ABSTRACT
Objective
To elucidate the underlying mechanism by which the proliferation and migration abilities of human umbilical cord mesenchymal stem cells (hUC-MSCs) determine their therapeutic efficacy in rheumatoid arthritis treatment.
Methods
The DBA/1J mice were utilized to establish a collagen-induced RA (CIA) mouse model and to validate the therapeutic efficacy of hUC-MSCs transfected with CD151 siRNA. RNA-seq, QT-PCR and western blotting were utilized to evaluate the mRNA and protein levels of the PI3K/AKT pathway, respectively.
Results
IFN-γ significantly enhanced the proliferation and migration abilities of hUC-MSCs, up-regulating the expression of CD151, a gene related to cell proliferation and migration. Effective inhibition of this effect was achieved through CD151 siRNA treatment. However, IFN-γ did not affect hUC-MSCs differentiation or changes in cell surface markers. Additionally, transplantation of CD151-interfered hUC-MSCs (siRNA-CD151-hUC-MSCs) resulted in decreased colonization in the toes of CIA mice and worse therapeutic effects compared to empty vector treatment (siRNA-NC-hUC-MSCs).
Conclusion
IFN-γ facilitates the proliferation and migration of hUC-MSCs through the CD151/PI3K/AKT pathway. The therapeutic efficacy of siRNA-CD151-hUC-MSCs was found to be inferior to that of siRNA-NC-hUC-MSCs.
Introduction
The transplantation of mesenchymal stem cells (MSCs) has demonstrated favorable outcomes in the treatment of rheumatoid arthritis (Citation1,Citation2). However, there remains a subset of patients who exhibit poor response to transplantation. Subsequent analysis revealed significant variations in the colonization of transplanted MSCs at the injured site, which may contribute to the observed differences in therapeutic efficacy for rheumatoid arthritis (Citation3,Citation4). Extensive research has established that sustained proliferation of MSCs is an essential prerequisite for successful application in tissue engineering (Citation5). It has been suggested that low rates of survival and proliferation could result in a limited number of functional tissue-forming MSCs, with less than 3% survival rate at 2 weeks post-transplantation (Citation6). Furthermore, proper localization is crucial for MSCs to exert their therapeutic effects (Citation7). The PI3K/AKT pathway has been widely utilized to induce MSC proliferation and migration in vitro through various stimulators such as prostaglandin E2 (PGE2), hepatocyte growth factor (HGF), fibroblast growth factor (FGF), tumor necrosis factor α (TNF-α), transforming growth factor β (TGF-β), epidermal growth factor (EGF) and insulin, underscoring the pivotal role played by pathway activation in regulating MSC proliferation (Citation8,Citation9). Several activators targeting the PI3K/AKT pathway have shown potential in enhancing MSC migration (Citation10), however, it should be noted that this effect can be counteracted by PI3K inhibitors like SDF-1 (Citation11). To fulfill their therapeutic potential, MSCs must maintain their inherent properties within the disease microenvironment after transplantation including three lineages differentiation and expression of specific cell surface markers which determine their regenerative translational applications (Citation12). Therefore, this study aims to investigate the impact of IFN-γ on MSCs’ proliferation, migration, and differentiation, along with their underlying mechanisms, and to explore the crucial role played by proliferation and migration characteristics in the effectiveness of MSCs therapy for RA.
Methods
Cell culture
MSCs were isolated from the human umbilical cord and provided by Chongqing Fumei Stem Cell Biotechnology Development Company. For the passage culture of hUC-MSCs, the medium was replaced with a serum substitute-containing medium (10828028, Gibco) in an incubator at 5% CO2 and 37°C. The third to sixth passages of hUC-MSCs were used for subsequent experiments. When the cells were stimulated with IFN-γ (300–02, Peprotech), the MSCs medium was replaced with α-MEM (C3060–0500, Viva Cell) containing 5% fetal bovine serum (10099141C, Gibco).
siRNA targeting human CD151 (TCACAGGACTGGCGAGACATT) and control siRNA targeting p21 protein of human immunodeficiency virus were transfected into hUC-MSCs using the Lipofectamine 2000 transfection reagent (11668500, Thermo Fisher Scientific, Invitrogen) and cultured for 48 hours before subsequent analysis.
Induction, evaluation, and cell therapy of collagen-induced arthritis (CIA) and bioluminescence imaging experiments
DBA/1J mice (7–8 weeks old, Vital River Laboratory Animal Technology Co., Beijing) were subcutaneously administered an equal volume of an emulsified mixture containing type II chicken collagen (CII; Chondrex, MD Biosciences) and Freund’s complete adjuvant (CFA; Cartilage, MD Bioscience). On day 21, the mice received a subcutaneous injection of an equal volume of CII with an emulsified mixture of incomplete Freund’s adjuvant (IFA, Chondrex, MD Biosciences), containing 100 µl and 100ug of chicken collagen respectively. The severity of arthritis was also evaluated. On day 27, the CIA mice were subcutaneously injected with si-NC or si-CD151 transfected hUC-MSCs, respectively. Toe swelling was assessed every two days and the mice were sacrificed on day 50. The tactile hypersensitivity of CIA mice was assessed by acclimating them to a metal mesh floor for 15 minutes, followed by measuring the paw withdrawal threshold on the middle surface of their hind foot using the Von Frey force application. The pressure required to elicit a paw withdrawal response indicated the maximum injury threshold. Three stimuli were applied and the average paw withdrawal threshold was recorded.
The positive clones of hUC-MSCs transfected with fluorescently labeled siRNA were identified by luciferase activity to screen for cells with stable high expression. CIA mouse models were constructed as previously described and treated with stem cells via the tail vein. After 24 hours, the anesthetized mice were placed in a small animal in vivo imaging system (IVIS Series Lumina LT Series III) for imaging analysis.
Immunofluorescence staining
Cells were fixed with 4% paraformaldehyde for 10 minutes, followed by blocking in immunostaining buffer containing 2% bovine serum albumin at 37°C for 30 minutes. Subsequently, the samples were incubated overnight at 4°C with the indicated primary antibody Ki67 Rabbit mAb (9129S, Cell Signaling Technology) or PH3 (ab80612, Abcam) (diluted to a ratio of 1:100) and then with the corresponding secondary antibody (coupled to Alexa Fluor 488) for 1 hour at 37°C. The slides were sealed with an anti-fluorescence quenching sealing agent within 2 minutes of DAPI staining. Immunofluorescence images were acquired using a laser confocal microscope (Olympus, Tokyo) and analyzed using the Olympus Fluoview FV2000 acquisition software.
Clone formation assay
A single-cell suspension was prepared by digestion and centrifugation of the monolayer cells in the logarithmic growth phase, followed by resuspension of the cell precipitate, cell counting, and double dilution to a concentration of 1 × 103 cells/ml. Subsequently, the cells were seeded into six-well plates at gradient densities of 50, 100, and 200 cells per well (determined based on cell growth), supplemented with a 3 mL of culture medium. The plates were then placed in an incubator for cultivation for a period of 2–3 weeks until adherence to the plate surface was observed. On the following day, IFN-γ treatment was administered.
RTCA assay
Cells were digested using a trypsin solution and subsequently centrifuged at 1000 rpm/min for 5 minutes. After removing the supernatant, fresh medium was added and the cell concentration was adjusted to 2 × 105 cells/ml. Subsequently, each well of an E-Plate 16 (C515738, ACEA) or CIM-Plate 16 (388294, ACEA) was supplemented with 50 µl of medium and placed on the RTCA DP test bench to establish baseline measurements. Once the baseline measurements were completed, the E-plate was removed and a cell suspension (1000, 1500, 2000, or 2500 cells per well) in a volume of 100 µl was added for incubation at room temperature for 30 minutes. Finally, the E-plate or CIM-Plate 16 containing the cell suspension was returned to the test bench for the real-time dynamic detection of cell proliferation or migration.
Cell wound scratch assay
Cells were seeded at a density of 5 × 105 cells per well, with the option of adjusting the number based on the cell state while ensuring even distribution using either a cross or figure-of-eight method. A vertical horizontal line of 200ul was dispensed to ensure consistent strength. Subsequently, the cells were washed with PBS 2–3 times and cultured in a serum-free medium before being treated with IFN-γ. Incubations were incubated in a CO2 incubator at 37°C, and photographs were taken at 0 and 24 hours.
Transwell assay
Cells were removed from the medium and subjected to 24-hour serum deprivation by adding serum-free medium and were resuspended in serum-free medium and introduced into the chamber, whereas media containing IFN-γ were added externally. The 24-well plates were then incubated at 37°C, 5% CO2, and 90% humidity for a duration of 24 to 48 hours. Subsequently, the cells within the chamber were gently wiped using a PBS-soaked cotton swab before being fixed with a paraformaldehyde fixative (4%) for a period of 10 minutes. 600 μL crystal violet staining solution (C0121, Beyotime) was added to each well for an interval of 10 minutes. This process was followed by three rinses with PBS solution, the wells were observed under a microscope and photographed.
Surface marker screening
Cell identification of cells was confirmed by performing flow cytometry according to the minimum criteria set by the International Society for Cell and Gene Therapy (ISCT). The 3rd passage of hUC-MSCs was washed in FACS buffer containing 2% heat-inactivated FBS and 0.04% sodium azido-D-PBS, passed through a 40 μm cell filter, and then blocked with BD Human Fc BlockTM for 15 minutes. Subsequently, R-phycoerythrin (PE) conjugated antibodies against anti-CD29 (557332, BD), anti-CD34 (555822, BD), anti-CD44 (555479, BD), anti-CD45(555483, BD), anti-CD73(550257, BD), anti-CD90(555596, BD), and anti-CD105(560839, BD), and with their corresponding isotype controls were incubated at 4°C for 20 minutes. After washing twice in FACS buffer, the samples were resuspended in 200 μL of FACS buffer for analysis. Finally, the samples were analyzed using the FlowJo software (version 10.8.1).
Chondrogenic differentiation
Cells in the logarithmic growth phase were digested and counted and then resuspended in a chondrogenic induction medium (A1007101, Gibco). After centrifugation, the cell density was adjusted to 1.0 × 107 cells/mL. 20 μl cell suspension was pipetted into the center of a 24-well plate and incubated at 37°C in a 5% CO2 environment for 2 to 3 hours to allow cell adhesion. The culture medium was changed every 2 to 3 days throughout the induction period of 21–28 days. The bottom surface of each culture dish was covered with 4% formaldehyde. After fixation at room temperature for 30–60 minutes, the cells were washed twice with PBS. Alcian blue staining solution was added and the cells were allowed to stain for 30 minutes in the dark. The stained cells were subsequently washed twice with PBS. The chondrogenic staining effect was observed under a microscope, and image acquisition and induction evaluation, were performed accordingly.
Adipogenic differentiation
Cells in the logarithmic growth phase were seeded into the culture vessel at a cell density of 2.0 × 104 cells/cm (Citation2) and reached a confluence degree of 90–100%. After 3 days of culture, the medium was replaced with a maintenance medium for adipogenic induction and differentiation. After 1 day of culture, the cells were further replaced with an induction medium (A1007001, Gibco) for adipogenic induction and differentiation, and then cultured for an additional 3 days. This frequency of induction was repeated for a period ranging from 14 to 21 days. The cells were washed once with 1×PBS and fixed with a solution containing 4% formaldehyde at room temperature for 30–60 minutes, followed by two additional washes with 1×PBS. Normal saline and Oil red O stock solution were used to prepare the working solution (Oil Red O stock solution: normal saline = 3:2), which was then added to each well designated for induction staining for 30 minutes. Adipogenic staining was observed under a microscope, followed by image acquisition and evaluation of induction.
Osteogenic differentiation
Cells in the logarithmic growth phase were seeded at a density of 2.0 × 104 cells/cm (Citation2) in a culture dish and reached a confluence degree of 60–70%. Subsequently, osteogenic differentiation medium (A1007201, Gibco) was applied to the cells for a period of 14–21 days, with medium changes every 2–3 days. The fixed cells were treated with 4% formaldehyde at room temperature for 30–60 minutes, followed by two washes with 1×PBS solution. Alizarin red staining solution was then applied to stain the cells for approximately 3–5 minutes before washing twice again with PBS buffer. Finally, microscopic observation allowed the evaluation and collection of images depicting the osteogenic staining effect.
RNA-sequencing and data analysis
TRIzol™Plus RNA (12183555) was utilized to extract total RNA from hUC-MSCs. Subsequently, the PrimeScript first strand cDNA Synthesis kit (Takara Bio, San Jose, CA) was employed to synthesize cDNA starting from 1 μg of total RNA. For real-time PCR analysis, the iTaq™ Universal SYBR® Green Supermix (1725124, Bio-Rad) and optimized amplification conditions for the Bio-Rad CFX96 Touch Real-Time PCR qPCR system were used. Each gene was analyzed in triplicate using the comparative CT method with GAPDH serving as an internal normalization control. The RNA was extracted from interferon-treated cells using a TRIzolTM reagent. Differentially expressed transcripts between groups were analyzed with default settings and exon fragments per kilobase per million reads. Gene expression heatmaps, as well as Gene Ontology (GO) and Kyoto Encyclopedia of Genes and Genomes (KEGG) pathway analyses.
Western Blot assay
The cells were harvested, washed with PBS, and lysed using cell lysate (P0013, Beyotime) for 30 min on ice. The lysates were then centrifuged at 12 000 g for 15 min, and the resulting supernatant was mixed with 5× upper sample buffer (P0015L; Beyotime) and boiled at 100°C for 10 min. Subsequently, the proteins were separated by sodium dodecyl sulfate-polyacrylamide gel electrophoresis and transferred onto nitrocellulose membranes. Following this step, the membranes were incubated overnight with primary antibodies and washed thrice with Tris-buffered saline Tween (TBST). The membranes were then incubated with the corresponding secondary antibodies for 1 h at room temperature while shaking gently. The membranes were then washed three times with TBST. Protein detection was performed using a Bio-Rad imaging system (Bio-Rad, Hercules, CA, USA), and protein abundance was quantified using the ImageJ software (version 1.48; Wayne Rasband, Maryland). The primary antibodies used in the Western Blot assay were β-actin(4967, Cell Signaling Technology), CD151(96282, Cell Signaling Technology), PTN(ab133517, Abcam), FGF-7(90162, Cell Signaling Technology), CDC42(2462, Cell Signaling Technology), MYC(ab32072, Abcam), Wnt2(ab109222, Abcam), FGF-2(ab208687, Abcam), AKT(9272, Cell Signaling Technology), p-AKT(4060, Cell Signaling Technology), PI3K(4292, Cell Signaling Technology), p-PI3K(17366, Cell Signaling Technology), and β-catenin(9562, Cell Signaling Technology).
Statistical analysis
The data are presented as mean ± standard deviation (SD), and statistical analyses were conducted using SPSS software (version 19.0). One-way analysis of variance (ANOVA) was used to compare means between two groups, while Tukey’s multiple comparison test was used for comparisons among three or more groups. Statistical significance was set at p < .05.
Results
IFN-γ enhances the proliferation of hUC-MSCs
To investigate the impact of IFN-γ on the proliferation of hUC-MSCs, third passage cells were cultured and treated with 0, 5, 10, and 20 ng/ml IFN-γ for 24 hours. Proliferation ability was evaluated using immunofluorescence staining indicators Ki67 and PH3. The results demonstrated a significant enhancement in the proliferative ability of MSCs with increasing concentrations of IFN-γ. Specifically, at concentrations of 5, 10, and 20 ng/ml, there was an approximate increase in Ki67-positive cells by approximately 18%, 57%, and 135%, respectively, whereas PH3-positive cells increased by approximately 40%,100%, and 220%, respectively, compared to untreated cells (). Assessment of colony-forming ability serves as a robust approach for evaluating population-dependent characteristics and cellular proliferation capacity. It should be noted that not all adherent cells possess the capability to proliferate and form clones; only those exhibiting both adhesion and proliferation abilities can generate clones. The results demonstrated that the presence of 20 ng/ml IFN-γ led to increased formation of colony spheres (), which was further supported by the RTCA results, which unequivocally confirmed the significant promotion of MSCs proliferation by IFN-γ ().
Figure 1. IFN-γ of different concentrations enhances the proliferation ability of hUC-MSCs.
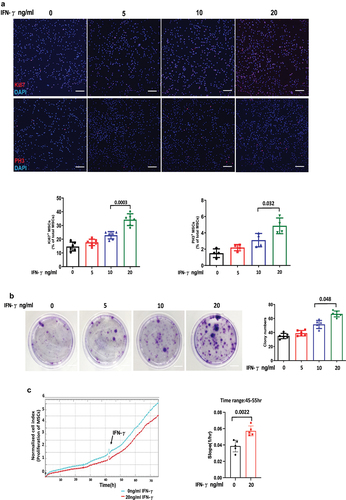
IFN-γ enhances the migration of hUC-MSCs
To further investigate the impact of IFN-γ on MSC migration, cell scratch and transwell assays were performed to assess the migratory capacity of MSCs. As depicted in , the migratory potential of hUC-MSCs exhibited a similar enhancement pattern as their proliferation at three different concentrations of hUC-MSCs, particularly following treatment with 20 ng/ml IFN-γ, which resulted in an increase of approximately 71% and 300% compared to the control group, respectively. Additionally, RTCA analysis demonstrated a more pronounced migratory effect of hUC-MSCs in response to IFN-γ ().
Figure 2. IFN-γ of different concentrations increases the migration ability of hUC-MSCs.
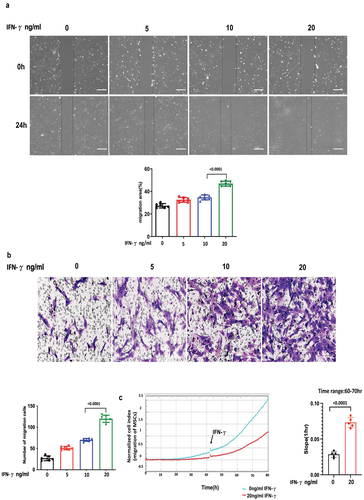
IFN-γ has a minor effect on the stemness properties of hUC-MSCs as identified by induction of three-lineage differentiation and surface markers
As shown in , the result illustrates that the trilineage differentiation capacity of hUC-MSCs remained unaffected by IFN-γ at concentrations ranging from 0, 10, and 20 ng/ml ng/ml, as evidenced by adipogenic, osteogenic, and chondrogenic assays, along with associated staining. Expression of the surface markers for hUC-MSC identification was assessed by flow cytometry (), and hUC-MSCs cultured in all conditions containing IFN-γ (0, 10, and 20 ng/ml) expressed the standard positive surface phenotype (≥90% expression of CD29, CD44, CD90, CD105, and CD73), and lacked expression (≤2%) of the negative markers CD34 and CD45. There were marked variations in the expression of CD73, a cell surface enzyme that can convert ATP with immune-activating function to adenosine with immunosuppressive effect to form an immunosuppressive microenvironment, except for CD73, which had no significant effect on the expression of other surface markers in hUC-MSCs.
Figure 3. IFN-γ of different concentrations has minner effect on the stemness properties of hUC-MSCs as identified by induction of three-lineage differentiation and surface markers.
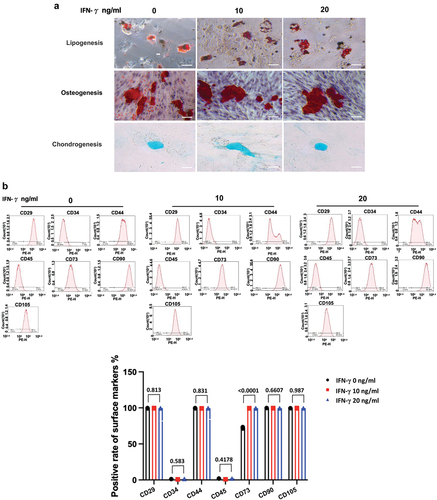
Loss of CD151 attenuates the effect of IFN-γ on proliferation and migration of hUC-MSCs
To explore how IFN-γ affects the proliferation and migration of hUC-MSCs, we performed transcriptome analysis via RNA-seq using total RNAs from passage 3 hUC-MSCs for IFN-γ treatment. Box plots of RNA-seq read counts in each sample demonstrated comparable overall variability, ensuring that the observed differences were not attributable to skewed data. GO and KEGG analyses showed that IFN-γ induced the transcriptional reprogramming of networks related to cell proliferation, activated genes involved in the mitotic cell cycle process, chromosome organization, mitotic spindle organization, nuclear division, and numerous cell migration-related genes, including cell-substrate adhesion, cell-matrix adhesion, substrate adhesion-dependent cell spreading, and calcium-independent cell-cell adhesion (). Among the 793 upregulated genes, 45 enriched cell proliferation/migration-related GO terms were screened (), and some representative genes and proteins were further validated by qRT-PCR () and immunoblotting (), respectively. Gene set enrichment analyses further indicated that IFN-γ-induced hUC-MSCs led to a dynamic bias toward the positive regulation of cell cycle/migration activation (). These results suggested that IFN-γ regulates hUC-MSC cycling by triggering transcriptional reprogramming of a large number of genes that drive hUC-MSC cell cycle/migration activation.
Figure 4. Loss of CD151 attenuates the effect of IFN-γ on the proliferation and migration of hUC-MSCs.
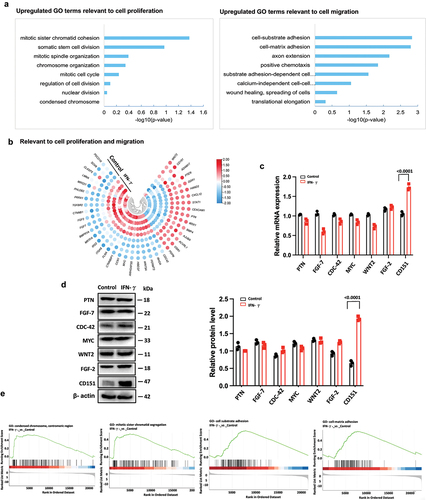
CD151 increases PI3K and AKT phosphorylation on promoters of proliferation/migration in hUC-MSCs
Numerous studies have demonstrated the crucial roles of the WNT and PI3K/AKT signaling pathways in cellular proliferation and migration (Citation13–17). Therefore, we conducted an initial screening to assess the expression of key molecules involved in these two pathways. Western blot analysis revealed that IFN-γ significantly upregulated the expression of p-AKT and p-PI3K, but did not affect the levels of β-catenin, AKT, and PI3K (). These findings suggest that IFN-γ may modulate hUC-MSC proliferation/migration through the PI3K/AKT signaling pathway. To further investigate the relationship between CD151 and this pathway in terms of proliferation/migration, we employed RNA interference to knock down CD151 expression in hUC-MSCs. Our results indicate that CD151 knockdown markedly attenuated both AKT and p-PI3K activation induced by IFN-γ (), consequently diminishing the enhancement of hUC-MSC proliferation () and migration () mediated by hUC-MSC treatment. Henceforth, it can be concluded that IFN-γ promotes hUC-MSCs proliferation/migration via the CD151/PI3K/AKT signaling pathway.
Figure 5. CD151 increases PI3K and AKT phosphorylation on promoters of proliferation/migration in hUC-MSCs.
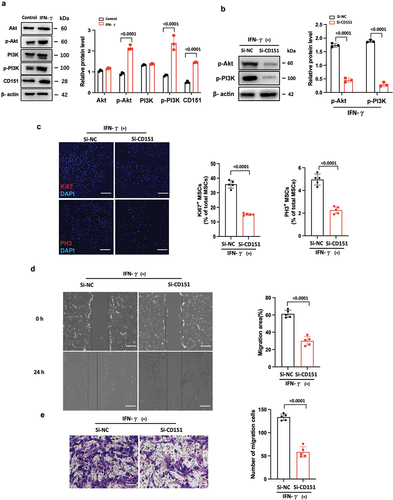
Knockdown of CD151 attenuates the local recruitment capacity and therapeutic effect of MSCs on CIA mice
To validate the therapeutic efficacy of CD151 in CIA mice through IFN-γ-enhanced hUC-MSC proliferation and migration, we initially established a CIA mouse model, as previously described. Extensive research has demonstrated the presence of IFN-γ in the microenvironment of CIA mice (Citation5). Consequently, hUC-MSCs modified with si-NC or si-CD151 were intravenously injected into the tail vein of CIA mice without inducing IFN-γ. The induction and treatment processes are shown in . The results revealed a significant reduction in the number of si-CD151-hUC-MSCs in the toe compared to the si-NC-hUC-MSCs group (). Additionally, si-CD151-hUC-MSCs exhibited limited therapeutic effects characterized by an increased joint swelling score (), reduced thermal pain threshold (), heightened mechanical stimulation sensitivity (), and exacerbated bone destruction ().
Figure 6. Knockdown of CD151 attenuates the local recruitment capacity and therapeutic effect of MSCs on CIA mice.
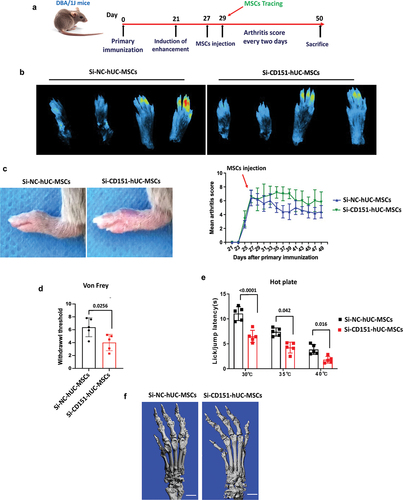
Discussion
hUC-MSCs have been extensively utilized in the transplantation of various diseases due to their easy accessibility, low immunogenicity, and potent immunomodulatory function, resulting in significant advancements (Citation18). IFN-γ, a crucial inflammatory factor, is secreted by activated T cells and NK cells. It is employed in clinical practice to combat viruses and tumors and regulate immune responses (Citation19) Numerous studies have demonstrated that IFN-γ also plays a crucial role in augmenting the immunosuppressive function of MSCs, thereby significantly enhancing the therapeutic efficacy of stem cell transplantation (Citation20–23). However, the proliferation, migration, and differentiation capabilities of MSCs also play crucial roles in determining the efficacy of stem cell therapies (Citation24). Therefore, it is imperative to investigate the impact of IFN-γ on these aspects, while concurrently focusing on modulating the immunomodulatory capacity of MSCs. In this study, we have confirmed through immunofluorescence staining assays, RTCA, cell cloning assay, cell scratch assay, and transwell assay that IFN-γ can substantially enhance both proliferation and migration capabilities of hUC-MSCs. These findings have significant implications for the advancement of high-quality and large-scale implementation of stem cell therapy.
CD151 is an oncogene belonging to the transmembrane 4 superfamily (TM4SF), which plays a crucial role in regulating cell adhesion, migration, and proliferation through specific binding to integrins and is involved in various pathophysiological processes such as cell adhesion, migration, hemidesmosome formation, and integrin transport via vesicles (Citation25–27). Moreover, numerous in vitro experiments have demonstrated that CD151 significantly promotes proliferation, migration, and reticular vessel formation of vascular endothelial cells, thereby playing a pivotal role in angiogenesis and remodeling (Citation28–31). In this study, we conducted RNA-seq QT-PCR and WB analysis of hUC-MSCs treated with IFN-γ for 24 hours and observed high expression levels of CD151 among genes associated with proliferation and migration. Consequently, we hypothesized that CD151 may also contribute to the promotion of MSC proliferation and migration. To validate this hypothesis, we constructed an interference RNA targeting CD151, which resulted in a significant decrease in cell proliferation and migration ability post-IFN-γ. Meanwhile, transplantation of MSCs transfected with si-CD151-hUC-MSCs to CIA mice significantly attenuated the therapeutic effect of hUC-MSCs compared to the si-NC-hUC-MSCs group.
Stem cell stemness plays a pivotal role in determining the therapeutic efficacy (Citation32–34). Hence, this study also aimed to assess the stemness properties of hUC-MSCs, including their trilineage differentiation potential and cell surface markers. The findings revealed that IFN-γ treatment had no impact on the differentiation capacity. CD73 expression was upregulated in a concentration-dependent manner, whereas the other surface markers exhibited no significant alterations. In addition to serving as a surface marker for MSCs, CD73 also functions as a cell surface enzyme that converts ATP to adenosine, thereby exerting an immunosuppressive effect and creating an immunosuppressive microenvironment (Citation35–37). This coincided with the enhanced immunosuppressive ability of hUC-MSCs following IFN-γ stimulation. Additionally, the surface markers CD29, CD44, CD90, and CD105 are commonly expressed in hUC-MSCs and play pivotal roles in diverse biological processes. These markers have been extensively employed for diagnostic and therapeutic purposes. However, our findings indicate that the expression of these markers is not affected by IFN-γ. This observation can be attributed to the fact that IFN-γ primarily exerts its effects through the JAK-STAT signaling pathway, which does not directly regulate the expression of CD29, CD44, CD90, and CD105. Moreover, it is crucial to further investigate whether other mechanisms are involved in modulating the expression of these markers in hUC-MSCs. In conclusion, the present study demonstrated that IFN-γ could enhance the proliferation and migration of hUC-MSCs through CD151/PI3K/AKT signaling. The therapeutic efficacy of hUC-MSCs on CIA mice was significantly diminished following transfection with small interfering RNA CD151. These findings provide additional rationale for enhancing the efficacy of stem-cell therapy.
Disclosure statement
No potential conflict of interest was reported by the author(s).
Correction Statement
This article has been corrected with minor changes. These changes do not impact the academic content of the article.
Additional information
Funding
References
- Gao F, Chiu SM, Motan DA, Zhang Z, Chen L, Ji HL, Tse HF, Fu QL, Lian Q. Mesenchymal stem cells and immunomodulation: current status and future prospects. Cell Death Disease. 2016;7(1):e206. doi:10.1038/cddis.2015.327.
- Shin MJ, Park JY, Park JY, Lim SH, Lim H, Choi JK, Park CK, Kang YJ, Khang D. Inflammation-targeting mesenchymal stem cells combined with photothermal treatment attenuate severe joint inflammation. Adv Mater. 2023;14(12):1–12. doi:10.1002/adma.202304333.
- Giuliana MB, Marie M, Christian J, Noël D. Therapeutic potential in rheumatic diseases of extracellular vesicles derived from mesenchymal stromal cells. Nat Rev Rheumatol. 2023;19(11):682–94. doi:10.1038/s41584-023-01010-7.
- Djouad F, Bouffi C, Ghannam S, Noël D, Jorgensen C. Mesenchymal stem cells: innovative therapeutic tools for rheumatic diseases. Nat Rev Rheumatol. 2009;5(7):392–99. doi:10.1038/nrrheum.2009.104.
- Lian Q, Zhang Y, Zhang J, Zhang HK, Wu X, Zhang Y, Lam FF, Kang S, Xia JC, Lai WH, et al. Functional mesenchymal stem cells derived from human induced pluripotent stem cells attenuate limb ischemia in mice. Circulation. 2010;121(9):1113–23. doi:10.1161/CIRCULATIONAHA.109.898312.
- Nguyen PK, Neofytou E, Rhee JW, Wu JC. Potential strategies to address the major clinical barriers facing stem cell regenerative therapy for cardiovascular disease: a review. JAMA Cardiol. 2016;1(8):953–62. doi:10.1001/jamacardio.2016.2750.
- Qin H, Zhao A. Mesenchymal stem cell therapy for acute respiratory distress syndrome: from basic to clinics. Protein Cell. 2020;11(10):707–22. doi:10.1007/s13238-020-00738-2.
- Samakova A, Gazova A, Sabova N, Valaskova S, Jurikova M, Kyselovic J. The PI3k/Akt pathway is associated with angiogenesis, oxidative stress and survival of mesenchymal stem cells in pathophysiologic condition in ischemia. Physiol Res. 2019;68(Suppl 2):S131–38. doi:10.33549/physiolres.934345.
- Chen J, Crawford R, Chen C, Xiao Y. The key regulatory roles of the PI3K/Akt signaling pathway in the functionalities of mesenchymal stem cells and applications in tissue regeneration. Tissue Eng Part B Rev. 2013;19(6):516–28. doi:10.1089/ten.TEB.2012.0672.
- Fathi E, Valipour B, Vietor L, Farahzadi R. An overview of the myocardial regeneration potential of cardiac c-Kit+ progenitor cells via PI3K and MAPK signaling pathways. Future Cardiol. 2020;16(3):199–209. doi:10.2217/fca-2018-0049.
- Lapidot T, Dar A, Kollet O. How do stem cells find their way home? Blood. 2005;106(6):1901–10. doi:10.1182/blood-2005-04-1417.
- Walewska A, Janucik A, Tynecka M, Moniuszko M, Eljaszewicz A. Mesenchymal stem cells under epigenetic control - the role of epigenetic machinery in fate decision and functional properties. Cell Death Disease. 2023;14(11):720. doi:10.1038/s41419-023-06239-4.
- Li S, Motiño O, Lambertucci F, Martins I, Sun L, Kroemer G. Protein regulator of cytokinesis 1: a potential oncogenic driver. Mol Cancer. 2023;22(1):128. doi:10.1186/s12943-023-01802-1.
- Jiang T, Wang H, Liu L, Song H, Zhang Y, Wang J, Liu L, Xu T, Fan R, Xu Y. CircIL4R activates the PI3K/AKT signaling pathway via the miR-761/TRIM29/PHLPP1 axis and promotes proliferation and metastasis in colorectal cancer. Mol Cancer. 2021;20(1):167. doi:10.1186/s12943-021-01474-9.
- Verret B, Cortes J, Bachelot T, Andre F, Arnedos M. Efficacy of PI3K inhibitors in advanced breast cancer. Ann Oncol. 2019;30(Supp 10):x12–20. doi:10.1093/annonc/mdz381.
- Mill C, George SJ. Wnt signaling in smooth muscle cells and its role in cardiovascular disorders. Cardiovasc Res. 2012;95(2):233–40. doi:10.1093/cvr/cvs141.
- Miller JR. The wnts. Genome Biol. 2002;3(1):1–15. doi:10.1186/gb-2001-3-1-reviews3001.
- Ding Y, Liang X, Zhang Y, Yi L, Shum H, Chen Q, Chan BP, Fan H, Liu Z, Tergaonkar V. Rap1 deficiency-provoked paracrine dysfunction impairs immunosuppressive potency of mesenchymal stem cells in allograft rejection of heart transplantation. Cell Death Disease. 2018;9(3):386. doi:10.1038/s41419-018-0414-3.
- Gálvez N, Bohmwald K, Pacheco GA, Andrade CA, Carreño LJ, Kalergis AM. Type I natural killer T cells as key regulators of the immune response to infectious diseases. Clin Microbiol Rev. 2021;34(2):1–8. doi:10.1128/CMR.00232-20.
- Lucero L, Marta E. TNF-α and IFN-γ participate in improving the immunoregulatory capacity of mesenchymal stem/stromal cells: importance of cell-cell contact and extracellular vesicles. Int J Mol Sci. 2021;22(17):1–17. doi:10.3390/ijms22179531.
- He X, Yang Y, Yao M, Yao M, Yang L, Ao L, Hu X, Li Z, Wu X, Tan Y, et al. Combination of human umbilical cord mesenchymal stem (stromal) cell transplantation with IFN-γ treatment synergistically improves the clinical outcomes of patients with rheumatoid arthritis. Ann Rheum Dis. 2020;79(10):1298–304. doi:10.1136/annrheumdis-2020-217798.
- Yao M, Chen Z, He X, Long J, Xia X, Li Z, Yang Y, Ao L, Xing W, Lian Q. Cross talk between glucose metabolism and immunosuppression in IFN-γ-primed mesenchymal stem cells. Life Sci Alliance. 2022;5(12):1–13. doi:10.26508/lsa.202201493.
- Liang X, Ding Y, Zhang Y, Tse HF, Lian Q. Paracrine mechanisms of mesenchymal stem cell-based therapy: current status and perspectives. Cell Transplant. 2014;23(9):1045–59. doi:10.3727/096368913X667709.
- Gao Q, Jia F, Li X, Kong Y, Tian Z, Bi L, Li L. Biophysical cues to improve the immunomodulatory capacity of mesenchymal stem cells: the progress and mechanisms. Biomed Pharmacother. 2023;162(9):1045–59. doi:10.1016/j.biopha.2023.114655.
- Takeda Y, Li Q, Kazarov AR, Epardaud M, Elpek K, Turley S, Hemler ME. Diminished metastasis in tetraspanin CD151-knockout mice. Blood. 2011;118(2):464–72. doi:10.1182/blood-2010-08-302240.
- Margot Z. Tetraspanins: push and pull in suppressing and promoting metastasis. Nat Rev Cancer. 2009;9(1):40–55. doi:10.1038/nrc2543.
- Takeda Y, Kazarov AR, Butterfield CE, Kaipainen A, Hemler ME. Deletion of tetraspanin Cd151 results in decreased pathologic angiogenesis in vivo and in vitro. Blood. 2007;109(4):1524–32. doi:10.1182/blood-2006-08-041970.
- Malla R, Marni R, Chakraborty A. Exploring the role of CD151 in the tumor immune microenvironment: Therapeutic and clinical perspectives. Biochim Biophys Acta Rev Cancer. 2023;1878(3):188898. doi:10.1016/j.bbcan.2023.188898.
- Erfani S, Hua H, Pan Y, Zhou BP, Yang XH. The context-dependent impact of integrin-associated CD151 and other tetraspanins on cancer development and progression: a class of versatile mediators of cellular function and signaling, tumorigenesis and metastasis. Cancers (Basel). 2021;13(9):9–15. doi:10.3390/cancers13092005.
- Peng D, Zuo H, Liu Z, Qin J, Li P, Wang D, Zeng H, Zhang X. The tetraspanin CD151‑ARSA mutant inhibits angiogenesis via the YRSL sequence. Mol Med Rep. 2023;28(4):1–8. doi:10.3892/mmr.2023.13069.
- Gao C, Jia W, Xu W, Wu Q, Wu J. Downregulation of CD151 restricts VCAM-1 mediated leukocyte infiltration to reduce neurobiological injuries after experimental stroke. J Neuroinflammation. 2021;18(1):118. doi:10.1186/s12974-021-02171-6.
- Kuhn NZ, Tuan RS. Regulation of stemness and stem cell niche of mesenchymal stem cells: implications in tumorigenesis and metastasis. J Cell Physiol. 2010;222(2):268–77. doi:10.1002/jcp.21940.
- Sbrana FV, Cortini M, Avnet S, Perut F, Columbaro M, Milito AD, Baldini N. The role of autophagy in the maintenance of stemness and differentiation of mesenchymal stem cells. Stem Cell Rev Rep. 2016;12(8):621–33. doi:10.1007/s12015-016-9690-4.
- Ma L, He X, Wu Q. The molecular regulatory mechanism in multipotency and differentiation of Wharton’s jelly stem cells. Int J Mol Sci. 2023;24(16):1–14. doi:10.3390/ijms241612909.
- Xia C, Yin S, To KK, Fu L. CD39/CD73/A2AR pathway and cancer immunotherapy. Mol Cancer. 2023;22(1):44. doi:10.1186/s12943-023-01733-x.
- Young A, Ngiow SF, Barkauskas DS, Sult E, Hay C, Blake SJ, Huang Q, Liu J, Takeda K, Teng MWL, et al. Co-inhibition of CD73 and A2AR adenosine signaling improves anti-tumor immune responses. Cancer Cell. 2016;30(3):391–403. doi:10.1016/j.ccell.2016.06.025.
- Tang T, Huang X, Lu M, Zhang G, Han X, Liang T. Transcriptional control of pancreatic cancer immunosuppression by metabolic enzyme CD73 in a tumor-autonomous and -autocrine manner. Cancer Res. 2023;14(1):3364. doi:10.1038/s41467-023-38578-3.