ABSTRACT
Pharmaceuticals are detected at trace levels in waters. Their adverse effects on aquatic ecosystems and human health demand novel pharmaceutical removal technologies for treating wastewater effluents. Iron (Fe) or manganese (Mn) may play important roles in these new technologies since these metals are abundantly available at low costs and are known to contribute to organic conversions via physico-chemical, chemical, and biologically related processes. Few reviews describe and discuss Fe- or Mn-based technologies for the purpose to remove pharmaceuticals from water. Therefore, we review the current literature sorted into the three removal mechanisms, that is., through physico-chemical, chemical, and biological processes. The principals, performance, and influential parameters of these three types of technologies are described. Current and potential applications of these technologies are critically evaluated in order to identify advantages and challenges. In addition, the Fe- or Mn-based technologies which are currently not used but promising to further develop to remove pharmaceuticals cost efficiently are proposed.
1. Introduction
Removing pollutants from water is crucial to human as well as ecosystem health. Micropollutants are a growingly popular subject due to their low concentration and potential hazard. Among the micropollutants, pharmaceuticals are well studied and can be used as a representative case for a variety of micropollutants. Pharmaceuticals, commonly known as medicines or drugs, are a vast array of chemical compounds used for medical diagnosis, cure, treatment, or prevention of disease (Rivera-Utrilla et al., Citation2013). Improvements in analytical technologies have resulted in detection techniques to quantify pharmaceuticals at trace level (ng/L - µg/L) in wastewater, groundwater and surface water, and drinking water (Dougherty et al., Citation2010; Chieng et al., Citation2011; Huerta-Fontela et al., Citation2011; Martin et al., Citation2012). Pharmaceuticals in water can threaten the quality of drinking water resources, can lead to spread of antibiotic resistance (Pruden et al., Citation2006; Yan et al., Citation2014), and can be toxic to aquatic organisms (Kümmerer, Citation2004; Sanderson et al., Citation2004). The presence and fate of these compounds have become a growing worldwide concern for politicians and the general public (Jelić et al., Citation2012; WHO, Citation2012). For example, U.S. Environmental Protection Agency and European Union have added pharmaceuticals to their watch list for water quality (EU, Citation2013; USEPA, Citation2015). Due to adverse effects of pharmaceuticals in water on the quality of drinking water and food supply to humans, and on the functioning of ecosystems, regulators start to call for cost-efficient removal technologies to reduce emissions of such compounds.
Pharmaceuticals from human consumption, excretion, and disposal mainly enter the environment via wastewater (Ikehata et al., Citation2006). If not removed, they will pass through wastewater treatment trains and enter water bodies directly (Tixier et al., Citation2003; Monteiro and Boxall, Citation2010). Advanced technologies are employed to remove these pharmaceuticals from water (Klavarioti et al., Citation2009; Bae et al., Citation2012; Segura et al., Citation2013; Zhan et al., Citation2013; Gao et al., Citation2013; He et al., Citation2016). Iron (Fe) and manganese (Mn) play important roles in many water treatment technologies, including physico-chemical removal (e.g., polyferric coagulation) (Xing and Sun, Citation2009), chemical removal (e.g., Fenton) (Li et al., Citation2012; Mackulak et al., Citation2015), and biologically related removal (e.g., biogenic Mn oxides oxidation) (Forrez et al., Citation2010; Furgal et al., Citation2015). Fe- or Mn-based technologies for treating organic chemicals and heavy metals in general are reviewed in the literature (Li et al., Citation2006; Cundy et al., Citation2008; Taylor et al., Citation2008). None of these is specifically oriented on pharmaceuticals. The number of studies that have examined the processes and applications of Fe- or Mn-based technologies to remove pharmaceuticals is growing. These studies indicate the special character of pharmaceuticals, that is, their complex chemical structures, which strongly influence compound specific physico-chemical and biological processes related to Fe or Mn reactive species. Therefore, an integrated review of this literature, on Fe- or Mn-based technologies and assessment of their scientific soundness, application potential, and main prevailing knowledge gaps, is highly needed.
This article reviews Fe- or Mn-based technologies which are used to remove pharmaceuticals from water. All these technologies are sorted based on 3 removal mechanisms: physico-chemical removal, chemical removal, and biologically related removal. We evaluate the benefits and limitations of these technologies and discuss their application. In addition, we suggest promising Fe- or Mn-based technologies that should be explored for pharmaceutical removal. The results of this review are thus a critical assessment of the currently available and future potential Fe- or Mn-based technologies for pharmaceutical removal.
2. Processes review
2.1. Physico-chemical removal
Fe and Mn compounds are used in water treatments, which involve physical and physico-chemical processes such as flocculation and coagulation, adsorption, and coprecipitation. These processes will be discussed in more detail in the following sections. Generally, pollutants are immobilized by interaction with Fe or Mn particles, which are subsequently settled. These physico-chemical technologies are used to remove pharmaceuticals from the water phase but not to convert or to degrade them.
2.1.1. Flocculation and coagulation
In flocculation and coagulation, the soluble or colloidal compounds are taken out of solution or suspension in the form of a floc or flake by using chemical flocculants or coagulants; subsequently, the formed particles are settled. Flocculation is the aggregation of particles while coagulation is a physico-chemical destabilization of the colloidal system (Benjamin and Lawler, Citation2013). In literature, the flocculation and coagulation is interchangeable, and in this review, we will use the term “flocculation and coagulation” to refer to either or both of these related processes as used in Bratby (Citation2006). These flocculants and coagulants can be organic polymers, metal salts such as FeCl3, and prehydrolyzed metal salts such as polyferric sulfate (Metcalf et al., Citation2004; Benjamin and Lawler, Citation2013).
Ferric salts, including FeCl3 and Fe2(SO4)3, are commonly used in flocculation and coagulation processes for organic matter removal from drinking water and wastewater (Budd et al., Citation2004; Matilainen et al., Citation2010; Liu et al., Citation2013). Excess Fe(III) is generated by zero-valent iron/H2O2 system and improves flocculation and coagulation of organic pollutants (Neyens and Baeyens, Citation2003; Kallel et al., Citation2009). Recently, polyferric sulfate (PFS), an inorganic polymeric flocculant, is used as a new coagulant to remove pharmaceuticals. In wastewater from a pharmaceutical production facility where the main organic COD (chemical oxygen demand) consisted of the pharmaceuticals, such as cefpirome, latomoxef, aztreonam, cefoperazone, cefatridine, ceftazidime, and other chemicals like propylene glycol, over 70% of 3300 mg/L COD was removed by flocculation and coagulation with PFS (Xing and Sun, Citation2009). Results indicate that both the pH and PFS concentration influenced the removal, with the highest removal being obtained at an optimum pH of ∼4 with 300 mg/L PFS. At pH 4, the COD removal increases from 0% to 80% with increasing PFS concentrations from 0 to 200 mg/L; when 200–900 mg/L PFS was dosed, COD removal only increased 10% (Xing and Sun, Citation2009). PFS contains large amounts of polynuclear complex ions such as (Fe2(OH)3)3+, (Fe2(OH)2)4+, and (Fe8(OH)20)4+, which leads to higher removal performance of organic compounds (Zouboulis et al., Citation2008; Verma et al., Citation2012), as compared to conventional flocculation and coagulation like FeSO4 and FeCl3.
Table 1. Pharmaceutical removal from water by Fe- or Mn-based technologies.
2.1.2. Adsorption
Fe, Mn, and their oxides can be used as adsorbents, especially when present as nanoparticles, that is, particles ranging in size between 10 and 100 nm. During adsorption, the pollutant is removed from the liquid phase through transfer to the surface of adsorbent. Especially nanoscale metal oxides have an extremely large specific surface area supporting efficient removal of pharmaceuticals (Tratnyek and Johnson, Citation2006). If the adsorbent has a preferential affinity for certain compounds in the liquid phase, the efficiencies can then be further enlarged with orders of magnitude (Metcalf et al., Citation2004).
The performance of pharmaceutical adsorption to Fe(III) has been evaluated by previous studies (Feitosa-Felizzola et al., Citation2009; Zhang and Huang, Citation2007; Brooks and Huggett, Citation2012). Two human-used macrolide antibacterial agents, clarithromycin and roxithromycin, adsorb strongly (>90%) to Fe(III) in the form of ferrihydrite. This is probably due to the macrolide antibacterial agents that can form a complex on the surface of Fe(III) (Feitosa-Felizzola et al., Citation2009). Due to the different properties of pharmaceuticals, the ability to form a complex with Fe(III) is different for each pharmaceutical, which leads to selective adsorption. The results indicate that the efficiency of adsorption is closely related to the process condition. For example, at weakly acidic pH (∼6), the highest adsorption is achieved due to changes in the Fe(III) surface chemistry, thus making Fe(III) more selective for the investigated pharmaceuticals (Zhang and Huang, Citation2007).
Nanoparticles and nanoscale materials including nano Fe species can also remove pollutants by adsorption. Due to their small size, ranging from 10 to 100 nm, and large specific surface area, nanoscale materials such as nanoscale zero-valent iron (nZVI) can efficiently remove pharmaceuticals via adsorption (Khin et al., Citation2012). For example, nZVI adsorption can contribute to the removal of carbamazepine (Shirazi et al., Citation2013), amoxicillin, and ampicillin (Ghauch et al., Citation2009). Supportive materials such as polyethylene glycol (PEG) and zeolite are used to improve the performance of nZVI as an adsorbent. Results show 10% more amoxicilline removal and 30% more ampicillin removal by PEG-nZVI, while nearly 30% more ampicilline removal is found by zeolite-nZVI (Ghauch et al., Citation2009). Another nanoscale Fe-related adsorbent in pharmaceutical removal is magnetic permanently confined micelle arrays (Mag-PCMAs), which has a magnetite core confined in a silica porous layer (Wang et al., Citation2009; Huang and Keller, Citation2013). Pharmaceuticals including atenolol, gemfibrozil, and sulfamethoxazole can be absorbed and removed at mg/L level by these Mag-PCMAs from water (Huang and Keller, Citation2013). Magnetic iron oxide nanoparticles (MxFe3-xO4) are used to remove organic pollutants via adsorption, where M represents one or more components from Fe, Mn, Co, Li, Ni, Zn, etc. MFe2O4 (M = Fe, Mn, Co, Zn) can remove more than 96% of tetracycline, oxytetracycline, and chlortetracycline at 100 µg/L by adsorption within 5 min (Bao et al., Citation2013). Incorporation of MxFe3-xO4 into other chemicals like activated carbon (AC) can also be used to remove pharmaceuticals. For example, the maximum adsorption capacity of MnFe2O4/AC to sulfamethoxazole is 159 mg/g at pH 7 (Wan et al., Citation2014), and maximum adsorption of hierarchically porous MgFe2O4/γ-Fe2O3 magnetic microspheres to minocycline is 201 mg/g (Lu et al., Citation2016). Metal-organic framework (MOF) is a class of highly crystalline porous materials consisting of a metal ion and an organic linker molecule (Khan et al., Citation2013). It is used to remove hazardous organics from water by adsorption and photocatalysis (Khan et al., Citation2013; Wang and Wang, Citation2015). MIL-100-Fe is a type of Fe-organic framework, and it is used in adsorption processes to remove pharmaceuticals including naproxen and clofibric acid (Hasan et al., Citation2012), as well as other organic pollutants like bisphenol A (Huo and Yan, Citation2012; Qin et al., Citation2014). The adsorption capacity of MIL-100-Fe can reach 100 mg/g, which is even higher than granular activated carbon (∼50 mg/g) (Hasan et al., Citation2012).
MnO2 can be used to remove pharmaceuticals and other pollutants by adsorption including antibacterial agents like sulfonamides and tetracycline, and endocrine disruptors (Bernard et al., Citation1997; Remucal and Ginder-Vogel, Citation2014). Results showed that clarithromycin and roxithromycin can be rapidly absorbed onto this amorphous MnO2 at pH 5 (Feitosa-Felizzola et al., Citation2009), but adsorption did not contribute to carbamazepine removal with amorphous MnO2 (He et al., Citation2012; Remucal and Ginder-Vogel, Citation2014). Similar to Fe(III), adsorption of pharmaceuticals onto MnO2 is also through surface complex forming. The different properties of pharmaceuticals affect the surface complex and will lead to different performances of adsorption.
2.1.3. Coprecipitation
Coprecipitation is a process in which soluble compounds are removed by sequestration in a precipitating phase (Pradyot, Citation2004; Noubactep, Citation2010). Pharmaceutical coprecipitation is observed with Fe species including Fe corrosion products (Filip et al., Citation2007; Ghauch et al., Citation2009; Noubactep, Citation2010). For example, amoxicillin and ampicillin can be sequestered by precipitation with Fe(OH)3 (Ghauch et al., Citation2009). Triazole, the raw material to produce antimycotic drugs such as terconazole, is also removed with zero-valent iron (ZVI) by coprecipitation (Jia et al., Citation2007; Noubactep, Citation2008b).
2.2. Chemical removal
In chemical removal of pharmaceuticals, Fe and Mn play important roles as oxidants, reductants, or catalysts such as Fe(II) in Fenton processes (Kochi, Citation1967; Guan et al., Citation2010; Chelliapan and Sallis, Citation2013; Remucal and Ginder-Vogel, Citation2014; Segura et al., Citation2015). Chemical removal of pharmaceuticals in water treatment occurs through chemical oxidation via oxidizing agents (Fe(III), Fe(VI), Mn(IV), Mn(VII)), or chemical reduction via reducing agents like nZVI to degrade a compound or a group of compounds (Lee et al., Citation2009; Guan et al., Citation2010). In addition to conventional oxidation processes, advanced oxidation processes (AOPs) including Fenton, photolysis, and ozonation are used to remove pharmaceuticals. In these processes, Fe and Mn species work as catalysts in the formation of free radicals such as hydroxyl radicals (OH•) and sulfate radicals (SO4•−), which are used as strong oxidants to destroy organic compounds.
2.2.1. Chemical oxidation
(1) Fe and Mn as oxidants
Fe(III), Fe(V), and Fe(VI) are used to oxidize pharmaceuticals from water, including tetracycline, clarithromycin, and roxithromycin (Wang et al., Citation2015). Both Fe(V) and Fe(VI) have a higher standard redox potential of +2.2 V under acidic pH 4–5 than under neutral pH 7 () (Jiang, Citation2007; Chen et al., Citation2010; Jiang, Citation2014). These ferrate compounds can react with pharmaceuticals which contain electron-rich moieties (Lee et al., Citation2009; Yang et al., Citation2012; Jiang and Zhou, Citation2013; Jiang et al., Citation2013), which leads to the formation of nontoxic by-products and Fe(III) (Sharma et al., Citation2005; Jiang et al., Citation2013). The removal efficiency varies for the different compounds at 10–100 µg/L were as follows: ciprofloxacin >60%, naproxen >40%, and n-acetyl sulfamethoxazole <10% (Yang et al., Citation2012; Jiang and Zhou, Citation2013; Jiang et al., Citation2013). In addition, 88% removal efficiencies were achieved for selected pharmaceuticals and other micropollutants by combining oxidation, and flocculation and coagulation with Fe(VI) (Yang et al., Citation2012). Owing to the high standard redox potential of Fe(III) (+0.770 V, ), the removal of tetracycline antibiotics, including tetracycline, oxytetracycline, and chlorotetracycline, with Fe(III) is 2–20 times higher than without Fe(III) (Wang et al., Citation2015). This process is influenced by pharmaceutical concentration as well as the concentration of Fe(III) (Wang et al., Citation2015). At pH 7 and 20°C, increasing of initial tetracycline concentration caused a decrease in the reaction rate, while increasing the initial Fe(III) concentration resulted in an increase in the reaction rate.
Figure 1. Eh-pH diagram for Metal in water at 25°C (A for Fe; B for Mn). Adapted from previous data (Stumm and Morgan, Citation2013) using Material Project website (Jain et al., Citation2013).
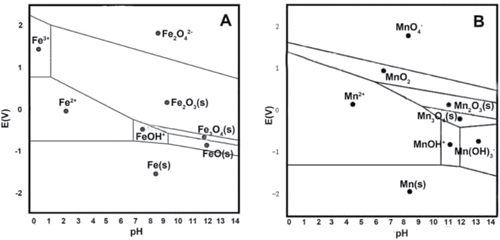
Mn(VII) and Mn(IV) are used to remove pharmaceuticals and other pollutants in water and wastewater because of their high standard redox potential (+1.23 V for Mn(IV) and +1.52 V for Mn(VII), see ) (Gao et al., Citation2012; He et al., Citation2012; Meerburg et al., Citation2012; Remucal and Ginder-Vogel, Citation2014; Tu et al., Citation2014; Li et al., Citation2015a). Permanganate (MnO4−) can be used to remove pharmaceuticals and other micropollutants containing electron-rich moieties (Guan et al., Citation2010; Hendratna, Citation2011). One study found that ciprofloxacin, lincomycin, and trimethoprim are removed in a second-order reaction from drinking water by Mn(VII), with rate constants of 0.61, 1.6, 3.6 M−1 s−1, respectively (Hu et al., Citation2010; Hu et al., Citation2011). In a second study, more than 90% of sulfamethoxazole at concentrations between 0.5 and 5 mg/L are removed with 2 mg/L Mn(VII) at pH 7 (Gao et al., Citation2014). Mn(VII) is also used to oxidize nonsteroidal anti-inflammatory drugs ibuprofen, diclofenac, naproxen, ketoprofen, fenoprofen, indomethacin, and salicylic acid (Rodriguez-Alvarez et al., Citation2013). Results showed diclofenac and indomethacin were completely removed, while the others decrease less than 30% (Rodriguez-Alvarez et al., Citation2013).
Mn(IV) (+1.23 V) has a stronger oxidation potential than Fe(III) (+0.77 V). This is observed in the faster transformation of clarithromycin and roxithromycin by Mn(IV), which has a 2–3 times higher reaction rate per surface unit than Fe(III) (Feitosa-Felizzola et al., Citation2009). Studies show that amorphous MnO2 can be used to remove pharmaceuticals such as carbamazepine, sulfamethazine, and diclofenac (Gao et al., Citation2012; He et al., Citation2012; Meerburg et al., Citation2012; Tu et al., Citation2014; Li et al., Citation2015a). This chemical removal process is pH dependent and effective when pH < 6 (Gao et al., Citation2012; He et al., Citation2012). Over 70% of diclofenac at about 3 mg/L is removed by oxidation in a MnO2 bed filter with amorphous MnO2 in natural environment, where adsorption of both parent compound and by-products of diclofenac oxidation is observed (Huguet et al., Citation2013; Huguet et al., Citation2014). Similarly, 95% of amoxicillin at around 360 mg/L is oxidized in 4 hrs with 1 × 1 molecular sieve-structured MnO2 while only 4.5% of amoxicillin is removed by adsorption (Kuan et al., Citation2013).
In addition to pH, other environmental parameters also affect chemical oxidation of pharmaceuticals by MnO2. For instance, MnO2 oxidation is negatively influenced by cosolute compounds, such as metal ions and natural organic compounds which inhibit the chemical reaction by occupying the reactive surface site of MnO2 (Zhang and Huang, Citation2003; Lin et al., Citation2009; Hendratna, Citation2011; He et al., Citation2012). In addition, reactant loading has influence on this oxidation process. For example, loading for triclosan, ciprofloxacin, and carbadox has no effect on reaction rate constants because of the fixed number of reactive surface sites of MnO2 (Zhang et al., Citation2008). In contrast, higher loading for tetracycline leads to lower reaction rate constants because of the self-competition for the same reactive surface sites of MnO2 (Zhang et al., Citation2008).
Most studies using MnO2 to remove pharmaceuticals are carried out under oxic condition. There are only two published studies using anoxic condition as control group. Results show that anoxic condition has no influence on fluoroquinolone removal (Zhang and Huang, Citation2005) while it can inhibit the sulfamethazine removal (Gao et al., Citation2012).
(2) Fe and Mn as catalysts
In classic Fenton's reagent (Fe/H2O2 system), a mixture of Fe(II) and hydrogen peroxide generates OH• () (Neyens and Baeyens, Citation2003; Pignatello et al., Citation2006). OH• can oxidize pharmaceuticals (Badawy et al., Citation2009; Li et al., Citation2012; Hussain et al., Citation2013) together with another oxidant produced in the process, for instance, Fe(IV) species at pH>5 (Eq. (Equation1(1) )) (Ogier, Citation2008; Hug and Leupin, Citation2003). For example, more than 90% berberine at around 1000 mg/L and over 70% metronidazole at 1 mg/L are removed in Fe/H2O2 oxidation systems (Shemer et al., Citation2006; Cui et al., Citation2015). In addition, nearly complete removal of paracetamol, chloramphenicol, and diclofenac is obtained (Badawy et al., Citation2009). In Fenton's reaction, ethylenediaminetetraacetic acid (EDTA) is used to improve the removal performance (Keenan and Sedlak, Citation2008; Laine et al., Citation2008; Zhou et al., Citation2010; Bautitz et al., Citation2012). The complex of Fe(II) and EDTA reduces dissolved O2 and produces H2O2 and OH• (Laine et al., Citation2008; Bautitz et al., Citation2012). In addition, the presence of EDTA enhances the Fe(III) solubility, thus preventing Fe(III) precipitation as well as possible Fe(II) coprecipitation with Fe(III) (Keenan and Sedlak, Citation2008; Bautitz et al., Citation2012). Additional flocculation and coagulation by Fe(III) improves removal in Fenton's reaction (Tekin et al., Citation2006).
(1)
Figure 2. Activated species generated in Fe-based advanced oxidation processes (AOPs) where (A) is for OH•-based AOPs (Neyens and Baeyens, Citation2003; Pignatello et al., Citation2006; Hartmann et al., Citation2010; Correia de Velosa and Pupo Nogueira, Citation2013) and (B) is for SO4•−-based AOPs (Ghauch et al., Citation2013; Ji et al., Citation2014), (Equation1(1) ) generated activated species from Fe/H2O2, photo/Fe/H2O2, electro/Fe/H2O2, sono/Fe/H2O2, etc.;(2) generated activated species from ZVI/O2;(3) generated activated species from photo degradation, electro degradation, sono degradation, etc.; and (4) generated activated species from Fe/persulfate.
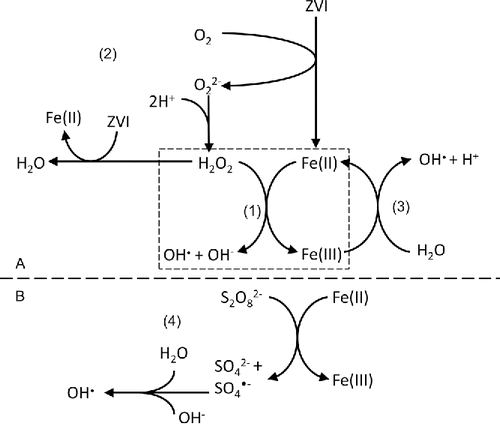
Other Fe species or compounds containing Fe can work as catalysts in Fenton's reactions. In these processes, pharmaceuticals and other micropollutants can be removed via oxidation (Correia de Velosa and Pupo Nogueira, Citation2013; Segura et al., Citation2013; Shirazi et al., Citation2013). For example, ZVI and nZVI are used as a catalyst in Fenton's reaction with H2O2 to remove pharmaceuticals (Segura et al., Citation2013; Shirazi et al., Citation2013) and other pollutants (Kowalski and Sogaard, Citation2014; Li et al., Citation2014; Segura et al., Citation2015). Under acidic conditions (pH < 3), ZVI is oxidized to Fe(II) on the surface of the metal and then Fe(II) catalyzes the Fenton process. In the presence of 1.2 g/L ZVI, 3.2 g/L H2O2, and 0.1 g/L total organic carbon (TOC), 80% mixture of pharmaceuticals and other organic pollutants are removed in 1 hr from pharmaceutical wastewater with a pH of 3 (Segura et al., Citation2013). Similarly, carbamazepine can be totally removed from both distilled water and groundwater with 20 mg/L nZVI and 25 mg/L H2O2 (Shirazi et al., Citation2013).
Furthermore, the Fenton's reaction can be enhanced by ultraviolet-visible photo, electronic, and ultrasonic irradiation (Babuponnusami and Muthukumar, Citation2012; Babuponnusami and Muthukumar, Citation2014), which are photo/Fe/H2O2 system, electro/Fe/H2O2 system, and sono/Fe/H2O2 system, respectively. In the photo/Fe/H2O2 system, a wavelength below 254 nm or higher than 300 nm enhances the photolysis of Fe(III) to Fe(II) () (Cruz et al., Citation2013; Trovo et al., Citation2009; Veloutsou et al., Citation2014). Via photo/Fe/H2O2 system, 16 mg/L metoprolol and 17.6 mg/L atenolol are completely removed within 150 min (Veloutsou et al., Citation2014), while 76% of 10 mg/L sulfamethoxazole is removed within 7 hrs (Trovo et al., Citation2009).
Electro-Fe(II)/H2O2 system is another advanced process in which H2O2 is generated continuously if sufficient O2 is present at a suitable cathode (Eq. (Equation2(2) ), ) (Brillas et al., Citation2009). In addition, the production of OH• is accelerated by the regeneration of Fe(II) from soluble Fe(III) (Eq. Equation3
(3) ).
(2)
(3)
Experiments with electro/Fe/H2O2 system indicate that this process is efficient for pharmaceutical removal (Sires et al., Citation2007; Isarain-Chavez et al., Citation2010; Olvera-Vargas et al., Citation2015). For example, 40% mineralization of atenolol at 158 mg/L (Isarain-Chavez et al., Citation2010) and nearly 30% mineralization of ranitidine at 33.8 mg/L (Olvera-Vargas et al., Citation2015) are obtained. Additionally, a combination of photo and electro irradiation for photo-electro/Fe/H2O2 system increases the performance in pharmaceutical removal even further (Isarain-Chavez et al., Citation2010; Olvera-Vargas et al., Citation2015). Results showed the complete removal of atenolol and 80% mineralization of ranitidine by photo-electro/Fe/H2O2 system with solar radiation. This is due to (Equation1(1) ) the production of more OH• than photo/Fe/H2O2 system or electro/Fe/H2O2 system alone () and (Equation2
(2) ) the regeneration of Fe(II) (Isarain-Chavez et al., Citation2010; Olvera-Vargas et al., Citation2015). Sono/Fe/H2O2 system has been employed to remove pharmaceuticals as ultrasonic irradiation improves the Fenton's reaction. Complete removal of levofloxacin is obtained by sono/Fe/H2O2 system in the presence of Fe3O4 magnetic nanoparticles (Wei et al., Citation2015).
Persulfate can be activated at pH 6 by different Fe species, such as ZVI, Fe(II), and Fe3O4. This process generates free SO4•− as well as other active species, such as OH• () (Yan et al., Citation2011; Ghauch et al., Citation2013; Ji et al., Citation2014). Standard redox potential of SO4•− is +2.6 V, which is similar to OH• with +2.72 V (Neta et al., Citation1988). Sulfate radical-based advanced oxidation processes (SR-AOPs) are new methods to oxidize pharmaceuticals in water (Yan et al., Citation2011; Ghauch et al., Citation2013; Shah et al., Citation2013; Ji et al., Citation2014).
In previous studies, Fe-activated persulfate has been used to remove pharmaceuticals. Fe(II)-activated persulfate oxidation removes 50% carbamazepine at 6 mg/L (Rao et al., Citation2014), and 96% ciprofloxacin and 75% sulfamethoxazole both at 7.6 mg/L (Ji et al., Citation2014). In micrometric ZVI-activated persulfate oxidation, 10 mg/L sulfamethoxazole is completely removed after 60 min (Ghauch et al., Citation2013). Additionally, more than 90% removal efficiency of sulfamonomethoxine at 16.8 mg/L is observed with Fe3O4-activated persulfate oxidation (Yan et al., Citation2011). Together with simulated solar light irradiation, complete removal of carbamazepine is obtained by an Fe(II)/persulfate/UV-Vis system, in which persulfate is activated by Fe(II) (Ahmed and Chiron, Citation2014). Furthermore, Fe can activate peroxymonosulfate (KHSO5, PMS) to generate SO4•−. One study shows that this Fe(II)-activated PMS can completely remove triclosan, sulfamethoxazole, and acetaminophen, which is more effective than Fe(II)-activated persulfate under the same conditions (Nfodzo and Choi, Citation2011).
MXFe3-XO4, including incorporation of MXFe3-XO4 to other chemicals, has also been used in catalytic oxidation processes to remove pharmaceuticals. A new synthetic material, core–shell-structured Fe3O4/α-MnO2 microspheres (Fe3O4/α-MnO2), is used to remove pharmaceuticals via catalytic oxidation with persulfate. In one study, removal is observed for ciprofloxacin: 90% of 50 mg/L by Fe3O4/ α-MnO2-activated persulfate, 80% by α-MnO2-activated persulfate, and almost 30% by Fe3O4-activated persulfate (Zhao et al., Citation2014).
Similarly to Fenton's reaction, Fe can work as a catalyst in other advanced oxidation processes including photo degradation, sono degradation, and ozonation. However, as opposed to Fenton or Fenton-like processes, these AOPs do not use H2O2 to generate OH• (Andreozzi et al., Citation2004; Ikehata and El-Din, Citation2006; Deng and Ezyske, Citation2011; Chelliapan and Sallis, Citation2013). Fe-ZnO is used in catalytic photo degradation, catalytic sono degradation, and catalytic photo-sono degradation, in which irradiation of ultrasonic frequencies or/and visible light can generate reactive radicals from water through forming vapor cavities (Madhavan et al., Citation2010). Results show that the removal rates for diclofenac increase in the following order: catalytic photo degradation (0.4 × 10−7 M min−1) < catalytic sono degradation (14.3 × 10−7 M min−1) < catalytic photo-sono degradation (15.3 × 10−7 M min−1) (Madhavan et al., Citation2010).
Even without H2O2, ZVI can catalyze processes in the presence of O2 () (Bautitz et al., Citation2012; Grcic et al., Citation2012; Correia de Velosa and Pupo Nogueira, Citation2013). 96% diazepam at 25 mg/L is removed by the ZVI/O2 system after 60 min. Moreover, 60% diazepam is mineralized (Bautitz et al., Citation2012). However, complexation of Fe may increase yield of H2O2 and change the nature of the reactive oxidant such as Fe(V) (Keenan and Sedlak, Citation2008).
Ozonation effectively removes pharmaceuticals because ozone is a strong oxidant with a standard redox potential of +2.08 V (Huber et al., Citation2003; Javier Benitez et al., Citation2009). Fe species are used in catalytic ozonation process and showed a high, stable catalytic activity (Lv et al., Citation2010). Removal efficiencies of more than 90% of herbicides and pharmaceuticals were achieved within 40 min with magnetic cobalt-doped Fe3O4 (FeCo), whereas less than 40% is removed without the catalyst. Hematite (α-Fe2O3) and Fe3O4 show catalytic activity in ozonation as well. The order of catalytic activity is FeCo > Fe3O4 > α-Fe2O3 (Lv et al., Citation2012).
Mn can also function as catalyst to remove pharmaceuticals. Manganese oxide supported or doped by other compounds has been used in catalytic ozonation process. Alumina-supported manganese oxide suspension is used as catalyst in ozonation to remove phenazone, ibuprofen, diphenhydramine, phenytoin, and diclofenac. Results show that more than 90% TOC of pharmaceuticals mixture is removed with alumina-supported manganese oxide, whereas only 20% is removed without a catalyst (Yang et al., Citation2009). Carbon nanotube-supported manganese oxides are used to assist ozone to remove ciprofloxacin. Ciprofloxacin removal increases within 15 min from 26.7% of 10 mg/L to 87.5% when the catalyst was added (Sui et al., Citation2012). MnO2-CuO/γ-Al2O3 catalyst is also used to remove ibuprofen in ozonation (Betancur-Corredor et al., Citation2015). Ibuprofen removal increases from 27% of 5 mg/L to 55% in the presence of the catalyst. A novel Ce-doped manganese oxide octahedral molecular sieve is generated recently and used as a catalyst in ozonation (Zhang et al., Citation2016); 81% ciprofloxacin of 10 mg/L was removed in the ozonation process with the catalyst. Both Mn-Ce-O catalyst prepared in the laboratory and commercial Fe-Mn-O catalyst are used in catalytic ozonation process and show similar catalytic capacity (Martins et al., Citation2015). Approximately 60% COD of the mixture of sulfamethoxazole and diclofenac is removed in the presence of either catalyst within 120 min. Tetracycline can complex with dissolved Mn2+ ions, thus improving tetracycline removal by oxygen. At pH 8–9.5, over 90% removal of tetracycline is achieved in Mn2+-mediated system, and the reactivity trend is as follows: oxytetracycline > tetracycline >> isochlorotetracycline (Chen and Huang, Citation2009).
2.2.2. Chemical reduction
Chemical reduction is often used to remove pollutants including heavy metals (Villacis-Garcia et al., Citation2015) and nitrate (Hou et al., Citation2015). However, its application for pharmaceutical removal has not been extensively studied. During chemical reduction, pharmaceuticals receive electrons from other chemical compounds. For example, (n)ZVI can reduce pharmaceuticals, resulting in the oxidation of Fe; this occurs alone or in conjunction with other removal processes such as adsorption (Noubactep, Citation2008a; Ghauch et al., Citation2009; Raychoudhury and Scheytt, Citation2013; Shirazi et al., Citation2013; Zhou et al., Citation2014). Previous studies showed that pharmaceutical compounds with certain functional groups (such as C-N, N = N, nitro or halogens) can be reduced by (n)ZVI via chemical reduction (Raychoudhury and Scheytt, Citation2013). Results indicated rapid reduction of amoxicillin and ampicillin, while less than 12.6% removal of carbamazepine is observed (Ghauch et al., Citation2009). Diazepam is removed by chemical reduction for almost 65% (Bautitz et al., Citation2012) In addition, complete removal of chloramphenicol is obtained (Xia, et al., Citation2014).
2.3. Biological-related removal
There are pharmaceutical removal processes related to the metal-related activity of different types of microorganisms, including bacteria and fungi (Kagle et al., Citation2009; Onesios et al., Citation2009). In these processes, microorganisms can produce Fe- or Mn-oxides, which are used to remove pharmaceuticals by chemical oxidation. In addition, bacteria can work in advanced oxidation together with Fe which is known as biologically catalyzed advanced oxidation (Marco-Urrea et al., Citation2009; Gros et al., Citation2014).
Biogenic Mn oxides (bioMnOx, also known as Mn bio-oxides) can efficiently remove pharmaceuticals (Forrez et al., Citation2011; Furgal et al., Citation2015) and other pollutants (Hennebel et al., Citation2009; Meng et al., Citation2009). These bioMnOx are produced by oxidation of Mn(II) by bacteria such as Pseudomonas putida MnB6 (BCCM/LMG 2322) and Bacillus sp. SG-1, or fungi (Hennebel et al., Citation2009; Tebo et al., Citation2004). Enrichment of P. putida which was used to generate bioMnOx was achieved under nitrifying conditions in a down flow sponge reactor with artificial wastewater (Cao et al., Citation2015). As compared to synthetic MnO2, microbially produced bioMnOx has a unique structure, yielding a variety of advantages for oxidation. BioMnOx are reactive under neutral pH (∼7), instead of the acidic conditions required for abiotically produced MnOx (Kuan et al., Citation2013; Remucal and Ginder-Vogel, Citation2014). Additionally, the bacteria can bind the intermediate Mn(III) compounds via ligands, thus effectively increasing the oxidative power of a Mn-bacteria mixture (Meerburg et al., Citation2012). As a result, diclofenac removal via adsorption and chemical oxidation with bioMnOx is 10-times faster than with synthetic MnO2 (Forrez et al., Citation2010). Additionally, complete removal of ciprofloxacin (Tu et al., Citation2014) and 17% removal of carbamazepine are obtained (Forrez et al., Citation2011). Studies also show that the performance will increase in the presence of trace metals, such as Ag or Pb. The interaction between the metals and the microorganism cell leads to the formation of reactive oxygen species (Meerburg et al., Citation2012). These reactive species can improve the pharmaceutical removal (Villalobos et al., Citation2004; Meerburg et al., Citation2012).
Biological Fenton-like system is a recently discovered biological advanced oxidation process in which radicals are generated in the presence of white-rot fungi Trametes versicolor. The biological Fenton-like reaction occurs in the presence of lignin-derived quinone (2,6,-dimethoxy-1,4-benzoquinone, DBQ) and Fe3+ () (Marco-Urrea et al., Citation2009; Gros et al., Citation2014). In this process, DBQ is reduced to hydroquinone (DBQH2) in the presence of an intracellular quinone reductase produced from the fungi, followed by the generation of semiquinone radicals (DBQ•−) via subsequent oxidation of DBQH2 by lignin-modifying enzymes (laccases and peroxidases) which are also from the white-rot fungi. Fenton's reagent is formed by DBQ•− auto-oxidation catalyzed by Fe3+, and OH• is produced via this cycling (Gomez-Toribio et al., Citation2009; Marco-Urrea et al., Citation2010). In this system, removal efficiencies of 80% were achieved for clofibric acid, carbamazepine, atenolol, and propranolol; more than 20% of the observed carbamazepine removal could be attributed to biological activity by this white-rot fungi (Marco-Urrea et al., Citation2010).
Figure 3. Biological Fenton's-like system catalyzed by white-rot fungi (Marco-Urrea et al., Citation2010) (Equation1(1) ) fungi catalyze the conversion of BDQ to DBQH2 by an intracellular quinone reductase from the fungi; (Equation2
(2) ) oxidation of DBQH2 to DBQ•− by lignin-modifying enzymes (laccases and peroxidases) from the white-rot fungi; (Equation3
(3) ) Fenton's reagent is formed by semiquinone radicals autoxidation catalyzed by Fe(III).
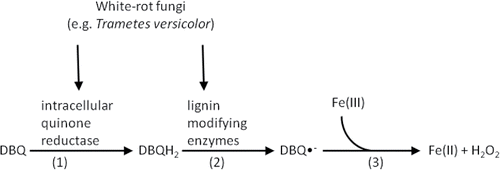
Manganese peroxidase (MnP) is an extracellular enzyme from the lignin-degrading basidiomycetes fungi such as Phanerochaete chrysosporium, which can oxidize Mn2+ to Mn3+. Mn3+ can act as a mediator to oxidize phenolic compounds. The presence of Mn2+ also stimulates the MnP production and functions as a substrate for MnP. The MnP is a key factor for fungi to remove pharmaceuticals including carbamazepine (Golan-Rozen et al., Citation2011; Santosa et al., Citation2012). Recently, a crude MnP is produced by white-rot fungi Phanerochaete chrysosporium and used as a biocatalyst to remove tetracycline and oxytetracycline via enzymatic degradation (Wen et al., Citation2010). Results show that 72.5% of 50 mg/L tetracycline and 84.3% of 50 mg/L oxytetracycline are removed at 40 U/L within 4 hr.
3. Evaluation and discussion
Fe- and Mn-based water treatment technologies have the potential to play important roles in pharmaceutical removal processes. However, there are some challenges to the direct application of available water technologies for the removal of pharmaceuticals. The four main challenges, including advantages and challenges, will be explained in detail in the paragraphs 3.1–3.4.
First of all, most described technologies are nonspecific, meaning that they can remove various pharmaceuticals as well as other pollutants. While this versatility can be an advantage, it can also lead to more consumption of reagents in order to also remove pharmaceuticals. Secondly, the high removal efficiency obtained with Fe- or Mn-based technologies require specific reaction conditions, which may result in more consumption of energy or chemicals. Finally, some Fe- or Mn-based technologies can oxidize the pharmaceuticals into by-products or intermediates. While these intermediates may be more biodegradable than the parent pharmaceutical, in some cases, these daughter compounds are either toxic or recalcitrant to further removal. Finally, the crystal structures and morphologies will also affect pharmaceutical removal performance. Additionally, using these Fe- and Mn-based technologies will introduce Fe or Mn compounds into the environment. In general, the presence of Fe or Mn in the environment is safe. However, some compounds used in Fe- or Mn-based technologies such as nZVI or persulfate can be toxic to the native microorganisms.
In the following section, we will evaluate the positive and negative aspects of using the currently available Fe- or Mn-based technologies for pharmaceutical removal, highlighting the challenges of application but also the potential of these technologies.
3.1. Nonspecificity
Many physico-chemical, chemical, and biologically related pharmaceutical removal processes with Fe or Mn are nonspecific. This improves the robustness and versatility of the technology, as most of these processes can remove various pharmaceuticals (). For example, Fe/H2O2/system is suitable for removing compounds with a range of chemical properties, including antibiotics, analgesics, beta-blockers, and lipid-lowering drugs. However, this versatility means that pharmaceuticals will be removed together with other organic and inorganic pollutants. For instance, bioMnOx is used to remove pharmaceuticals, and also heavy metals, such as Pb and As (Hennebel et al., Citation2009). This nonspecificity toward pharmaceuticals can reduce the removal efficiency, as other pollutants will be removed together with pharmaceuticals, thus competing for the available Fe or Mn. For example, organic compounds and colloids can compete for the surface of amorphous MnO2, leading to lower removal of carbamazepine (He et al., Citation2012). Even with selective oxidation processes like ferrate and permanganate oxidation, they will not only attack pharmaceuticals containing electron-rich organic moieties, but also aliphatic and aromatic organic compounds (Ghernaout and Naceur, Citation2011; Tiwari and Lee, Citation2011), phosphate (Lee et al., Citation2009), and taste and odor compounds (Srinivasan and Sorial, Citation2011). Thus, other compounds in the matrix compete with the pharmaceuticals for Fe or Mn. While this can achieve the goal of removing both common pollutants and pharmaceuticals, the nonspecificity of the process will increase the reagent consumption for removal of a certain amount of pharmaceuticals.
3.2. Treatment conditions
There are a variety of robust technologies that can achieve high pharmaceutical removal efficiencies. However, efficient and reliable removal can only be obtained under specific treatment conditions. Almost all pharmaceuticals can be completely removed by all AOPs, including SR-AOPs (). In addition, direct chemical oxidation (e.g., Fe(III), Mn(IV), ferrate, and permanganate oxidation) and chemical reduction (ZVI and nZVI reduction) result in more than 90% removal of pharmaceuticals. In order to achieve optimal removal, chemical treatments usually require acidic conditions (pH < 6). The sterilized condition for pure culture cultivation is used in biologically related pharmaceutical removal process. BioMnOx used for pharmaceutical removal can be produced by P. putida MnB6 (BCCM/LMG 2322), which is grown in synthetic medium (Forrez et al., Citation2010; Forrez et al., Citation2011; Meerburg et al., Citation2012; Furgal et al., Citation2015). Similarly, the biological Fenton-like system with the white-rot fungi T. versicolor (Marco-Urrea et al., Citation2010) requires an acidic medium (pH = 4.5) for its cultivation (Marco-Urrea et al., Citation2009; Marco-Urrea et al., Citation2010; Cruz-Morato et al., Citation2013; Gros et al., Citation2014). Thus, specific cultivation equipment and sterile conditions are needed, and this might limit further application of these technologies in pharmaceutical removal technologies.
3.3. Intermediates and by-products
In most pharmaceutical removal processes with Fe or Mn, incomplete mineralization may occur. For example, only 30%–40% mineralization is obtained in electro/Fe/H2O2 system processes (Isarain-Chavez et al., Citation2010; Olvera-Vargas et al., Citation2015). The presence and accumulation of by-products can be advantageous for further downstream treatment. For example, processes like Fe/H2O2/system will result in partial oxidation of compounds, thus improving the biodegradability of the pharmaceuticals in wastewater (Tekin et al., Citation2006; Badawy et al., Citation2009). A previous study using Fe/H2O2/system as pretreatment shows improved BOD/COD ratios from 0.25 to 0.50 following chemical oxidation, indicating improved biodegradability (Badawy et al., Citation2009). Thus, combining chemical oxidation of the pharmaceutical with biological treatment of the by-products can result in complete mineralization. Similarly, after ferrate treatment, biodegradation of water containing beta-blockers improves from nonbiodegradable to 14%–70% biodegradation (Wilde et al., Citation2013).
While improved biodegradability can be observed, some by-products will be similarly or even more toxic or recalcitrant as their parent compounds, especially by-products from the oxidation process. In a previous study, the toxicity of atenolol's intermediates from photo/Fe/H2O2 system process is higher than the parent compound (Veloutsou et al., Citation2014). Moreover, sulfamethoxazole removal from wastewater by solar photo/Fe/H2O2 system increases the toxicity of the wastewater from 16% to 86%, as assessed by Vibrio fischeri bioassays (Trovo et al., Citation2009). Another important product in these pharmaceutical removal processes is Fe2+ or Mn2+. Even though they are less threat to human health, they can have a negative effect on the taste, appearance, and staining of water. These results indicate that (Equation1(1) ) these treatment technologies must go beyond merely considering removal of the parent pharmaceutical, and (Equation2
(2) ) many treatment technologies will require a downstream step to ensure complete removal of all toxic compounds.
3.4. Effects of Fe or Mn compounds
Fe or Mn compounds exist in different crystal structures and morphologies, and this can lead to different pharmaceutical removal performance. For example, comparing different iron particles for pharmaceutical removal, ZVI has a faster removal rate for ampicillin than nZVI (Ghauch et al., Citation2009). Similarly, the oxidation efficiency of naproxen with α-MnO2 nanomaterials follows the order of commercial particles < nanorods < flower-like nanostructures < nanoparticles (Zhang et al., Citation2012).
In general, Fe or Mn compounds in pharmaceutical removal processes are safe to human or ecosystem. For example, nanomaterials like nZVI and MNPs are promising materials for pharmaceutical removal, but they are also potentially toxic to the environment. Previous studies show that nZVI results in ecotoxicity to organisms in both fresh water and marine water (Phenrat et al., Citation2009; Keller et al., Citation2012). Daphnia magna survival exposed to nZVI (Fe ≥ 5mg/L) drops within 96 h (Keller et al., Citation2012). In addition, nZVI is also potentially toxic to nerve cells, animal cells, and human cells (Phenrat et al., Citation2009; Crane and Scott, Citation2012; Kharisov et al., Citation2012). One possible explanation is the physical disruption of cell membranes by nZVI. It can also enhance the biocide effect of Fe (Lee et al., Citation2008). Toxicity to microorganisms is also observed with other Fe- or Mn-containing nanoparticles (Bellusci et al., Citation2014). Other compounds used in Fe- and Mn-based technologies like peroxymonosulfate are also hazardous for human (Olmez-Hanci et al., Citation2014).
4. Outlooks
The challenges and limitations of current Fe- or Mn-based pharmaceutical technologies discussed above require either further optimizing current technologies or developing new technologies (). These new technologies have many of the treatment advantages mentioned in Section 3, including nonspecificity, high removal efficiency, and/or potentially biodegradability improvements. These promising technologies require less specific treatment conditions and/or the products will be less toxic, thus addressing some of the challenges mentioned in Section 3.
4.1. Fe- or Mn-enhanced processes
There are some existing technologies for pharmaceutical removal which could be further enhanced through the addition of Fe and Mn. After improvement or enhancement, these technologies are likely to more efficiently remove pharmaceuticals.
Powdered activated carbon (PAC) adsorption is a physico-chemical removal process for pharmaceuticals (Lima et al., Citation2014; Mailler et al., Citation2015) and other organic pollutants (Quinlivan et al., Citation2005; Ho et al., Citation2011; Ghafoori et al., Citation2014). Studies show that the addition of Fe species can improve the performance of PAC adsorption (Han et al., Citation2015; Park et al., Citation2015). Research investigating the removal of bisphenol A and natural organic matter shows that the adsorption capacities were in the following sequence: bare PAC < hematite/PAC < magnetite/PAC < ferrihydrite/PAC (Park et al., Citation2015). These results show that adding Fe species can improve the adsorption of compounds onto PAC, which is a known physico-chemical process for pharmaceutical removal. While the adsorption on Fe/PAC is a nonspecific pharmaceutical removal process, this physico-chemical technique does not result in toxic products formation.
AOPs such as photo degradation and sono degradation can be accelerated by catalysts. Fe can work as a doping agent for catalysts such as TiO2 in catalytic photo degradation and sono degradation. In catalytic photo degradation, Fe-doped catalyst can improve the removal of volatile organic compounds acetone and benzyl alcohol (Zhou et al., Citation2006; Spasiano et al., Citation2013). Similarly, sono degradation catalyzed by Fe-doped TiO2 shows notably higher removal of the dyes Blue 4 dye and azo fuchsine than undoped TiO2 (Wang et al., Citation2008; Jamalluddin and Abdullah, Citation2011).
Wet oxidation is the oxidation of organic and inorganic substances in an aqueous solution or suspension by means of oxygen or air at 200°C–320°C and 2–20 MPa either in the presence or absence of catalysts (Levec and Pintar, Citation2007; Wang et al., Citation2007; Klavarioti et al., Citation2009; Kong et al., Citation2012). Wet oxidation includes wet air oxidation and wet peroxide oxidation and is used as a pretreatment technology for pharmaceutical wastewater (Melero et al., Citation2009; Hosseini et al., Citation2011; Zheng, Citation2011; Zhan et al., Citation2013). Results show that Fe and Mn (together with Ce, Pt, or C) can catalyze wet oxidation for phenolic compounds removal (Santiago et al., Citation2006; Rey et al., Citation2009; Collado et al., Citation2010; Garrido-Ramirez et al., Citation2012). AOPs can form OH• and other active oxygen species which are efficient and nonspecific in pharmaceutical removal processes. In previous studies, Mn is used as a catalyst in supercritical water oxidation to treat pharmaceutical laboratory wastewater (Ruamchat et al., Citation2006). In addition, results show wet oxidation can significantly improve the biodegradability of industrial pharmaceutical wastewater (Gotvajn et al., Citation2007; Zhan et al., Citation2013; Pantelidou et al., Citation2014). For example, the ratio of BOD5/COD in pharmaceutical wastewater increases from 0.1 to 0.75 by catalytic wet air oxidation at 220°C (Zhan et al., Citation2013).
4.2. Advanced Fe or Mn compounds
Advanced Fe or Mn compounds such as Fe-organic framework, nZVI, and bioMnOx are used to remove pharmaceuticals. These Fe or Mn compounds will be synthetized by combining with other materials as well as developing new generation processes. These advanced Fe or Mn compounds may lead to better pharmaceutical removal.
Some advanced Fe or Mn compounds are used as catalysts in AOPs (Fenton, photo degradation, and SR-AOPs), which are currently employed to remove pharmaceuticals. Nano particulate Fe oxides (nano FeOx) is a newly synthetic Fe compound which can catalyze Fenton's reaction (Zelmanov and Semiat, Citation2008; Zelmanov and Semiat, Citation2009). Results show that higher concentrations of nano FeOx lead to a higher reaction rate (Zelmanov and Semiat, Citation2008; Zelmanov and Semiat, Citation2009). Moreover, nano FeOx can improve the removal of pollutants via adsorption because of the large specific surface area of these nanoparticles (Zelmanov and Semiat, Citation2008; Zelmanov and Semiat, Citation2009). Metal-organic framework containing Fe or Mn is used as a catalyst in photo degradation with both visible and UV irradiation (Wang et al., Citation2014). Another new synthetic AOPs catalyst is ferrite nanoparticles (MxFe3-xO4 nanoparticles, M = Mn, Fe, Co, etc.). For example, MnFe2O4 (MFN) can catalyze photo degradation or Fenton's reactions. Over 90% removal of dye compounds was obtained (Mahmoodi, Citation2013). MFN can also activate PMS, which is used to remove pollutants via SR-AOPs (Yao et al., Citation2014). In this removal process, adsorption is an important mechanism (Wang et al., Citation2011). Incorporation of MxFe3-xO4 to metal oxides or carbon is also promising in pharmaceutical removal. For example, Fe3O4–Cr2O3 magnetic nanocomposite (MNC) is used to catalyze photo degradation. This MNC shows efficient removal of 4 chlorophenol under UV irradiation, good magnetic separation for recovery, and recyclability (Singh et al., Citation2015). Kaolinite-supported nZVI (k-nZVI) is recently synthesized (Üzüm et al., Citation2009; Chen et al., Citation2013b). nZVI is proven to remove pharmaceuticals by adsorption and chemical reduction (Ghauch et al., Citation2009; Shirazi et al., Citation2013), and it can catalyze Fenton-like processes to remove pharmaceuticals. In addition, kaolinite alone can remove the pharmaceutical metoprolol by adsorption together with talc (Li et al., Citation2015b). Thus, this newly synthesized k-nZVI is a promising reagent for pharmaceutical removal.
Biologically produced MnOx has been shown to remove pharmaceuticals. Similar to bioMnOx, Fe oxides can also be generated by microbial activity, resulting in bioFeOx (Hennebel et al., Citation2009). Their potential to remove pollutants has been observed. The large surface area of bioFeOx leads to the adsorption of heavy metals as well as potential adsorption of pharmaceuticals (Hennebel et al., Citation2009; Forrez et al., Citation2011). In addition, bioFeOx is effective in dehalogenation reactions of organic pollutants, including chlorinated solvents, pesticides, and freons (Komlos et al., Citation2007; Hennebel et al., Citation2009). For pharmaceuticals containing halogens like diclofenac, applicability of dehalogenation by bioFeOx should be investigated.
4.3. Other promising processes
Some novel processes with Fe or Mn compounds are also promising in pharmaceutical removal. These processes should be explored to determine their application for pharmaceutical removal.
Due to the similar properties between Fe and Mn (), Mn can work as a catalyst in Fenton's reaction (Ma et al., Citation2004; Kouraichi et al., Citation2010; Yao et al., Citation2014). In Mn/H2O2 system, both amorphous and crystalline Mn(IV) compounds are used as catalysts to remove carbon tetrachloride, with removal efficiencies of ∼90% at pH 6 (Watts et al., Citation2005). OH• is generated in Mn/H2O2 system, and it is applicable to remove pharmaceuticals. Moreover, the OH• is generated at nearly neutral conditions (pH 6), instead of the acidic conditions at pH 3 that are needed for Fe/H2O2 system (Pignatello et al., Citation2006). Consequently, Mn/H2O2 will be more cost-efficient for pharmaceutical removal in practice.
Dissimilatory Fe or Mn reduction is demonstrated as a process to remove organic pollutants (Lovley and Phillips, Citation1988; Salas et al., Citation2009; Rosenberg et al., Citation2013; Chen et al., Citation2013a). In this process, dissimilatory Fe-reducing bacteria (DIRB), such as Geobacter metallireducens, metabolically degrade organic matter while respiring on Fe(III) or Mn(IV). Dissimilatory iron or manganese reduction has been applied for the removal of monoaromatic compounds, such as benzene, toluene, ethylbenzene, and xylene isomers (BTEX) (Langenhoff et al., Citation1996b; Anderson et al., Citation1998; Villatoro-Monzon et al., Citation2003; Jahn et al., Citation2005;; Rosenberg et al., Citation2013). A previous study shows the complete removal of BTEX with Fe(III) at a rate of 0.19 μmol/(L·d) (Villatoro-Monzon et al., Citation2003). Dissimilatory iron reduction has been shown for a variety of soluble and insoluble Fe(III) forms, indicating the versatility of this process (Lovley, Citation1991; Lovley et al., Citation2004). We assume that biological processes which can remove phenolic or aromatic compounds might have the potential to remove pharmaceuticals, as many pharmaceuticals contain at least one aromatic ring. Thus, dissimilatory Fe reduction was potential to remove pharmaceuticals.
Mn(IV) has similar characteristics and an even higher standard redox potential compared to Fe(III). In addition, Mn(IV) can be used as an alternative electron acceptor by some DIRB (Rosenberg et al., Citation2013). Together with Mn(IV)-reducing bacteria, the process is employed to remove aromatic compounds, for example, BTEX (Dorer et al., Citation2016; Villatoro-Monzon et al., Citation2003). Results show that complete biodegradation of BTEX (Villatoro-Monzon et al., Citation2003) and >60% naphthalene is mineralized to CO2 with Mn(IV) as the terminal electron acceptor (Langenhoff et al., Citation1996a).
The mentioned dissimilatory Fe or Mn reduction requires a neutral pH and anoxic conditions, so there are no needs to adjust the pH or add large amounts of oxygen. These prerequisites make it an attractive, sustainable, and low-cost technology. This biological removal process will remove not only pharmaceuticals but also other organic materials. If given enough time, bacteria will degrade and eventually completely mineralize the pharmaceuticals. Products of the process, Fe(II) or Mn(II), can be oxidized into Fe(III) or Mn(IV), thus be recycled and re-used for the removal process.
5. Conclusions
Fe- or Mn-based technologies are capable of removing pharmaceuticals in water systems. Removing pharmaceuticals decreases the possibility of developing antibiotic-resistant gene or antibiotic-resistant bacteria. Furthermore, the AOPs using Fe or Mn compounds can also remove antibiotic-resistant gene and antibiotic-resistant bacteria (Michael et al., Citation2012; Sousa et al., Citation2016). These technologies are efficient for a wide variety of pharmaceuticals, as well as other pollutants under favorable conditions. As discussed in this review, the removal mechanism involved physico-chemical, chemical, and biologically related processes. So far, current Fe- or Mn-based technologies to remove pharmaceuticals focus on chemical removal. The Fe or Mn compounds are generally safe to the human and environment, but there are still some of them like nZVI can be toxic. Even when these technologies partially remove pharmaceuticals, their by-products can be more biodegradable than the parent compounds. These challenges require further attention to optimize these technologies. We have also discussed that Fe- or Mn-based technologies look promising in pharmaceutical removal processes and are worth studying, for example, based on combined technologies and/or biological processes. Especially biological processes are interesting, due to their mild operational conditions and environmental-friendly characteristics.
The combination of current and potential Fe- or Mn-based processes is therefore promising to develop novel technologies for the removal of pharmaceuticals from (waste)water. Furthermore, all these Fe- or Mn-based technologies are also valuable for removal both conventional pollutants and micropollutants.
Abbreviations
AC | = | activated carbon |
Al-MnO2 | = | alumina-supported manganese oxide |
AOPs | = | advanced oxidation processes |
bioFeOx | = | biogenic Fe oxides |
bioMnOx | = | biogenic Mn oxides |
BTEX | = | benzene, toluene, ethylbenzene, and xylenes |
COD | = | chemical oxygen demand |
DBQ | = | 2,6,-dimethoxy-1,4-benzoquinone |
DBQ•− | = | semiquinone radicals |
DBQH2 | = | hydroquinone |
DIRB | = | dissimilatory Fe-reducing bacteria |
EDTA | = | ethylenediaminetetraacetic acid |
Fe3O4/α-MnO2 | = | core–shell structured Fe3O4/α-MnO2 microspheres |
FeCo | = | magnetic cobalt-doped Fe3O4 |
k-nZVI | = | kaolinite-supported nanoscale zero-valent iron |
Mag-PCMAs | = | magnetic permanently confined micelle arrays |
MXFe3-XO4 | = | magnetic iron oxide nanoparticles |
MFN | = | manganese ferrite nanoparticles (MnFe2O4) |
MNC | = | Fe3O4–Cr2O3 magnetic nanocomposite |
MnP | = | manganese peroxidase |
MNPs | = | Fe3O4 magnetic nanoparticles |
MOF | = | metal-organic framework |
nano Fe-C | = | Fe-C nanocomposite |
nano FeOx | = | nano particulate Fe–oxides |
nZVI | = | nanoscale zero-valent iron |
OH• | = | hydroxyl radical |
PAC | = | powdered activated carbon |
PEG | = | polyethylene glycol |
PFS | = | polyferric sulfate |
PMS | = | peroxymonosulfate (KHSO5) |
SO4•− | = | sulfate radical |
SR-AOPs | = | sulfate radical-based advanced oxidation processes |
TOC | = | total organic carbon |
UV-Vis | = | Ultraviolet-visible |
ZVI | = | zero-valent iron |
Acknowledgments
The authors thank Dr Thomas ter Laak (KWR Watercycle Research Institute, the Netherlands), Justine van Enennaam, and Thomas Wagner (both from Wageningen University) for valuable discussions.
Funding
Funding is supported by China Scholarship Council (CSC, File No.201308610161) and Wageningen University, the Netherlands.
References
- Ahmed, M.M., and Chiron, S. (2014). Solar photo-Fenton like using persulphate for carbamazepine removal from domestic wastewater. Water Res., 48, 229–236.
- Anderson, R.T., Rooney-Varga, J.N., Gaw, C.V., and Lovley, D.R. (1998). Anaerobic benzene oxidation in the Fe(III) reduction zone of petroleum contaminated aquifers. Environ. Sci. Technol., 32, 1222–1229.
- Andreozzi, R., Campanella, L., Fraysse, B., Garric, J., Gonnella, A., Giudice, R.L., Marotta, R., Pinto, G., and Pollio, A. (2004). Effects of advanced oxidation processes (AOPs) on the toxicity of a mixture of pharmaceuticals. Water Sci. Technol., 50, 23–28.
- Babuponnusami, A., and Muthukumar, K. (2012). Advanced oxidation of phenol: A comparison between Fenton, electro-Fenton, sono-electro-Fenton and photo-electro-Fenton processes. Chem. Eng. J., 183, 1–9.
- Babuponnusami, A., and Muthukumar, K. (2014). A review on Fenton and improvements to the Fenton process for wastewater treatment. J. Environ. Chem. Eng., 2, 557–572.
- Badawy, M.I., Wahaab, R.A., and El-Kalliny, A.S. (2009). Fenton-biological treatment processes for the removal of some pharmaceuticals from industrial wastewater. J. Hazard Mater., 167, 567–574.
- Bae, S., Song, S.U., Kim, D., and Lee, W. (2012). Oxidative degradation of pharmaceutical by Fenton-like reaction using pyrite and H2O2. [abstract No. EVNR 203]. In: The 244th ACS National Meeting & Exposition, At Philadelphia, Pennsylvania, USA.
- Bao, X., Qiang, Z., Ling, W., and Chang, J.H. (2013). Sonohydrothermal synthesis of MFe2O4 magnetic nanoparticles for adsorptive removal of tetracyclines from water. Sep. Purif. Technol., 117, 104–110.
- Bautitz, I.R., Velosa, A.C., and Nogueira, R.F. (2012). Zero valent iron mediated degradation of the pharmaceutical diazepam. Chemosphere 88, 688–692.
- Bellusci, M., La Barbera, A., Padella, F., Mancuso, M., Pasquo, A., Grollino, M.G., Leter, G., Nardi, E., Cremisini, C., Giardullo, P., and Pacchierotti, F. (2014). Biodistribution and acute toxicity of a nanofluid containing manganese iron oxide nanoparticles produced by a mechanochemical process. Int. J. Nanomed., 9, 1919–1929.
- Benjamin, M.M., and Lawler, D.F. (2013). Water quality engineering: Physical/chemical treatment processes. Hoboken, NJ: John Wiley & Sons, Inc.
- Bernard, S., Chazal, P., and Mazet, M. (1997). Removal of organic compounds by adsorption on pyrolusite (β-MnO2). Water Res., 31, 1216–1222.
- Betancur-Corredor, B., Soltan, J., and Peñuela, G.A. (2015). Mineralization of Ibuprofen and Humic Acid through Catalytic Ozonation. Ozone: Sci. Eng., 38, 203–210.
- Bratby, J. (2006). Coagulation and flocculation in water and wastewater treatment. London: IWA Publishing.
- Brillas, E., Sires, I., and Oturan, M.A. (2009). Electro-Fenton process and related electrochemical technologies based on Fenton's reaction chemistry. Chem. Rev., 109, 6570–6631.
- Brooks, B.W., and Huggett, D.B. (2012). Human pharmaceuticals in the environment: Current and future perspectives. New York: Springer.
- Budd, G.C., Hess, A.F., Shorney-Darby, H., Neemann, J.J., Spencer, C.M., Bellamy, J.D., and Hargette, P.H. (2004). Coagulation applications for new treatment goals. J. Am. Water Works Assoc., 96, 102–113.
- Cao, L.T., Kodera, H., Abe, K., Imachi, H., Aoi, Y., Kindaichi, T., Ozaki, T., and Ohashi, A. (2015). Biological oxidation of Mn(II) coupled with nitrification for removal and recovery of minor metals by downflow hanging sponge reactor. Water Res., 68, 545–553.
- Chelliapan, S., and Sallis, P.J. (2013). Removal of organic compound from pharmaceutical wastewater using advanced oxidation processes. J. Sci. Ind. Res., 72, 248–254.
- Chen, M., Cao, F., Li, F., Liu, C., Tong, H., Wu, W., and Hu, M. (2013a). Anaerobic transformation of DDT related to iron(III) reduction and microbial community structure in paddy soils. J. Agric. Food Chem., 61, 2224–2233.
- Chen, W.-R., Ding, Y., Johnston, C.T., Teppen, B.J., Boyd, S.A., and Li, H. (2010). Reaction of Lincosamide Antibiotics with Manganese Oxide in Aqueous Solution. Environ. Sci. Technol., 44, 4486–4492.
- Chen, W.R., and Huang, C.H. (2009). Transformation of tetracyclines mediated by Mn(II) and Cu(II) ions in the presence of oxygen. Environ. Sci. Technol., 43, 401–407.
- Chen, Z., Wang, T., Jin, X., Chen, Z., Megharaj, M., and Naidu, R. (2013b). Multifunctional kaolinite-supported nanoscale zero-valent iron used for the adsorption and degradation of crystal violet in aqueous solution. J. Colloid Interface Sci., 398, 59–66.
- Chieng, N., Rades, T., and Aaltonen, J. (2011). An overview of recent studies on the analysis of pharmaceutical polymorphs. J. Pharm. Biomed. Anal., 55, 618–644.
- Collado, S., Quero, D., Laca, A., and Diaz, M. (2010). Fe2+-catalyzed wet oxidation of phenolic acids under different pH values. Ind. Eng. Chem. Res., 49, 12405–12413.
- Correia de Velosa, A., and Pupo Nogueira, R.F. (2013). 2,4-Dichlorophenoxyacetic acid (2,4-D) degradation promoted by nanoparticulate zerovalent iron (nZVI) in aerobic suspensions. J. Environ. Manage., 121, 72–79.
- Crane, R.A., and Scott, T.B. (2012). Nanoscale zero-valent iron: Future prospects for an emerging water treatment technology. J. Hazard. Mater., 211–212, 112–125.
- Cruz-Morato, C., Ferrando-Climent, L., Rodriguez-Mozaz, S., Barcelo, D., Marco–Urrea, E., Vicent, T., and Sarra, M. (2013). Degradation of pharmaceuticals in non-sterile urban wastewater by Trametes versicolor in a fluidized bed bioreactor. Water Res., 47, 5200–5210.
- Cruz, N.d.l., Esquius, L., Grandjean, D., Magnet, A., Tungler, A., Alencastro, L.F.d., and Pulgarin, C. (2013). Degradation of emergent contaminants by UV, UV/H2O2 and neutral photo-Fenton at pilot scale in a domestic wastewater treatment plant. Water Res., 47, 5836–5845.
- Cui, X.Y., Zeng, P., Qiu, G.L., Liu, Y.Q., Song, Y.H., Xie, X.L., and Han, L. (2015). Pilot-scale treatment of pharmaceutical berberine wastewater by Fenton oxidation. Environ. Earth Sci., 73, 4967–4977.
- Cundy, A.B., Hopkinson, L., and Whitby, R.L. (2008). Use of iron-based technologies in contaminated land and groundwater remediation: a review. Sci. Total Environ., 400, 42–51.
- Deng, Y., and Ezyske, C.M. (2011). Sulfate radical-advanced oxidation process (SR-AOP) for simultaneous removal of refractory organic contaminants and ammonia in landfill leachate. Water Res., 45, 6189–6194.
- Dorer, C., Vogt, C., Neu, T.R., Stryhanyuk, H., and Richnow, H.-H. (2016). Characterization of toluene and ethylbenzene biodegradation under nitrate-, iron(III)- and manganese(IV)-reducing conditions by compound-specific isotope analysis. Environ. Pollut., 211, 271–281.
- Dougherty, J.A., Swarzenski, P.W., Dinicola, R.S., and Reinhard, M. (2010). Occurrence of herbicides and pharmaceutical and personal care products in surface water and groundwater around Liberty Bay, Puget Sound, Washington. J. Environ. Qual., 39, 1173–1180.
- Feitosa-Felizzola, J., Hanna, K., and Chiron, S. (2009). Adsorption and transformation of selected human-used macrolide antibacterial agents with iron(III) and manganese(IV) oxides. Environ. Pollut., 157, 1317–1322.
- Filip, J., Zboril, R., Schneeweiss, O., Zeman, J., Cernik, M., Kvapil, P., and Otyepka, M. (2007). Environmental applications of chemically pure natural ferrihydrite. Environ. Sci. Technol., 41, 4367–4374.
- Forrez, I., Carballa, M., Verbeken, K., Vanhaecke, L., Ternes, T., Boon, N., and Verstraete, W. (2010). Diclofenac oxidation by biogenic manganese oxides. Environ. Sci. Technol., 44, 3449–3454.
- Forrez, I., Carballa, M., Fink, G., Wick, A., Hennebel, T., Vanhaecke, L., Ternes, T., Boon, N., and Verstraete, W. (2011). Biogenic metals for the oxidative and reductive removal of pharmaceuticals, biocides and iodinated contrast media in a polishing membrane bioreactor. Water Res., 45, 1763–1773.
- Furgal, K.M., Meyer, R.L., and Bester, K. (2015). Removing selected steroid hormones, biocides and pharmaceuticals from water by means of biogenic manganese oxide nanoparticles in situ at ppb levels. Chemosphere 136, 321–326.
- Gao, A.F., Gao, H., Wan, J.M., and Wu, C.S. (2013). Treatment pharmaceutical wastewater using fenton oxidation, ultraviolet, and ultrasound processes. Fresenius Environ. Bull., 22, 449–454.
- Gao, J., Hedman, C., Liu, C., Guo, T., and Pedersen, J.A. (2012). Transformation of sulfamethazine by manganese oxide in aqueous solution. Environ. Sci. Technol., 46, 2642–2651.
- Gao, S., Zhao, Z., Xu, Y., Tian, J., Qi, H., Lin, W., and Cui, F. (2014). Oxidation of sulfamethoxazole (SMX) by chlorine, ozone and permanganate–a comparative study. J. Hazard Mater., 274, 258–269.
- Garrido-Ramirez, E.G., Sivaiah, M.V., Barrault, J., Valange, S., Theng, B.K.G., Ureta-Zanartu, M.S., and Mora, M.D. (2012). Catalytic wet peroxide oxidation of phenol over iron or copper oxide-supported allophane clay materials: Influence of catalyst SiO2/Al2O3 ratio. Microporous and Mesoporous Mater., 162, 189–198.
- Ghafoori, S., Shah, K.K., Mehrvar, M., and Chan, P.K. (2014). Pharmaceutical wastewater treatment using granular activated carbon and UV/H2O2 processes: Experimental analysis and modelling. Can. J. Chem. Eng., 92, 1163–1173.
- Ghauch, A., Tuqan, A., and Assi, H.A. (2009). Antibiotic removal from water: elimination of amoxicillin and ampicillin by microscale and nanoscale iron particles. Environ. Pollut., 157, 1626–1635.
- Ghauch, A., Ayoub, G., and Naim, S. (2013). Degradation of sulfamethoxazole by persulfate assisted micrometric Fe0 in aqueous solution. Chem. Eng. J., 228, 1168–1181.
- Ghernaout, D., and Naceur, M.W. (2011). Ferrate(VI): In situ generation and water treatment - a review. Desalin. Water Treat., 30, 319–332.
- Golan-Rozen, N., Chefetz, B., Ben-Ari, J., Geva, J., and Hadar, Y. (2011). Transformation of the recalcitrant pharmaceutical compound carbamazepine by Pleurotus ostreatus: Role of cytochrome P450 monooxygenase and manganese peroxidase. Environ. Sci. Technol., 45, 6800–6805.
- Gomez-Toribio, V., Garcia-Martin, A.B., Martinez, M.J., Martinez, A.T., and Guillen, F. (2009). Induction of extracellular hydroxyl radical production by white-rot fungi through quinone redox cycling. Appl. Environ. Microbiol., 75, 3944–3953.
- Gotvajn, A.Z., Zagorc-Koncan, J., and Tisler, T. (2007). Pretreatment of highly polluted pharmaceutical waste broth by wet air oxidation. J. Environ. Eng., 133, 89–94.
- Grcic, I., Papic, S., Zizek, K., and Koprivanac, N. (2012). Zero-valent iron (ZVI) Fenton oxidation of reactive dye wastewater under UV–C and solar irradiation. Chem. Eng. J., 195, 77–90.
- Gros, M., Cruz-Morato, C., Marco-Urrea, E., Longree, P., Singer, H., Sarra, M., Hollender, J., Vicent, T., Rodriguez-Mozaz, S., and Barcelo, D. (2014). Biodegradation of the X-ray contrast agent iopromide and the fluoroquinolone antibiotic ofloxacin by the white rot fungus Trametes versicolor in hospital wastewaters and identification of degradation products. Water Res., 60, 228–241.
- Guan, X.H., He, D., Ma, J., and Chen, G.H. (2010). Application of permanganate in the oxidation of micropollutants: a mini review. Front. Environ. Sci. Eng. China 4, 405–413.
- Han, Z., Sani, B., Akkanen, J., Abel, S., Nybom, I., Karapanagioti, H.K., and Werner, D. (2015). A critical evaluation of magnetic activated carbon's potential for the remediation of sediment impacted by polycyclic aromatic hydrocarbons. J. Hazard Mater., 286, 41–47.
- Hartmann, M., Kullmann, S., and Keller, H. (2010). Wastewater treatment with heterogeneous Fenton-type catalysts based on porous materials. J. Mater. Chem., 20, 9002–9017.
- Hasan, Z., Jeon, J., and Jhung, S.H. (2012). Adsorptive removal of naproxen and clofibric acid from water using metal-organic frameworks. J. Hazard Mater., 209–210, 151–157.
- He, Y., Xu, J., Zhang, Y., Guo, C., Li, L., and Wang, Y. (2012). Oxidative transformation of carbamazepine by manganese oxides. Environ. Sci. Pollut. Res., 19, 4206–4213.
- He, Y.J., Sutton, N.B., Rijnaarts, H.H.H., and Langenhoff, A.A.M. (2016). Degradation of pharmaceuticals in wastewater using immobilized TiO2 photocatalysis under simulated solar irradiation. Appl. Catal. B Environ., 182, 132–141.
- Hendratna, A. (2011). The application of MnO2 and KMnO4 for persistent organic compounds and COD removals in wastewater treatment process. Department of Land and Water Resources Engineering. STOCKHOLM: Royal Institute of Technology.
- Hennebel, T., De Gusseme, B., Boon, N., and Verstraete, W. (2009). Biogenic metals in advanced water treatment. Trends Biotechnol., 27, 90–98.
- Ho, L., Lambling, P., Bustamante, H., Duker, P., and Newcombe, G. (2011). Application of powdered activated carbon for the adsorption of cylindrospermopsin and microcystin toxins from drinking water supplies. Water Res., 45, 2954–2964.
- Hosseini, A.M., Tungler, A., and Bakos, V. (2011). Wet oxidation properties of process waste waters of fine chemical and pharmaceutical origin. Reaction Kinet. Mech. Catal., 103, 251–260.
- Hou, M., Tang, Y., Xu, J., Pu, Y., Lin, A., Zhang, L., Xiong, J., Yang, X.J., and Wan, P. (2015). Nitrate reduction in water by aluminum–iron alloy particles catalyzed by copper. J. Environ. Chem. Eng., 3, 2401–2407.
- Hu, L., Martin, H.M., and Strathmann, T.J. (2010). Oxidation kinetics of antibiotics during water treatment with potassium permanganate. Environ. Sci. Technol., 44, 6416–6422.
- Hu, L., Stemig, A.M., Wammer, K.H., and Strathmann, T.J. (2011). Oxidation of antibiotics during water treatment with potassium permanganate: reaction pathways and deactivation. Environ. Sci. Technol., 45, 3635–3642.
- Huang, Y.X., and Keller, A.A. (2013). Magnetic nanoparticle adsorbents for emerging organic contaminants. Acs Sustain. Chem. Eng., 1, 731–736.
- Huber, M.M., Canonica, S., Park, G.Y., and von Gunten, U. (2003). Oxidation of pharmaceuticals during ozonation and advanced oxidation processes. Environ. Sci. Technol., 37, 1016–1024.
- Huerta-Fontela, M., Galceran, M.T., and Ventura, F. (2011). Occurrence and removal of pharmaceuticals and hormones through drinking water treatment. Water Res., 45, 1432–1442.
- Hug, S.J., and Leupin, O. (2003). Iron-catalyzed oxidation of arsenic(III) by oxygen and by hydrogen peroxide: pH-dependent formation of oxidants in the Fenton reaction. Environ. Sci. Technol., 37, 2734–2742.
- Huguet, M., Simon, V., and Gallard, H. (2014). Transformation of paracetamol into 1,4-benzoquinone by a manganese oxide bed filter. J. Hazard Mater., 271, 245–251.
- Huguet, M., Deborde, M., Papot, S., and Gallard, H. (2013). Oxidative decarboxylation of diclofenac by manganese oxide bed filter. Water Res., 47, 5400–5408.
- Huo, S.H., and Yan, X.P. (2012). Metal-organic framework MIL-100(Fe) for the adsorption of malachite green from aqueous solution. J. Mater. Chem., 22, 7449–7455.
- Hussain, S., Shaikh, S., and Farooqui, M. (2013). COD reduction of waste water streams of active pharmaceutical ingredient - atenolol manufacturing unit by advanced oxidation-Fenton process. J. Saudi Chem. Soc., 17, 199–202.
- Ikehata, K., and El-Din, M.G. (2006). Aqueous pesticide degradation by hydrogen peroxide/ultraviolet irradiation and Fenton-type advanced oxidation processes: a review. J. Environ. Eng. Sci., 5, 81–135.
- Ikehata, K., Naghashkar, N.J., and Ei-Din, M.G. (2006). Degradation of aqueous pharmaceuticals by ozonation and advanced oxidation processes: a review. Ozone: Sci. Eng., 28, 353–414.
- Isarain-Chavez, E., Arias, C., Cabot, P.L., Centellas, F., Rodriguez, R.M., Garrido, J.A., and Brillas, E. (2010). Mineralization of the drug beta-blocker atenolol by electro-Fenton and photoelectro-Fenton using an air-diffusion cathode for H2O2 electrogeneration combined with a carbon-felt cathode for Fe2+ regeneration. Appl. Catal. B: Environ., 96, 361–369.
- Jahn, M.K., Haderlein, S.B., and Meckenstock, R.U. (2005). Anaerobic degradation of benzene, toluene, ethylbenzene, and o-xylene in sediment-free iron-reducing enrichment cultures. Appl. Environ. Microbiol., 71, 3355–3358.
- Jain, A., Ong, S.P., Hautier, G., Chen, W., Richards, W.D., Dacek, S., Cholia, S., Gunter, D., Skinner, D., Ceder, G., and Persson, K.A. (2013). Commentary: The Materials Project: A materials genome approach to accelerating materials innovation. Appl. Mater., 1, 011002.
- Jamalluddin, N.A., and Abdullah, A.Z. (2011). Reactive dye degradation by combined Fe(III)/TiO2 catalyst and ultrasonic irradiation: Effect of Fe(III) loading and calcination temperature. Ultrason. Sonochem., 18, 669–678.
- Javier Benitez, F., Acero, J.L., Real, F.J., and Roldan, G. (2009). Ozonation of pharmaceutical compounds: rate constants and elimination in various water matrices. Chemosphere 77, 53–59.
- Jelić, A., Petrović, M., and Barceló, D. (2012). Pharmaceuticals in drinking water Emerging organic contaminants and human health (47–70). Berlin-Heidelberg: Springer.
- Ji, Y., Ferronato, C., Salvador, A., Yang, X., and Chovelon, J.M. (2014). Degradation of ciprofloxacin and sulfamethoxazole by ferrous-activated persulfate: implications for remediation of groundwater contaminated by antibiotics. Sci. Total Environ., 472, 800–808.
- Jia, Y., Aagaard, P., and Breedveld, G.D. (2007). Sorption of triazoles to soil and iron minerals. Chemosphere 67, 250–258.
- Jiang, J.Q. (2007). Research progress in the use of ferrate(VI) for the environmental remediation. J. Hazard Mater., 146, 617–623.
- Jiang, J.Q. (2014). Advances in the development and application of ferrate(VI) for water and wastewater treatment. J. Chem. Technol. Biotechnol., 89, 165–177.
- Jiang, J.Q., and Zhou, Z. (2013). Removal of Pharmaceutical residues by ferrate(VI). Plos One 8, e55729.
- Jiang, J.Q., Zhou, Z.W., Patibandla, S., and Shu, X.H. (2013). Pharmaceutical removal from wastewater by ferrate(VI) and preliminary effluent toxicity assessments by the zebrafish embryo model. Microchem. J., 110, 239–245.
- Kagle, J., Porter, A.W., Murdoch, R.W., Rivera-Cancel, G., and Hay, A.G. (2009). Chapter 3 Biodegradation of pharmaceutical and personal care products. Adv. Appl. Microbiol., 67, 65–108.
- Kallel, M., Belaid, C., Mechichi, T., Ksibi, M., and Elleuch, B. (2009). Removal of organic load and phenolic compounds from olive mill wastewater by Fenton oxidation with zero-valent iron. Chem. Eng. J., 150, 391–395.
- Keenan, C.R., and Sedlak, D.L. (2008). Ligand-enhanced reactive oxidant generation by nanoparticulate zero-valent iron and oxygen. Environ. Sci. Technol., 42, 6936–6941.
- Keller, A.A., Garner, K., Miller, R.J., and Lenihan, H.S. (2012). Toxicity of nano-zero valent iron to freshwater and marine organisms. Plos One 7, e43983.
- Khan, N.A., Hasan, Z., and Jhung, S.H. (2013). Adsorptive removal of hazardous materials using metal-organic frameworks (MOFs): a review. J. Hazard Mater., 244–245, 444–456.
- Kharisov, B.I., Dias, H.R., Kharissova, O.V., Jiménez-Pérez, V.M., Perez, B.O., and Flores, B.M. (2012). Iron-containing nanomaterials: synthesis, properties, and environmental applications. Rsc Adv., 2, 9325–9358.
- Khin, M.M., Nair, A.S., Babu, V.J., Murugan, R., and Ramakrishna, S. (2012). A review on nanomaterials for environmental remediation. Energy Environ. Sci., 5, 8075–8109.
- Klavarioti, M., Mantzavinos, D., and Kassinos, D. (2009). Removal of residual pharmaceuticals from aqueous systems by advanced oxidation processes. Environ. Int., 35, 402–417.
- Kochi, J.K. (1967). Mechanisms of organic oxidation and reduction by metal complexes. Science 155, 415–424.
- Komlos, J., Kukkadapu, R.K., Zachara, J.M., and Jaffe, P.R. (2007). Biostimulation of iron reduction and subsequent oxidation of sediment containing Fe-silicates and Fe-oxides: effect of redox cycling on Fe(III) bioreduction. Water Res., 41, 2996–3004.
- Kong, L.N., Wei, W., Zhao, Q.F., Wang, J.Q., and Wan, Y. (2012). Active coordinatively unsaturated manganese monoxide-containing mesoporous carbon catalyst in wet peroxide oxidation. ACS Catal., 2, 2577–2586.
- Kouraichi, R., Delgado, J.J., Lopez-Castro, J.D., Stitou, M., Rodriguez-Izquierdo, J.M., and Cauqui, M.A. (2010). Deactivation of Pt/MnOx-CeO2 catalysts for the catalytic wet oxidation of phenol: Formation of carbonaceous deposits and leaching of manganese. Catal. Today 154, 195–201.
- Kowalski, K.P., and Sogaard, E.G. (2014). Implementation of zero-valent iron (ZVI) into drinking water supply - role of the ZVI and biological processes. Chemosphere 117, 108–114.
- Kuan, W.H., Hu, C.Y., Liu, B.S., and Tzou, Y.M. (2013). Degradation of antibiotic amoxicillin using 1×1 molecular sieve-structured manganese oxide. Environmental Technology 34, 2443–2451.
- Kümmerer, K. (2004). Pharmaceuticals in the environment: Sources, fate, effects and risks; with 77 tables. Berlin-Heidelberg: Springer.
- Laine, D.F., Blumenfeld, A., and Cheng, I.F. (2008). Mechanistic study of the ZEA organic pollutant degradation system: evidence for H2O2, HO•, and the homogeneous activation of O2 by FeIIEDTA. Ind. Eng. Chem. Res., 47, 6502–6508.
- Langenhoff, A.A., Zehnder, A.J., and Schraa, G. (1996a). Behaviour of toluene, benzene and naphthalene under anaerobic conditions in sediment columns. Biodegradation 7, 267–274.
- Langenhoff, A.A.M., Zehnder, A.J.B., and Schraa, G. (1996b). Behaviour of toluene, benzene and naphthalene under anaerobic conditions in sediment columns. Biodegradation 7, 267–274.
- Lee, C., Kim, J.Y., Lee, W.I., Nelson, K.L., Yoon, J., and Sedlak, D.L. (2008). Bactericidal Effect of Zero-Valent Iron Nanoparticles on Escherichia coli. Environ. Sci. Technol., 42, 4927–4933.
- Lee, Y., Zimmermann, S.G., Kieu, A.T., and Von Gunten, U. (2009). Ferrate (Fe(VI)) application for municipal wastewater treatment: a novel process for simultaneous micropollutant oxidation and phosphate removal. Environ. Sci. Technol., 43, 3831–3838.
- Levec, J., and Pintar, A. (2007). Catalytic wet-air oxidation processes: a review. Catalysis Today 124, 172–184.
- Li, L., Fan, M.H., Brown, R.C., Van Leeuwen, J.H., Wang, J.J., Wang, W.H., Song, Y.H., and Zhang, P.Y. (2006). Synthesis, properties, and environmental applications of nanoscale iron-based materials: A review. Critical Reviews in Environ. Sci. Technol., 36, 405–431.
- Li, S.L., Wang, W., Liu, Y.Y., and Zhang, W.X. (2014). Zero-valent iron nanoparticles (nZVI) for the treatment of smelting wastewater: a pilot-scale demonstration. Chem. Eng. J., 254, 115–123.
- Li, W., Nanaboina, V., Zhou, Q., and Korshin, G.V. (2012). Effects of Fenton treatment on the properties of effluent organic matter and their relationships with the degradation of pharmaceuticals and personal care products. Water Res., 46, 403–412.
- Li, Y., Wei, D., and Du, Y. (2015a). Oxidative transformation of levofloxacin by δ-MnO2: Products, pathways and toxicity assessment. Chemosphere 119, 282–288.
- Li, Z.H., Fitzgerald, N.M., Albert, Z., Schnabl, A., and Jiang, W.T. (2015b). Contrasting mechanisms of metoprolol uptake on kaolinite and talc. Chem. Eng. J., 272, 48–57.
- Lima, D.R.S., Baeta, B.E.L., Aquino, S.F., Libanio, M., and Afonso, R.J.C.F. (2014). Removal of pharmaceuticals and endocrine disruptor compounds from natural waters by clarification associated with powdered activated carbon. Water Air Soil Pollut., 225, 2170.
- Lin, K., Liu, W., and Gant, J. (2009). Oxidative removal of bisphenol A by manganese dioxide: efficacy, products, and pathways. Environ. Sci. Technol., 43, 3860–3864.
- Liu, C., He, Y., Li, F., and Wang, H. (2013). Preparation of poly ferric sulfate and the application in micro-polluted raw water treatment. J. Chin. Adv. Mater. Soc., 1, 210–218.
- Lovley, D.R. (1991). Dissimilatory Fe(III) and Mn(IV) reduction. Microbiol. Rev., 55, 259–287.
- Lovley, D.R., and Phillips, E.J. (1988). Novel mode of microbial energy metabolism: organic carbon oxidation coupled to dissimilatory reduction of iron or manganese. Appl. Environ. Microbiol., 54, 1472–1480.
- Lovley, D.R., Holmes, D.E., and Nevin, K.P. (2004). Dissimilatory Fe(III) and Mn(IV) reduction. Adv. Microbial. Physiol., 49, 219–286.
- Lu, L., Li, J., Yu, J., Song, P., and Ng, D.H.L. (2016). A hierarchically porous MgFe2O4/γ-Fe2O3 magnetic microspheres for efficient removals of dye and pharmaceutical from water. Chem. Eng. J., 283, 524–534.
- Lv, A.H., Hu, C., Nie, Y.L., and Qu, J.H. (2010). Catalytic ozonation of toxic pollutants over magnetic cobalt and manganese co-doped gamma-Fe2O3. Appl. Catal. B: Environ., 100, 62–67.
- Lv, A.H., Hu, C., Nie, Y.L., and Qu, J.H. (2012). Catalytic ozonation of toxic pollutants over magnetic cobalt-doped Fe3O4 suspensions. Appl. Catal. B: Environ., 117, 246–252.
- Ma, J., Sui, M.H., Chen, Z.L., and Wang, L.N. (2004). Degradation of refractory organic pollutants by catalytic ozonation - activated carbon and Mn-loaded activated carbon as catalysts. Ozone: Sci. Eng., 26, 3–10.
- Mackulak, T., Mosny, M., Grabic, R., Golovko, O., Koba, O., and Birosova, L. (2015). Fenton-like reaction: a possible way to efficiently remove illicit drugs and pharmaceuticals from wastewater. Environ. Toxicol. Pharmacol., 39, 483–488.
- Madhavan, J., Kumar, P.S., Anandan, S., Zhou, M., Grieser, F., and Ashokkumar, M. (2010). Ultrasound assisted photocatalytic degradation of diclofenac in an aqueous environment. Chemosphere 80, 747–752.
- Mahmoodi, N.M. (2013). Manganese ferrite nanoparticle: Synthesis, characterization, and photocatalytic dye degradation ability. Desalin. Water Treat., 53, 84–90.
- Mailler, R., Gasperi, J., Coquet, Y., Deshayes, S., Zedek, S., Cren-Olive, C., Cartiser, N., Eudes, V., Bressy, A., Caupos, E., Moilleron, R., Chebbo, G., and Rocher, V. (2015). Study of a large scale powdered activated carbon pilot: Removals of a wide range of emerging and priority micropollutants from wastewater treatment plant effluents. Water Res., 72, 315–330.
- Marco-Urrea, E., Perez-Trujillo, M., Vicent, T., and Caminal, G. (2009). Ability of white-rot fungi to remove selected pharmaceuticals and identification of degradation products of ibuprofen by Trametes versicolor. Chemosphere 74, 765–772.
- Marco-Urrea, E., Radjenovic, J., Caminal, G., Petrovic, M., Vicent, T., and Barcelo, D. (2010). Oxidation of atenolol, propranolol, carbamazepine and clofibric acid by a biological Fenton-like system mediated by the white-rot fungus Trametes versicolor. Water Res., 44, 521–532.
- Martin, J., Camacho-Munoz, D., Santos, J.L., Aparicio, I., and Alonso, E. (2012). Occurrence of pharmaceutical compounds in wastewater and sludge from wastewater treatment plants: removal and ecotoxicological impact of wastewater discharges and sludge disposal. J. Hazard Mater., 239–240, 40–47.
- Martins, R.C., Cardoso, M., Dantas, R.F., Sans, C., Esplugas, S., and Quinta-Ferreira, R.M. (2015). Catalytic studies for the abatement of emerging contaminants by ozonation. J. Chem. Technol. Biotechnol., 90, 1611–1618.
- Matilainen, A., Vepsalainen, M., and Sillanpaa, M. (2010). Natural organic matter removal by coagulation during drinking water treatment: a review. Adv. Colloid Interface Sci., 159, 189–197.
- Meerburg, F., Hennebel, T., Vanhaecke, L., Verstraete, W., and Boon, N. (2012). Diclofenac and 2-anilinophenylacetate degradation by combined activity of biogenic manganese oxides and silver. Microbial. Biotechnol., 5, 388–395.
- Melero, J.A., Martinez, F., Botas, J.A., Molina, R., and Pariente, M.I. (2009). Heterogeneous catalytic wet peroxide oxidation systems for the treatment of an industrial pharmaceutical wastewater. Water Res., 43, 4010–4018.
- Meng, Y.T., Zheng, Y.M., Zhang, L.M., and He, J.Z. (2009). Biogenic Mn oxides for effective adsorption of Cd from aquatic environment. Environ. Pollut., 157, 2577–2583.
- Metcalf, L., Eddy, H.P., and Tchobanoglous, G. (2004). Wastewater engineering: Treatment, disposal, and reuse. New York [etc.]: McGraw-Hill.
- Michael, I., Hapeshi, E., Michael, C., Varela, A.R., Kyriakou, S., Manaia, C.M., and Fatta-Kassinos, D. (2012). Solar photo-Fenton process on the abatement of antibiotics at a pilot scale: Degradation kinetics, ecotoxicity and phytotoxicity assessment and removal of antibiotic resistant enterococci. Water Res., 46, 5621–5634.
- Monteiro, S.C., and Boxall, A.B. (2010). Occurrence and fate of human pharmaceuticals in the environment. Rev. Environ. Contam. Toxicol., 202, 53–154.
- Neta, P., Huie, R.E., and Ross, A.B. (1988). Rate constants for reactions of inorganic radicals in aqueous-solution. J. Phys. Chem. Ref. Data 17, 1027–1284.
- Neyens, E., and Baeyens, J. (2003). A review of classic Fenton's peroxidation as an advanced oxidation technique. J. Hazard Mater., 98, 33–50.
- Nfodzo, P., and Choi, H. (2011). Sulfate radicals destroy pharmaceuticals and personal care products. Environ. Eng. Sci., 28, 605–609.
- Noubactep, C. (2008a). A critical review on the process of contaminant removal in Fe0-H2O systems. Environ. Technol., 29, 909–920.
- Noubactep, C. (2008b). Comments on “Sorption of triazoles to soil and iron minerals”. by Y. Jia, et al. [ Chemosphere 67 (2007) 250–258]. Chemosphere 71, 802–806.
- Noubactep, C. (2010). The fundamental mechanism of aqueous contaminant removal by metallic iron. Water Sa 36, 663–670.
- Ogier, J. (2008). Fenton process for contaminant control: investigation of OH radical formation with two water types. Nieuwegein: KWR Watercycle Research Institute.
- Olmez-Hanci, T., Arslan-Alaton, I., and Dursun, D. (2014). Investigation of the toxicity of common oxidants used in advanced oxidation processes and their quenching agents. J. Hazard Mater., 278, 330–335.
- Olvera-Vargas, H., Oturan, N., Oturan, M.A., and Brillas, E. (2015). Electro-Fenton and solar photoelectro-Fenton treatments of the pharmaceutical ranitidine in pre-pilot flow plant scale. Sep. Purif. Technol., 146, 127–135.
- Onesios, K.M., Yu, J.T., and Bouwer, E.J. (2009). Biodegradation and removal of pharmaceuticals and personal care products in treatment systems: a review. Biodegradation 20, 441–466.
- Pantelidou, N.A., Theologides, C.P., Olympiou, G.G., Savva, P.G., Vasquez, M.I., and Costa, C.N. (2014). Catalytic removal of pharmaceutical compounds in water medium under an H2 stream over various metal-supported catalysts: a promising process. Desalin. Water Treat., 53, 3363–3370.
- Park, H.S., Koduru, J.R., Choo, K.H., and Lee, B. (2015). Activated carbons impregnated with iron oxide nanoparticles for enhanced removal of bisphenol A and natural organic matter. J. Hazard Mater., 286, 315–324.
- Phenrat, T., Long, T.C., Lowry, G.V., and Veronesi, B. (2009). Partial oxidation (“Aging”) and surface modification decrease the toxicity of nanosized zerovalent iron. Environ. Sci. Technol., 43, 195–200.
- Pignatello, J.J., Oliveros, E., and MacKay, A. (2006). Advanced oxidation processes for organic contaminant destruction based on the Fenton reaction and related chemistry. Crit. Rev. Environ. Sci. Technol., 36, 1–84.
- Pradyot, P. (2004). Preliminary separation methods Dean's Analytical Chemistry Handbook, Second Edition. McGraw Hill Professional, Access Engineering.
- Pruden, A., Pei, R.T., Storteboom, H., and Carlson, K.H. (2006). Antibiotic resistance genes as emerging contaminants: Studies in northern Colorado. Environ. Sci. Technol., 40, 7445–7450.
- Qin, F.-X., Jia, S.-Y., Liu, Y., Li, H.-Y., and Wu, S.-H. (2014). Adsorptive removal of bisphenol A from aqueous solution using metal-organic frameworks. Desalin. Water Treat., 1–10.
- Quinlivan, P.A., Li, L., and Knappe, D.R. (2005). Effects of activated carbon characteristics on the simultaneous adsorption of aqueous organic micropollutants and natural organic matter. Water Res., 39, 1663–1673.
- Rao, Y.F., Qu, L., Yang, H., and Chu, W. (2014). Degradation of carbamazepine by Fe(II)-activated persulfate process. J. Hazard Mater., 268, 23–32.
- Raychoudhury, T., and Scheytt, T. (2013). Potential of zerovalent iron nanoparticles for remediation of environmental organic contaminants in water: a review. Water Sci. Technol., 68, 1425–1439.
- Remucal, C.K., and Ginder-Vogel, M. (2014). A critical review of the reactivity of manganese oxides with organic contaminants. Environ. Sci. Process. Impacts 16, 1247–1266.
- Rey, A., Faraldos, M., Casas, J.A., Zazo, J.A., Bahamonde, A., and Rodriguez, J.J. (2009). Catalytic wet peroxide oxidation of phenol over Fe/AC catalysts: Influence of iron precursor and activated carbon surface. Appl. Catal. B: Environ., 86, 69–77.
- Rivera-Utrilla, J., Sanchez-Polo, M., Ferro-Garcia, M.A., Prados-Joya, G., and Ocampo-Perez, R. (2013). Pharmaceuticals as emerging contaminants and their removal from water. a review. Chemosphere 93, 1268–1287.
- Rodriguez-Alvarez, T., Rodil, R., Quintana, J.B., Trinanes, S., and Cela, R. (2013). Oxidation of non-steroidal anti-inflammatory drugs with aqueous permanganate. Water Res., 47, 3220–3230.
- Rosenberg, E., DeLong, E., Lory, S., Stackebrandt, E., Thompson, F., and Lovley, D. (2013). Dissimilatory Fe(III)- and Mn(IV)-reducing prokaryotes The Prokaryotes, 287–308. Springer Berlin Heidelberg.
- Ruamchat, T., Hayashi, R., Ngamprasertsith, S., and Oshima, Y. (2006). A novel on-site system for the treatment of pharmaceutical laboratory wastewater by supercritical water oxidation. Environ. Sci., 13, 297–304.
- Salas, E.C., Berelson, W.M., Hammond, D.E., Kampf, A.R., and Nealson, K.H. (2009). The influence of carbon source on the products of dissimilatory iron reduction. Geomicrobiol. J., 26, 451–462.
- Sanderson, H., Brain, R.A., Johnson, D.J., Wilson, C.J., and Solomon, K.R. (2004). Toxicity classification and evaluation of four pharmaceuticals classes: antibiotics, antineoplastics, cardiovascular, and sex hormones. Toxicology 203, 27–40.
- Santiago, A.F., Sousa, J.F., Guedes, R.C., Jeronimo, C.E., and Benachour, M. (2006). Kinetic and wet oxidation of phenol catalyzed by non-promoted and potassium-promoted manganese/cerium oxide. J. Hazard Mater., 138, 325–330.
- Santosa, I.J., Grossmana, M.J., Sartorattob, A., Ponezib, A.N., and Durranta, L.R. (2012). Degradation of the recalcitrant pharmaceuticals carbamazepine and 17α-ethinylestradiol by ligninolytic fungi. Chem. Eng., 27, 169–174.
- Segura, Y., Martinez, F., and Melero, J.A. (2013). Effective pharmaceutical wastewater degradation by Fenton oxidation with zero-valent iron. Appl. Catal. B: Environ., 136, 64–69.
- Segura, Y., Martinez, F., Melero, J.A., and Fierro, J.L.G. (2015). Zero valent iron (ZVI) mediated Fenton degradation of industrial wastewater: Treatment performance and characterization of final composites. Chem. Eng. J., 269, 298–305.
- Shah, N.S., He, X., Khan, H.M., Khan, J.A., O'Shea, K.E., Boccelli, D.L., and Dionysiou, D.D. (2013). Efficient removal of endosulfan from aqueous solution by UV-C/peroxides: a comparative study. J. Hazard Mater., 263 Pt 2, 584–592.
- Sharma, V.K., Kazama, F., Jiangyong, H., and Ray, A.K. (2005). Ferrates (iron(VI) and iron(V)): environmentally friendly oxidants and disinfectants. J. Water Health 3, 45–58.
- Shemer, H., Kunukcu, Y.K., and Linden, K.G. (2006). Degradation of the pharmaceutical metronidazole via UV, Fenton and photo-Fenton processes. Chemosphere 63, 269–276.
- Shirazi, E., Torabian, A., and Nabi-Bidhendi, G. (2013). Carbamazepine removal from groundwater: effectiveness of the TiO2/UV, nanoparticulate zero-valent iron, and Fenton (nZVI/H2O2) processes. Clean-Soil Air Water 41, 1062–1072.
- Singh, K.K., Senapati, K.K., Borgohain, C., and Sarma, K.C. (2015). Newly developed Fe3O4–Cr2O3 magnetic nanocomposite for photocatalytic decomposition of 4-chlorophenol in water. J. Environ. Sci. (China).
- Sires, I., Garrido, J.A., Rodriguez, R.M., Brillas, E., Oturan, N., and Oturan, M.A. (2007). Catalytic behavior of the Fe3+/Fe2+ system in the electro-Fenton degradation of the antimicrobial chlorophene. Appl. Catal. B Environ., 72, 382–394.
- Sousa, J.M., Macedo, G., Pedrosa, M., Becerra-Castro, C., Castro-Silva, S., Pereira, M.F., Silva, A.M., Nunes, O.C., and Manaia, C.M. (2016). Ozonation and UV radiation for the removal of microorganisms and antibiotic resistance genes from urban wastewater. J. Hazard Mater.(at press)
- Spasiano, D., Marotta, R., Di Somma, I., Andreozzi, R., and Caprio, V. (2013). Fe(III)-photocatalytic partial oxidation of benzyl alcohol to benzaldehyde under UV-solar simulated radiation. Photochem. Photobiol. Sci., 12, 1991–2000.
- Srinivasan, R., and Sorial, G.A. (2011). Treatment of taste and odor causing compounds 2-methyl isoborneol and geosmin in drinking water: A critical review. J. Environ. Sci. (China) 23, 1–13.
- Stumm, W., and Morgan, J.J. (2013). Aquatic chemistry: Chemical equilibria and rates in natural waters. Hoboken, NJ: Wiley-Interscience.
- Sui, M., Xing, S., Sheng, L., Huang, S., and Guo, H. (2012). Heterogeneous catalytic ozonation of ciprofloxacin in water with carbon nanotube supported manganese oxides as catalyst. J. Hazard Mater., 227–228, 227–236.
- Taylor, K.M., Rieter, W.J., and Lin, W. (2008). Manganese-based nanoscale metal-organic frameworks for magnetic resonance imaging. J. Am. Chem. Soc., 130, 14358–14359.
- Tebo, B.M., Bargar, J.R., Clement, B.G., Dick, G.J., Murray, K.J., Parker, D., Verity, R., and Webb, S.M. (2004). Biogenic manganese oxides: properties and mechanisms of formation. Ann. Rev. Earth Planet. Sci., 32, 287–328.
- Tekin, H., Bilkay, O., Ataberk, S.S., Balta, T.H., Ceribasi, I.H., Sanin, F.D., Dilek, F.B., and Yetis, U. (2006). Use of Fenton oxidation to improve the biodegradability of a pharmaceutical wastewater. J. Hazard Mater., 136, 258–265.
- Tiwari, D., and Lee, S.M. (2011). Ferrate(VI) in the treatment of wastewaters: a new generation green chemical. In F. S. García Einschlag (Eds.), Waste Water - Treatment and Reutilization (Chapter 12, pp. 241–276). InTech, Available from: http://www.intechopen.com/books/waste-watertreatment-and-reutilization/ferrate-vi-in-the-treatment-of-wastewaters-a-new-generation-green-chemical.
- Tixier, C., Singer, H.P., Oellers, S., and Muller, S.R. (2003). Occurrence and fate of carbamazepine, clofibric acid, diclofenac, ibuprofen, ketoprofen, and naproxen in surface waters. Environ. Sci. Technol., 37, 1061–1068.
- Tratnyek, P.G., and Johnson, R.L. (2006). Nanotechnologies for environmental cleanup. Nano Today 1, 44–48.
- Trovo, A.G., Nogueira, R.F., Aguera, A., Fernandez-Alba, A.R., Sirtori, C., and Malato, S. (2009). Degradation of sulfamethoxazole in water by solar photo-Fenton. Chemical and toxicological evaluation. Water Res., 43, 3922–3931.
- Tu, J., Yang, Z., Hu, C., and Qu, J. (2014). Characterization and reactivity of biogenic manganese oxides for ciprofloxacin oxidation. J. Environ. Sci. (China) 26, 1154–1161.
- Eurpoean Union (2013). Directive 2013/39/EU of the European Parliament and of the Council. Retrieved from Retrieved from http://eur-lex.europa.eu/LexUriServ/LexUriServ.do?uri=OJ:L:2013:226:0001:0017:EN:PDF
- US Environmental Protection Agency (2015). Contaminant Candidate List 3 - CCL 3. Retrieved from http://www2.epa.gov/ccl/contaminant-candidate-list-3-ccl-3#main-content
- Üzüm, Ç., Shahwan, T., Eroğlu, A.E., Hallam, K.R., Scott, T.B., and Lieberwirth, I. (2009). Synthesis and characterization of kaolinite-supported zero-valent iron nanoparticles and their application for the removal of aqueous Cu 2+ and Co 2+ ions. Appl. Clay Sci., 43, 172–181.
- Veloutsou, S., Bizani, E., and Fytianos, K. (2014). Photo-Fenton decomposition of beta-blockers atenolol and metoprolol; study and optimization of system parameters and identification of intermediates. Chemosphere 107, 180–186.
- Verma, A.K., Dash, R.R., and Bhunia, P. (2012). A review on chemical coagulation/flocculation technologies for removal of colour from textile wastewaters. J. Environ. Manage., 93, 154–168.
- Villacis-Garcia, M., Villalobos, M., and Gutierrez-Ruiz, M. (2015). Optimizing the use of natural and synthetic magnetites with very small amounts of coarse Fe(0) particles for reduction of aqueous Cr(VI). J. Hazard Mater., 281, 77–86.
- Villalobos, M., Sposito, G., and Bargar, J.R. (2004). Pb(II) reactivity on a biogenic Mn oxide: Evidence of inner-sphere bonding at internal and external sites. Abstr. Pap. Am. Chem. Soc., 227, U1217–U1217.
- Villatoro-Monzon, W.R., Mesta-Howard, A.M., and Razo-Flores, E. (2003). Anaerobic biodegradation of BTEX using Mn(IV) and Fe(III) as alternative electron acceptors. Water Sci. Technol., 48, 125–131.
- Wan, J., Deng, H.P., Shi, J., Zhou, L., and Su, T. (2014). Synthesized magnetic manganese ferrite nanoparticles on activated carbon for sulfamethoxazole removal. Clean - Soil, Air, Water 42, 1199–1207.
- Wang, C.C., Li, J.R., Lv, X.L., Zhang, Y.Q., and Guo, G.S. (2014). Photocatalytic organic pollutants degradation in metal-organic frameworks. Energy Environ. Sci., 7, 2831–2867.
- Wang, H., Yao, H., Sun, P., Pei, J., Li, D., and Huang, C.H. (2015). Oxidation of tetracycline antibiotics induced by Fe(III) ions without light irradiation. Chemosphere 119, 1255–1261.
- Wang, J., Sun, W., Zhang, Z., Jiang, Z., Wang, X., Xu, R., Li, R., and Zhang, X. (2008). Preparation of Fe-doped mixed crystal TiO2 catalyst and investigation of its sonocatalytic activity during degradation of azo fuchsine under ultrasonic irradiation. J. Colloid Interface Sci., 320, 202–209.
- Wang, L., Hung, Y.-T., Shammas, N., Zou, L., Li, Y., and Hung, Y.-T. (2007). Wet Air Oxidation for Waste Treatment Advanced Physicochemical Treatment Technologies (Handbook of Environmental Engineering, Ch. 13, 575–610). Totowa, NJ: Humana Press.
- Wang, P., Shi, Q., Shi, Y., Clark, K.K., Stucky, G.D., and Keller, A.A. (2009). Magnetic permanently confined micelle arrays for treating hydrophobic organic compound contamination. J. Am. Chem. Soc., 131, 182–188.
- Wang, S., and Wang, X. (2015). Multifunctional metal-organic frameworks for photocatalysis. Small 11, 3097–3112.
- Wang, Y.Q., Cheng, R.M., Wen, Z., and Zhao, L.J. (2011). Synthesis and characterization of single-crystalline MnFe2O4 ferrite nanocrystals and their possible application in water treatment. Eur. J. Inorganic Chem., 2011, 2942–2947.
- Watts, R.J., Sarasa, J., Loge, F.J., and Teel, A.L. (2005). Oxidative and reductive pathways in manganese-catalyzed Fenton's reactions. J. Environ. Eng., 131, 158–164.
- Wei, H., Hu, D., Su, J., and Li, K.B. (2015). Intensification of levofloxacin sono-degradation in a US/H2O2 system with Fe3O4 magnetic nanoparticles. Chin. J. Chem. Eng., 23, 296–302.
- Wen, X., Jia, Y., and Li, J. (2010). Enzymatic degradation of tetracycline and oxytetracycline by crude manganese peroxidase prepared from Phanerochaete chrysosporium. J. Hazard Mater., 177, 924–928.
- Wilde, M.L., Mahmoud, W.M., Kummerer, K., and Martins, A.F. (2013). Oxidation-coagulation of beta-blockers by K2FeVIO4 in hospital wastewater: assessment of degradation products and biodegradability. Sci. Total Environ., 452–453, 137–147.
- World Health Organization (2012). Pharmaceuticals in drinking-water. Switzerland: WHO Press.
- Xia, S., Gu, Z., Zhang, Z., Zhang, J., and Hermanowicz, S.W. (2014). Removal of chloramphenicol from aqueous solution by nanoscale zero-valent iron particles. Chem. Eng. J., 257, 98–104.
- Xing, Z.P., and Sun, D.Z. (2009). Treatment of antibiotic fermentation wastewater by combined polyferric sulfate coagulation, Fenton and sedimentation process. J. Hazard Mater., 168, 1264–1268.
- Yan, J., Lei, M., Zhu, L., Anjum, M.N., Zou, J., and Tang, H. (2011). Degradation of sulfamonomethoxine with Fe3O4 magnetic nanoparticles as heterogeneous activator of persulfate. J. Hazard Mater., 186, 1398–1404.
- Yan, Q., Gao, X., Chen, Y.P., Peng, X.Y., Zhang, Y.X., Gan, X.M., Zi, C.F., and Guo, J.S. (2014). Occurrence, fate and ecotoxicological assessment of pharmaceutically active compounds in wastewater and sludge from wastewater treatment plants in Chongqing, the Three Gorges Reservoir Area. Sci. Total Environ., 470–471, 618–630.
- Yang, B., Ying, G.G., Zhao, J.L., Liu, S., Zhou, L.J., and Chen, F. (2012). Removal of selected endocrine disrupting chemicals (EDCs) and pharmaceuticals and personal care products (PPCPs) during ferrate(VI) treatment of secondary wastewater effluents. Water Res., 46, 2194–2204.
- Yang, L., Hu, C., Nie, Y., and Qu, J. (2009). Catalytic ozonation of selected pharmaceuticals over mesoporous alumina-supported manganese oxide. Environ. Sci. Technol., 43, 2525–2529.
- Yao, Y., Cai, Y., Lu, F., Wei, F., Wang, X., and Wang, S. (2014). Magnetic recoverable MnFe2O4 and MnFe2O4-graphene hybrid as heterogeneous catalysts of peroxymonosulfate activation for efficient degradation of aqueous organic pollutants. J. Hazard Mater., 270, 61–70.
- Zelmanov, G., and Semiat, R. (2008). Iron(3) oxide-based nanoparticles as catalysts in advanced organic aqueous oxidation. Water Res., 42, 492–498.
- Zelmanov, G., and Semiat, R. (2009). Iron (2,3) oxides based nano-particles as catalysts in advanced organic aqueous oxidation. Desalin. Water Treat., 6, 190–191.
- Zhan, W., Wang, X., Li, D., Ren, Y., Liu, D., and Kang, J. (2013). Catalytic wet air oxidation of high concentration pharmaceutical wastewater. Water Sci. Technol., 67, 2281–2286.
- Zhang, H., and Huang, C.H. (2003). Oxidative transformation of triclosan and chlorophene by manganese oxides. Environ. Sci. Technol., 37, 2421–2430.
- Zhang, H., and Huang, C.H. (2005). Oxidative transformation of fluoroquinolone antibacterial agents and structurally related amines by manganese oxide. Environ. Sci. Technol., 39, 4474–4483.
- Zhang, H., and Huang, C.H. (2007). Adsorption and oxidation of fluoroquinolone antibacterial agents and structurally related amines with goethite. Chemosphere 66, 1502–1512.
- Zhang, H., Chen, W.R., and Huang, C.H. (2008). Kinetic modeling of oxidation of antibacterial agents by manganese oxide. Environ. Sci. Technol., 42, 5548–5554.
- Zhang, L., Tu, J., Lyu, L., and Hu, C. (2016). Enhanced catalytic degradation of ciprofloxacin over Ce-doped OMS-2 microspheres. Appl. Catal. B: Environ., 181, 561–569.
- Zhang, Y., Yang, Y., Zhang, Y., Zhang, T., and Ye, M. (2012). Heterogeneous oxidation of naproxen in the presence of α-MnO 2 nanostructures with different morphologies. Appl. Catal. B: Environ., 127, 182–189.
- Zhao, H., Cui, H.-J., and Fu, M.-L. (2014). Synthesis of core–shell structured Fe3O4@α-MnO2microspheres for efficient catalytic degradation of ciprofloxacin. Rsc Adv., 4, 39472.
- Zheng, Y. (2011). Pretreatment of pharmaceutical wastewater by catalytic wet air oxidation(CWAO) C3 - ISWREP 2011 - Proceedings of 2011 International Symposium on Water Resource and Environmental Protection, 1316–1318.
- Zhou, M., Yu, J., and Cheng, B. (2006). Effects of Fe-doping on the photocatalytic activity of mesoporous TiO2 powders prepared by an ultrasonic method. J. Hazard Mater., 137, 1838–1847.
- Zhou, T., Lim, T.T., Li, Y., Lu, X., and Wong, F.S. (2010). The role and fate of EDTA in ultrasound-enhanced zero-valent iron/air system. Chemosphere 78, 576–582.
- Zhou, Y., Gao, B., Zimmerman, A.R., Chen, H., Zhang, M., and Cao, X. (2014). Biochar-supported zerovalent iron for removal of various contaminants from aqueous solutions. Bioresour. Technol., 152, 538–542.
- Zouboulis, A.I., Moussas, P.A., and Vasilakou, F. (2008). Polyferric sulphate: preparation, characterisation and application in coagulation experiments. J. Hazard Mater., 155, 459–468.