrier, remain elusive, and further explorations are necessary for a better characterization of these strategies before their use in humans.
Potential targets
Despite all its advantages, gene therapy requires a thorough understanding of the disease to identify the right target. However, the pathophysiology of placental dysfunction and its consequences are far from being characterized. The field is moving fast, and some pathways have already shown their potential. Modulating their activity in a therapeutic strategy is mainly based on siRNA, with some using overexpression ().
Table 1. Representative studies exploring gene therapy in placental dysfunction.
The angiogenic balance
In normal pregnancy, the placenta secretes a high amount of placenta growth factor (PGF) in the maternal circulation to promote the binding of VEGF to its receptor VEGFR-2 instead of VEGFR-1. Preferential activation of VEGFR-2 in the endothelial cells is important for appropriate vascular adaptations of the mother. In preeclampsia, high levels of circulating sFLT-1 in the maternal circulation are known to scavenge PGF and VEGF, resulting in decreased VEGFR-2 signaling and extensive endothelial dysfunction (Citation4). Therefore, an increased sFLT-1/PGF ratio is often referred to as the “angiogenic imbalance” and is a predictive marker of developing preeclampsia (Citation60). Injecting sFLT-1 induces preeclampsia in pregnant rodents, while a pilot clinical study in humans demonstrated that removing sFLT-1 by apheresis can mitigate endothelial dysfunction and delay delivery (Citation61). Although apheresis is not accessible for large-scale clinical applications owing to its high price and complex requirements, it suggests that correcting the angiogenic imbalance could be a powerful therapeutic lever, offering a specific target suitable for gene therapy.
In 2017, Yu et al. developed a siRNA-sflt1-poly-amidoamine complex that suppressed sFLT1 expression and secretion in HTR-8/SVneo cells and improved pregnancy outcomes and maternal symptoms (mean arterial pressure and proteinuria) in a rat model of preeclampsia (Citation52). The year after, Turanov’s team selectively silenced three sFLT1 mRNAs responsible for sFLT-1 overexpression in the placenta without influencing the expression of full-length FLT1 mRNA by using cholesterol siRNA conjugation. They successfully suppressed the clinical signs of placental dysfunction without adverse effects on fetuses in pregnant mice and baboons (Citation53). After this landmark proof-of-concept paper, several studies were published, mainly aiming to optimize the delivery system to improve the outcomes and reduce the side effects (Citation54–56). This work led to the development of the subcutaneously delivered sFLT-1 silencing drug CBP-4888, which obtained FDA Fast Track approval in August 2023 to undergo the phase 1 clinical trial. This drug is a fixed-dose combination of two chemically synthesized, lipid-conjugated siRNA duplex oligonucleotides (siRNA-2283 and siRNA-2519) targeting two sFLT1 mRNA isoforms to specifically knock down sFLT1 expression in the placenta (Citation62).
On the other side of the balance, VEGF was also considered another crucial molecule for the health of the endothelium, from the renal glomerulus to the brain and liver, as well as a crucial protein in FGR (Citation63,Citation64). Previous research reported that sFLT-1-induced preeclampsia in rodents was mitigated by injecting recombinant VEGF (Citation65). Thus, the potential of VEGF-based gene therapy was acknowledged, and a 6-year prospective study aiming to assess the safety and efficacy of an adenovirus-carrying VEGF (Ad.VEGF) therapy in severe early-onset FGR was started (the EVERREST Project, NCT02097667) (Citation66). Initially developed for FGR, Ad.VEGF therapy uses adenoviral vectors to overexpress VEGF-A and VEGF-D as they have the strongest angiogenic potency among the VEGF family (Citation67). In 2020, Rossi et al. demonstrated the potential of VEGF-A and -D adenovirus conjugation in treating placental dysfunction by showing their ability to induce tube formation in endothelium cells from humans, sheep, guinea pigs, and rabbits (Citation68). Recently, Kelsey et al. transferred VEGFA mRNA encapsulated in ionizable lipid nanoparticles into trophoblast cell line JEG-3 and mice placentas, showing that VEGFA mRNA alone was sufficient to mediate placental vasodilation and, therefore, potentially treat placental insufficiency (Citation57).
The insulin growth factors
Fetal growth restriction is a consequence of placental dysfunction and is often associated with hypertension in preeclampsia. In this regard, insulin growth factors (IGF), secreted by the placenta during gestation, are associated with trophoblast development, and their expression is positively correlated with fetal growth. There is growing evidence that targeted delivery of IGFs improves fetal growth. For instance, Jones’ team extensively studied IGF1 overexpression in in vitro and in vivo settings to treat FGR (Citation69). They encapsulated plasmids expressing IGF1 under the control of the trophoblast-specific promoter CYP19A1 with a non-viral PHPMA115-b-PDMEAMA115 co-polymer. They demonstrated that their nanoparticles improved EVT differentiation, vascular remodeling, and glucose and amino acid transport capacities in guinea pigs (Citation59). Similarly, the targeted delivery of liposomes-IGF-2 protein to the placenta in an FGR mouse model improved placental weight and reduced the number of FGR fetuses, confirming the potential of this pathway (Citation70). Although initially developed for FGR, these strategies show high promise for treating mild placental dysfunction and could be of interest in more dysfunctional placentas, as seen in preeclampsia.
Micro RNAs
MicroRNAs (miRNAs) are a cluster of short and non-coding RNA, focusing on post-transcriptional regulation of gene expression and participating in diverse pathological processes. Unlike siRNA, miRNA can target multiple mRNAs simultaneously, thus modulating several signaling pathways. Evidence suggests that endogenous miRNAs play an essential role in placental transcriptomics and are pivotal causes of preeclampsia. Indeed, miRNAs regulate cellular processes (such as trophoblast differentiation, proliferation, migration, and invasion) and the expression of angiogenesis-related factors, including sFLT-1 and VEGF (Citation71). For example, placental expression of miR-210, miR-126, and miR-148/152 statistically differ between normotensive and preeclampsia patients, suggesting their potential as diagnostic tools or therapeutic targets (Citation72). Beards et al. demonstrated that miR-145 or miR-675 inhibitor peptide nucleic acid conjugate promoted cytotrophoblast proliferation in human first-trimester placental explants and increased fetal and placental weight in mice models (Citation73). Although no miRNA-based drugs have been approved to treat placental dysfunction, several miRNA-based therapeutics against genetic, metabolic, and oncological diseases are undergoing clinical trials (Citation74). It is not excluded that, due to the similarities between trophoblast differentiation and tumor development, anti-cancer miRNA-based therapeutics will also offer valuable options for preeclampsia shortly (Citation75).
The renin-angiotensin-aldosterone system
Because of the proteinuria, it has been speculated that the renin-angiotensin-aldosterone system (RAAS) could play a role in preeclampsia. Although all the authors do not share this vision, animal models displaying excessive RAAS activation lead to preeclampsia-like phenotypes (Citation76,Citation77). Angiotensinogen (AGT) mutants are linked to hypertension in pregnancy, and reducing angiotensinogen levels in the blood by lipid nanoparticles encapsulated siRNA lowered blood pressure in spontaneous hypertensive Sprague-Dawley rats (Citation78). In 2020, Haase et al. used N-acetylgalactosamine-siRNA targeting Agt in the reduced uterine perfusion pressure (RUPP) model, a rat model of preeclampsia. The results showed that reducing Agt expression mitigated maternal hypertension and improved fetal outcomes without crossing the placental barrier, suggesting that RAAS could be usefully inhibited to treat the manifestations of placental dysfunction (Citation58).
Pitfalls and conclusion
Although defective placentation is the primum movens of preeclampsia, the disease remains multifactorial, and the current research aims to target various adaptative mechanisms of the syncytium. Since the thalidomide tragedy, it is now acknowledged that the placenta is not a sanctuary for the fetus but a complex and dynamic interface between the maternal and fetal circulations. The systematic testing for developmental toxicity and our imperfect understanding of placental biology significantly slow down the advent of gene therapy in our field.
Model heterogeneity
Given the unique nature of the human placenta and ethical concerns, obstetric medicine has always suffered from the lack of a valid research model. Commonly used trophoblast cell lines like HTR-8/SVneo or BeWo oversimplify the research, while primary cytotrophoblasts are challenging to isolate and prone to variations between experiments. The human trophoblast-derived organoids offer substantial advantages compared with 2D-culture models but are limited by the operational variability and the lack of uterine microenvironment (Citation79). Animals offer a much more comprehensive reflection of the interactions and responses within an entire biological system, but even if placentas share many standard functions, they are arguably the least conserved and most rapidly evolving mammalian organs (Citation80). Rodents and rabbits are commonly used for studying placental development and associated diseases, yet significant differences exist between human and rodent placentas in structure and functioning (Citation81). For instance, mice have a relatively short gestation period and shallower placental invasion, which limits their utility in studying placental transport efficiency and permeability. Inter-species variations in genetic material, epigenetic modifications, and regulatory molecular networks inevitably limit the reliability and translatability of the findings to humans. Even the non-human primates (except gorillas and chimpanzees), our closest relatives with hemochorial placentation, do not show deep trophoblast invasion and hence do not spontaneously develop placental dysfunction (Citation82). Thus, promising compounds can sometimes show strong efficiency in vitro and preclinical studies but turn out to be disappointing in humans (Citation83).
Drug safety
Specific delivery of genetic information to the trophoblasts remains the main obstacle, but it is also an unprecedented opportunity to reduce adverse effects for the fetus. The placenta imposes constraints on the transport of large molecules, making the molecular size and shape, targeting ligands, surface charge, and material composition critical factors influencing therapeutic outcomes (Citation43). The number of gestational weeks should also be considered when designing the drug and delivery system due to the evolving structure of the placenta. For the viral systems, safety issues mainly concern immunogenicity, especially for adenoviral vectors and epigenetic effects, even for nonintegrating vectors (Citation84). Regarding the nanoparticles, the characterization of their safety profile is improving, but most evidence is derived from cell and animal models. For instance, a multi-layered BeWo model revealed that cobalt and chromium nanoparticles penetrated cell barriers and damaged adjacent cell layers (Citation85). In mice, liver and neuronal toxicity were found in fetuses, although no pathological changes were evident in the placentas (Citation86). Further research is needed before translating to humans, and each type of non-viral vector must be independently tested for harmlessness. However, information about the safety and large-scale feasibility of DNA and mRNA cargo administration to the pregnant population was gained from the different vaccines against SARS-CoV-2 (Citation87). Complementary to animal studies, rehabilitating the ex vivo perfusion of human cotyledons could bring new data about the pharmacokinetics and capture of the cargos developed for placental gene delivery (Citation88,Citation89).
Prospects and challenges
Despite its substantial benefits, integrating gene therapy into clinical practice will encounter several challenges. As exposed before, safety still must be monitored in humans, but carrying out clinical trials in pregnant women remains costly and ethically questionable, notably regarding the potential long-term impacts on the fetal genome. Cost is also a limiting factor, especially when identifying new therapeutic targets. However, the COVID period proved that developing manufacturing chains for gene therapy was feasible and economically profitable. For instance, the anti-sFLT-1 siRNA CBP-4888 will likely be much more affordable and accessible to implement in large groups of patients than sFLT-1 apheresis (Citation61).
Conclusion
The decades of research in preeclampsia are paying off, although further insights into the molecular mechanisms remain necessary. The approval of CBP-4888 is the most advanced therapy and represents unprecedented hope for this incurable disease. We are likely at the dawn of a new era of treatments, providing specific, safe, and effective drugs for placental dysfunction.
Acknowledgments
We gratefully acknowledge the financial support provided to our research projects by the FNRS (Fonds de la Recherche Scientifique - National Fund for Scientific Research). We also thank the Chinese Research Council (CSC) and the Fonds pour la Formation à la Recherche dans l’Industrie et dans l’Agriculture (FRIA) for supporting our fellows (F.S., M.P., A.C.). We extend our appreciation to the private charities Fondation Saint-Luc, Fetus for Life, and Vocatio for their ongoing commitment to young researchers and advancing scientific research.
Disclosure statement
No potential conflict of interest was reported by the author(s).
Data availability statement
The authors confirm that the data supporting the findings of this study are available in the cited articles.
Additional information
Funding
References
- Magee LA, Brown MA, Hall DR, et al. The 2021 international society for the study of hypertension in pregnancy classification, diagnosis & management recommendations for international practice. Pregnancy Hypertens. 2022;27:148–11. doi: 10.1016/j.preghy.2021.09.008
- Say L, Chou D, Gemmill A, et al. Global causes of maternal death: a WHO systematic analysis. Lancet Glob Health. 2014;2(6):323–333. doi: 10.1016/S2214-109X(14)70227-X
- Thilaganathan B, Kalafat E. Cardiovascular system in preeclampsia and beyond. Hypertension. 2019;73(3):522–531. doi: 10.1161/HYPERTENSIONAHA.118.11191
- Bokuda K, Ichihara A. Preeclampsia up to date-what’s going on? Hypertens Res. 2023;46(8):1900–1907. doi: 10.1038/s41440-023-01323-w
- Hung SK, Lee M-S, Lin H-Y, et al. Impact of hypertensive disorders of pregnancy on the risk of stroke stratified by subtypes and follow-up time. Stroke. 2022;53(2):338–344. doi: 10.1161/STROKEAHA.121.034109
- Chappell LC, Cluver CA, Kingdom J, et al. Pre-eclampsia. Lancet. 2021;398(10297):341–354. doi: 10.1016/S0140-6736(20)32335-7
- Colella M, Frérot A, Novais ARB, et al. Neonatal and long-term consequences of fetal growth restriction. Curr Pediatr Rev. 2018;14(4):212–218. doi: 10.2174/1573396314666180712114531
- Dimitriadis E, Rolnik DL, Zhou W, et al. Pre-eclampsia. Nat Rev Dis Primers. 2023;9(1):8. doi: 10.1038/s41572-023-00417-6
- Chang CW, Wakeland AK, Parast MM. Trophoblast lineage specification, differentiation and their regulation by oxygen tension. J Endocrinol. 2018;236(1):R43–r56. doi: 10.1530/JOE-17-0402
- James JL, Carter AM, Chamley LW. Human placentation from nidation to 5 weeks of gestation. Part I: What do we know about formative placental development following implantation? Placenta. 2012;33(5):327–334. doi: 10.1016/j.placenta.2012.01.020
- Staff AC. The two-stage placental model of preeclampsia: An update. J Reprod Immunol. 2019;134-135:1–10. doi: 10.1016/j.jri.2019.07.004
- Colson A, Sonveaux P, Debiève F, et al. Adaptations of the human placenta to hypoxia: opportunities for interventions in fetal growth restriction. Hum Reprod Update. 2021;27(3):531–569. doi: 10.1093/humupd/dmaa053
- Redman CWG, Staff AC, Roberts JM. Syncytiotrophoblast stress in preeclampsia: the convergence point for multiple pathways. Am J Obstet Gynecol. 2022;226(2s):S907–s927. doi: 10.1016/j.ajog.2020.09.047
- Deer E, Herrock O, Campbell N, et al. The role of immune cells and mediators in preeclampsia. Nat Rev Nephrol. 2023;19(4):257–270. doi: 10.1038/s41581-022-00670-0
- Tyrmi JS, Kaartokallio T, Lokki AI, et al. Genetic risk factors associated with preeclampsia and hypertensive disorders of pregnancy. JAMA Cardiol. 2023;8(7):674–683. doi: 10.1001/jamacardio.2023.1312
- Blaese RM, Culver KW, Miller AD, et al. T lymphocyte-directed gene therapy for ADA − SCID: Initial trial results after 4 years. Science. 1995;270(5235):475–480. doi: 10.1126/science.270.5235.475
- Couzin J, Kaiser J. Gene therapy. As Gelsinger case ends, gene therapy suffers another blow. Science. 2005;307(5712):1028. doi: 10.1126/science.307.5712.1028b
- Hacein-Bey-Abina S, Garrigue A, Wang GP, et al. Insertional oncogenesis in 4 patients after retrovirus-mediated gene therapy of SCID-X1. J Clin Invest. 2008;118(9):3132–3142. doi: 10.1172/JCI35700
- David Barrett SM, Wendland A, Rose D, et al. Gene, cell, and RNA therapy landscape: Q4 2023 quarterly data report. 2023.
- Peek JL, Wilson MH. Cell and gene therapy for kidney disease. Nat Rev Nephrol. 2023;19(7):451–462. doi: 10.1038/s41581-023-00702-3
- Anguela XM, High KA. Entering the modern era of gene therapy. Annu Rev Med. 2019;70(1):273–288. doi: 10.1146/annurev-med-012017-043332
- Hu B, Zhong L, Weng Y, et al. Therapeutic siRNA: state of the art. Signal Transduct Target Ther. 2020;5(1):101. doi: 10.1038/s41392-020-0207-x
- Zhang Y, Wu Z-Y. Gene therapy for monogenic disorders: challenges, strategies, and perspectives. J Genet Genome. 2023.
- Chavez M, Chen X, Finn PB, et al. Advances in CRISPR therapeutics. Nat Rev Nephrol. 2023;19(1):9–22. doi: 10.1038/s41581-022-00636-2
- Gao J, Zhang W, Ehrhardt A. Expanding the spectrum of adenoviral vectors for cancer therapy. Cancers (Basel). 2020;12(5):12(5. doi: 10.3390/cancers12051139
- Muruve DA. The innate immune response to adenovirus vectors. Hum Gene Ther. 2004;15(12):1157–1166. doi: 10.1089/hum.2004.15.1157
- Bulcha JT, Wang Y, Ma H, et al. Viral vector platforms within the gene therapy landscape. Signal Transduct Target Ther. 2021;6(1):53. doi: 10.1038/s41392-021-00487-6
- Arabi F, Mansouri V, Ahmadbeigi N. Gene therapy clinical trials, where do we go? An overview. Biomed Pharmacother. 2022;153:113324. doi: 10.1016/j.biopha.2022.113324
- Patil S, et al. The development of functional non-viral vectors for gene delivery. Int J Mol Sci. 2019;20(21):20(21. doi: 10.3390/ijms20215491
- Sainz-Ramos M, Gallego I, Villate-Beitia I, et al. How far are non-viral vectors to come of age and reach clinical translation in gene therapy? Int J Mol Sci. 2021;22(14):7545. doi: 10.3390/ijms22147545
- Corydon IJ, Fabian-Jessing BK, Jakobsen TS, et al. 25 years of maturation: A systematic review of RNAi in the clinic. Mol Ther Nucleic Acids. 2023;33:469–482. doi: 10.1016/j.omtn.2023.07.018
- Herzog RW, Popplewell L. Gene Therapy. In: S. Karger, editor. Fast Facts. 2020. doi: 10.1159/isbn.978-3-318-06667-8
- Wu X, He X, Liu F, et al. Development and clinical translation of ex vivo gene therapy. Comput Struct Biotechnol J. 2022;20:2986–3003. doi: 10.1016/j.csbj.2022.06.015
- Parums DV. Editorial: First regulatory approvals for CRISPR-Cas9 therapeutic gene editing for sickle cell disease and transfusion-dependent beta-thalassemia. Med Sci Monit. 2024;30:e944204. doi: 10.12659/MSM.944204
- Frangoul H, Altshuler D, Cappellini MD, et al. CRISPR-Cas9 gene editing for sickle cell disease and β-thalassemia. N Engl J Med. 2021;384(3):252–260. doi: 10.1056/NEJMoa2031054
- Stadtmauer EA, Fraietta JA, Davis MM, et al. CRISPR-engineered T cells in patients with refractory cancer. Science. 2020;367(6481). doi: 10.1126/science.aba7365
- Stock SJ, Norman JE. Medicines in pregnancy. F1000Res. 2019;8:8. doi: 10.12688/f1000research.17535.1
- Pels A, Onland W, Berger RMF, et al. Neonatal pulmonary hypertension after severe early-onset fetal growth restriction: post hoc reflections on the Dutch STRIDER study. Eur J Pediatr. 2022;181(4):1709–1718. doi: 10.1007/s00431-021-04355-x
- Pepe GJ, Albrecht ED. Novel technologies for target delivery of therapeutics to the Placenta during pregnancy: a review. Genes (Basel). 2021;12(8):1255. doi: 10.3390/genes12081255
- Jones H, Crombleholme T, Habli M. Regulation of amino acid transporters by adenoviral-mediated human insulin-like growth factor-1 in a mouse model of placental insufficiency in vivo and the human trophoblast line BeWo in vitro. Placenta. 2014;35(2):132–138. doi: 10.1016/j.placenta.2013.11.012
- Keswani SG, Balaji S, Katz AB, et al. Intraplacental gene therapy with Ad-IGF-1 corrects naturally occurring rabbit model of intrauterine growth restriction. Hum Gene Ther. 2015;26(3):172–182. doi: 10.1089/hum.2014.065
- Swanson AM, Rossi CA, Ofir K, et al. Maternal therapy with Ad.VEGF-A 165 increases fetal weight at term in a guinea-pig model of fetal growth restriction. Hum Gene Ther. 2016;27(12):997–1007. doi: 10.1089/hum.2016.046
- Tang M, Zhang X, Fei W, et al. Advance in placenta drug delivery: concern for placenta-originated disease therapy. Drug Deliv. 2023;30(1):2184315. doi: 10.1080/10717544.2023.2184315
- Pritchard N, Kaitu’u-Lino T, Harris L, et al. Nanoparticles in pregnancy: the next frontier in reproductive therapeutics. Hum Reprod Update. 2021;27(2):280–304. doi: 10.1093/humupd/dmaa049
- Cureton N, Korotkova I, Baker B, et al. Selective targeting of a novel vasodilator to the uterine vasculature to treat impaired uteroplacental perfusion in pregnancy. Theranostics. 2017;7(15):3715–3731. doi: 10.7150/thno.19678
- Zhang B, Tan L, Yu Y, et al. Placenta-specific drug delivery by trophoblast-targeted nanoparticles in mice. Theranostics. 2018;8(10):2765–2781. doi: 10.7150/thno.22904
- Babischkin JS, Aberdeen GW, Lindner JR, et al. VasculaR endothelial growth factor delivery to placental basal plate promotes uterine artery remodeling in the primate. Endocrinology. 2019;160(6):1492–1505. doi: 10.1210/en.2019-00059
- Luan S, Xie R, Yang Y, et al. Acid-responsive aggregated gold nanoparticles for radiosensitization and synergistic chemoradiotherapy in the treatment of esophageal cancer. Small. 2022;18(19):e2200115. doi: 10.1002/smll.202200115
- Zhang B, Liang R, Zheng M, et al. Surface-functionalized nanoparticles as efficient tools in targeted therapy of pregnancy complications. Int J Mol Sci. 2019;20(15):20(15. doi: 10.3390/ijms20153642
- Dahiya UR, Ganguli M. Exocytosis - a putative road-block in nanoparticle and nanocomplex mediated gene delivery. J Control Release. 2019;303:67–76. doi: 10.1016/j.jconrel.2019.04.012
- Fein KC, Arral ML, Kim JS, et al. Placental drug transport and fetal exposure during pregnancy is determined by drug molecular size, chemistry, and conformation. JControlled Release. 2023;361:29–39. doi: 10.1016/j.jconrel.2023.07.029
- Yu J, Jia J, Guo X, et al. Modulating circulating sFlt1 in an animal model of preeclampsia using PAMAM nanoparticles for siRNA delivery. Placenta. 2017;58:1–8. doi: 10.1016/j.placenta.2017.07.360
- Turanov AA, Lo A, Hassler MR, et al. RNAi modulation of placental sFLT1 for the treatment of preeclampsia. Nature Biotechnol. 2018;36(12):1164–1173. doi: 10.1038/nbt.4297
- Li L, Li H, Xue J, et al. Nanoparticle-mediated simultaneous downregulation of placental Nrf2 and sFlt1 improves maternal and fetal outcomes in a preeclampsia mouse model. ACS Biomater Sci Eng. 2020;6(10):5866–5873. doi: 10.1021/acsbiomaterials.0c00826
- Li L, Yang H, Chen P, et al. Trophoblast-targeted nanomedicine modulates placental sFLT1 for preeclampsia treatment. Front Bioeng Biotechnol. 2020;8:64. doi: 10.3389/fbioe.2020.00064
- Davis SM, Hariharan VN, Lo A, et al. Chemical optimization of siRNA for safe and efficient silencing of placental sFLT1. Mol Ther Nucleic Acids. 2022;29:135–149. doi: 10.1016/j.omtn.2022.06.009
- Swingle KL, Safford HC, Geisler HC, et al. Ionizable lipid nanoparticles for in vivo mRNA delivery to the placenta during pregnancy. J Am Chem Soc. 2023;145(8):4691–4706. doi: 10.1021/jacs.2c12893
- Haase N, Foster DJ, Cunningham MW, et al. RNA interference therapeutics targeting angiotensinogen ameliorate preeclamptic phenotype in rodent models. J Clin Invest. 2020;130(6):2928–2942. doi: 10.1172/JCI99417
- Davenport BN, Jones HN, Wilson RL. Placental treatment with insulin-like growth factor 1 via nanoparticle differentially impacts vascular remodeling factors in guinea pig sub-placenta/decidua. Front Physiol. 2022;13:1055234. doi: 10.3389/fphys.2022.1055234
- Palmer KR, Tong S, Kaitu’u-Lino TJ. Placental-specific sFLT-1: role in pre-eclamptic pathophysiology and its translational possibilities for clinical prediction and diagnosis. Mol Hum Reprod. 2017;23(2):69–78. doi: 10.1093/molehr/gaw077
- Thadhani R, Hagmann H, Schaarschmidt W, et al. Removal of soluble fms-like tyrosine kinase-1 by dextran sulfate apheresis in preeclampsia. J Am Soc Nephrol. 2016;27(3):903–913. doi: 10.1681/ASN.2015020157
- Concord M. Comanche biopharma receives US FDA fast track designation for CBP-4888 for the treatment of sFlt-1 mediated pre-term preeclampsia. 2023. Accessed 2024 May 28. https://comanchebiopharma.com/comanche-biopharma-receives-us-fda-fast-track-designation-for-cbp-4888-for-the-treatment-of-sflt-1-mediated-pre-term-preeclampsia/
- Sezer SD, Küçük M, Döger FK, et al. VEGF, PIGF and HIF-1α in placentas of early- and late-onset pre-eclamptic patients. Gynecol Endocrinol. 2013;29(8):797–800. doi: 10.3109/09513590.2013.801437
- Umapathy A, Chamley LW, James JL. Reconciling the distinct roles of angiogenic/anti-angiogenic factors in the placenta and maternal circulation of normal and pathological pregnancies. Angiogenesis. 2020;23(2):105–117. doi: 10.1007/s10456-019-09694-w
- Li Z, Zhang Y, Ying Ma J, et al. Recombinant vascular endothelial growth factor 121 attenuates hypertension and improves kidney damage in a rat model of preeclampsia. Hypertension. 2007;50(4):686–692. doi: 10.1161/HYPERTENSIONAHA.107.092098
- Spencer R, Ambler G, Brodszki J, et al. EVERREST prospective study: a 6-year prospective study to define the clinical and biological characteristics of pregnancies affected by severe early onset fetal growth restriction. Bmc Pregnancy Childbirth. 2017;17(1):43. doi: 10.1186/s12884-017-1226-7
- Rissanen TT, Markkanen JE, Gruchala M, et al. VEGF-D is the strongest angiogenic and lymphangiogenic effector among VEGFs delivered into skeletal muscle via adenoviruses. Circ Res. 2003;92(10):1098–1106. doi: 10.1161/01.RES.0000073584.46059.E3
- Rossi C, Lees M, Mehta V, et al. Comparison of efficiency and function of vascular endothelial growth factor adenovirus vectors in endothelial cells for gene therapy of placental insufficiency. Hum Gene Ther. 2020;31(21–22):1190–1202. doi: 10.1089/hum.2020.006
- Wilson RL, Owens K, Sumser EK, et al. Nanoparticle mediated increased insulin-like growth factor 1 expression enhances human placenta syncytium function. Placenta. 2020;93:1–7. doi: 10.1016/j.placenta.2020.02.006
- King A, Ndifon C, Lui S, et al. Tumor-homing peptides as tools for targeted delivery of payloads to the placenta. Sci Adv. 2016;2(5):e1600349. doi: 10.1126/sciadv.1600349
- Lv Y, Lu C, Ji X, et al. Roles of microRnas in preeclampsia. J Cell Physiol. 2019;234(2):1052–1061. doi: 10.1002/jcp.27291
- Frazier S, McBride MW, Mulvana H, et al. From animal models to patients: the role of placental microRnas, miR-210, miR-126, and miR-148a/152 in preeclampsia. Clin Sci (Lond). 2020;134(8):1001–1025. doi: 10.1042/CS20200023
- Beards F, Jones LE, Charnock J, et al. Placental homing peptide-microRNA inhibitor conjugates for targeted enhancement of intrinsic placental growth signaling. Theranostics. 2017;7(11):2940–2955. doi: 10.7150/thno.18845
- Iacomino G. miRnas: The road from bench to bedside. Genes (Basel). 2023;14(2):314. doi: 10.3390/genes14020314
- Wilson RL, Jones HN. Targeting the dysfunctional placenta to improve pregnancy outcomes based on lessons learned in cancer. Clin Ther. 2021;43(2):246–264. doi: 10.1016/j.clinthera.2020.12.007
- Gathiram P, Moodley J. The role of the renin-angiotensin-aldosterone system in preeclampsia: a review. Curr Hypertens Rep. 2020;22(11):89. doi: 10.1007/s11906-020-01098-2
- Verdonk K, Visser W, Van Den Meiracker A, et al. The renin–angiotensin–aldosterone system in pre-eclampsia: the delicate balance between good and bad. Clin Sci (Lond). 2014;126(8):537–544. doi: 10.1042/CS20130455
- Olearczyk J, Gao S, Eybye M, et al. Targeting of hepatic angiotensinogen using chemically modified siRnas results in significant and sustained blood pressure lowering in a rat model of hypertension. Hypertens Res. 2014;37(5):405–412. doi: 10.1038/hr.2013.155
- Turco MY, Gardner L, Kay RG, et al. Trophoblast organoids as a model for maternal–fetal interactions during human placentation. Nature. 2018;564(7735):263–267. doi: 10.1038/s41586-018-0753-3
- Roberts RM, Green JA, Schulz LC. The evolution of the placenta. Reproduction. 2016;152(5):R179–89. doi: 10.1530/REP-16-0325
- Sakowicz A, Bralewska M, Kamola P, et al. Reliability of rodent and rabbit models in preeclampsia research. Int J Mol Sci. 2022;23(22):23(22. doi: 10.3390/ijms232214344
- Carter AM, Pijnenborg R. Evolution of invasive placentation with special reference to non-human primates. Best Pract Res Clin Obstet Gynaecol. 2011;25(3):249–257. doi: 10.1016/j.bpobgyn.2010.10.010
- Simon-Tillaux N, Lecarpentier E, Tsatsaris V, et al. Sildenafil for the treatment of preeclampsia, an update: should we still be enthusiastic? Nephrol Dial Transplant. 2019;34(11):1819–1826. doi: 10.1093/ndt/gfy328
- Bradbury A, Markusic D, Muhuri M, et al. Editorial: Immunogenicity and toxicity of AAV gene therapy. Front Immunol. 2023;14:1227231. doi: 10.3389/fimmu.2023.1227231
- Sood A, Salih S, Roh D, et al. Signalling of DNA damage and cytokines across cell barriers exposed to nanoparticles depends on barrier thickness. Nat Nanotech. 2011;6(12):824–833. doi: 10.1038/nnano.2011.188
- Hawkins SJ, Crompton LA, Sood A, et al. Nanoparticle-induced neuronal toxicity across placental barriers is mediated by autophagy and dependent on astrocytes. Nat Nanotech. 2018;13(5):427–433. doi: 10.1038/s41565-018-0085-3
- Li M, Wang H, Tian L, et al. COVID-19 vaccine development: milestones, lessons and prospects. Signal Transduct Target Ther. 2022;7(1):146. doi: 10.1038/s41392-022-00996-y
- Schneider H, Brownbill P, Albrecht C. Ex vivo dual perfusion of an isolated cotyledon of human placenta: History and future challenges. Placenta. 2021;107:8–12. doi: 10.1016/j.placenta.2021.02.017
- Valero L, Alhareth K, Gil S, et al. Assessment of dually labelled PEGylated liposomes transplacental passage and placental penetration using a combination of two ex-vivo human models: the dually perfused placenta and the suspended villous explants. Int J Pharm. 2017;532(2):729–737. doi: 10.1016/j.ijpharm.2017.07.076