ABSTRACT
Resource scarcity, hazardous waste, and climate change are the driving forces for developing energy efficient and low-toxic lighting sources. Currently, solid state lighting based on light-emitting diodes is expected to become the most dominant lighting technology of the future, mostly driven by its advantage with respect to energy efficiency. Parallel to the ongoing development of light-emitting diode-based lighting sources, a number of single case and comparative life cycle assessment studies of LED-lamps and components in varying study settings were carried out. However, these studies mostly rely to specific lamp designs, which limits general conclusions. This work includes a summary of the global market of lighting and LED-lamp technologies, followed by a comprehensive review and comparison of published life cycle assessment studies. In addition, we take also some aspects, which are relevant for the well-being of an end-user and which are usually not treated in life cycle assessment studies, like glare and health issues, into consideration. A critical interpretation of the assumptions and outcomes of these studies is given and questions that remain unsettled and therefore should be addressed in future studies are discussed.
Abbreviations | ||
ADP | = | abiotic depletion potential |
AiP | = | air pollution |
AP | = | acidification potential |
ARD | = | abiotic resource depletion |
av. | = | average |
Bx | = | LED failure rate |
CC | = | climate change |
CED | = | cumulative energy demand |
CFL | = | compact fluorescent lamp |
CMH | = | ceramic metal halide (lamp) |
CML | = | Centre of Environmental Science, University of Leiden, the Netherlands |
CO2e / CO2 eq. | = | carbon dioxide equivalent |
CQS | = | color quality scale |
CRI | = | color rendering index |
Ded-LED | = | dedicated LED |
EDP | = | ecosystem damage potential |
EI 99 | = | eco-indicator 99 |
ELC | = | European Lamp Companies Federation |
El. demand | = | electricity demand |
EP | = | eutrophication potential |
ESU | = | energy – materials – environment |
ETH | = | Swiss Federal Institute of Technology |
ETX | = | ecotoxicity |
EU | = | European Union |
EUR | = | Euro |
EUR m | = | million Euro |
e-waste | = | electronic waste |
FAETP | = | freshwater aquatic ecotoxicity potential |
FEP | = | freshwater eutrophication potential |
FETP | = | freshwater eco-toxicity potential |
FL / FLL | = | fluorescent lamp |
FSET | = | freshwater sediment ecotoxicity |
GaBi | = | Ganzheitliche Bilanzierung (life cycle engineering) |
GHG | = | greenhouse gas |
GLS | = | general lighting service |
GWP | = | global warming potential |
HID | = | high-intensity discharge (lamp) |
HPS | = | high-pressure sodium (lamp) |
HR | = | hours |
HT/HTP | = | human toxicity potential |
HTPce | = | human toxicity with cancer effects |
HTPnce | = | human toxicity with noncancer effects |
HW | = | hazardous waste |
HWL | = | hazardous waste landfilled |
ICL | = | incandescent lamp |
IDEMAT | = | Industrial Design & Engineering MATerials (database) |
IEA | = | International Energy Agency |
ILCD | = | International Life Cycle Data |
IMCOA | = | Industrial Minerals Company of Australia |
INC | = | incandescent lamp |
int-LED | = | integrated LED |
IR | = | ionizing radiation |
ISO | = | International Organization for Standardization |
IW | = | inert waste |
JRC | = | Joint Research Centre |
LCA | = | life cycle assessment |
LCIA | = | life cycle impact assessment |
LED | = | light emitting diode |
LFL | = | linear fluorescent lamp |
LU | = | land use |
LUP | = | land use potential |
Lx | = | luminous flux depreciation |
MA | = | maladors air |
MAET | = | marine aquatic ecotoxicity |
MAETP | = | marine aquatic ecotoxicity potential |
MEP | = | marine eutrophication potential |
MH | = | metal halide (lamp) |
MOCVD | = | metalorganic chemical vapor deposition |
mod. | = | modified |
MOVPE | = | metal organic chemical vapor phase epitaxy |
MSET | = | marine sediment ecotoxicity |
NERC | = | North American Electric Reliability Corp. |
NHW | = | nonhazardous waste |
NHWL | = | nonhazardous waste landfilled |
NIST | = | National Institute of Standards and Technology |
NRE | = | nonrenewable energy |
OD | = | stratospheric O3 depletion |
ODP | = | ozone depleting potential |
OLED | = | organic light emitting diode |
pcs | = | pieces |
PE | = | primary energy |
PEG | = | polyethylene glycol |
PiG | = | phosphor in glass |
PMMA | = | polymethyl methacrylate |
POCP | = | photochemical ozone creation potential |
POP | = | photochemical oxidation potential |
PV | = | photovoltaic |
PVC | = | polyvinylchloride |
R&D | = | research & development |
RE | = | renewable energy |
RE | = | rare earth |
REO | = | rare earth oxide |
RESP | = | respiratory effects |
RFC | = | Reliability First Corporation (US) |
RoHS | = | restriction of hazardous substances |
RW | = | radioactive waste |
RWL | = | radioactive waste landfilled |
SMD | = | surface-mounted device |
SSL | = | solid state lighting |
TAETP | = | terrestrial ecotoxicity potential |
TEP | = | terrestrial eutrophication potential |
TET | = | terrestrial ecotoxicity |
TRACI | = | Tool for the Reduction and Assessment of Chemical and other Environmental Impacts |
TV | = | television |
U.S. I/O | = | USA input/output database |
UCTE | = | Union for the Co-ordination of Transmission of Electricity |
UK | = | United Kingdom |
US | = | United States (of America) |
US DOE | = | United States Department of Energy |
USLCI | = | U.S. life cycle inventory (database) |
UV | = | ultraviolet |
WaC | = | water consumption |
WaP | = | water pollution |
WEEE | = | waste electrical and electronic equipment |
wt% | = | weight percent |
YAG | = | yttrium aluminium garnet |
Introduction
High-power LED-lamps have been on the market for more than 10 years. Estimates of the economic global market share of LED-lamps in the general lighting sector vary between 31% (LEDinside, Citation2014) and 45% (McKinsey and Company, 2012) for the period 2015/2016. Regardless of these significant values, it should be kept in mind that there are still technical problems concerning the long-term reliability of LED-lamps, such as thermal overload, irreversible color shift, decrease of efficacy, and other material fatigue problems (Chang et al., Citation2012; Fulmek et al., Citation2016; Khanna, Citation2014; Mehr et al., Citation2015)
In the European Union (EU), the gradual replacement of incandescent lamps by compact fluorescent lamps (CFL) and by LED-lamps was enforced by the EU household lamp regulation (Comm. Reg., Citation2009). This regulation is based on the EU Ecodesign Directive (Directive/EU, 2009) with the aim to reduce electricity consumption by energy efficient lamp technologies. The comparison of the luminous efficacies of incandescent lamps (10–20 lm/W) and high-power LED-lamps (> 60 lm/W) obviously shows the theoretical energy saving effect in the using phase. This was confirmed over the full life cycle in a number of life cycle assessment (LCA) studies for these lamp types (IEA, Citation2014; Navigant, Citation2009; Osram, Citation2009).
In the literature there are various data on the global electricity consumption of lighting. The International Energy Agency estimates that the global electricity consumption in 2030 will be 5000 TWh under no energy-efficiency policies, 4250 TWh under moderate policies, and 2618 TWh under least life-cycle cost policies (IEA, Citation2006). A McKinsey study reports that the global lighting market consumes approximately 20% of the total electric power generated (McKinsey and Company, 2012). The United Nations supported ‘‘en.lighting initiative” reports a share of 15% in 2010 (En.lighten, Citation2016). In a study on the potentials and challenges of Solid State Lighting for Europe, De Almeida et al. report on a 19% share of lighting on the global electricity consumption, while for the European Union this value is a bit lower (14%) (De Almeida et al., Citation2014). A Central Europe case study of Austria states a national value of 15% in the not differentiated category “lighting & IT.” Considering only households, the lighting electricity consumption was 3% of the country's total electricity consumption in 2012 (Franz and Nicolics, Citation2015). Nevertheless, the mentioned case study on power consumption in Austria showed that in the same period of the lighting technology transformation, electricity consumption of all household lamps did not decrease but increased by 29%. The British daily newspaper ‘‘The Guardian” reports that due to legally permitted tolerance values of up to 10% in the mandatory production information of the lamps, the declared values for luminosity and power consumption may be responsible for wrong consumption rates of up to 25% (Comm. Reg., Citation2009; Neslen, Citation2015).
Based on the energy-saving background, a number of life cycle assessment studies on lighting have been performed by manufacturing companies, consulting companies, and scientific researchers. These studies were carried out with different focuses on comparison objects, assessment methods, assumptions, and data transparency.
This work aims to provide a holistic view and review of the global environmental situation of LED-lamps for general lighting. The position and applications of LED-lamps in the global market of lighting is emphasized. Details of materials, technologies, and waste management considerations are presented. Based on this information, a comprehensive comparison of published life cycle assessment studies for LED-lamps, their assumptions on materials, manufacturing, power consumption, comparative data, and results is performed. LED-lighting with its technological and application-oriented complexity is elaborated and its reflection in existing life cycle assessment studies is investigated. Environmental aspects outside the scope of a life cycle assessment such as glaring, health effects on human and animals, and open research challenges are discussed.
Materials and methods
This review is based on three pillars. First, the actual global market of LED-lamps and their advantages with respect to energy efficiency are discussed in comparison with other lighting technologies. Second, a comprehensive summary of environmentally relevant parameters and aspects of different LED-lamp technologies, materials, and waste management considerations is presented. Third, most of the available life cycle assessment studies for LED-lamps are reviewed and compared in detail. This is followed by a detailed discussion including also aspects beyond energy efficiency, like potential health risks due to blue light exposure. More than 100 studies and other literature from the period 2007 to 2016 were examined.
Global market of lighting
The global lighting market is divided into three main sectors: general lighting (79%), automotive lighting (20%), and backlighting (1%), with an estimated total producer price volume of 91 billion Euro in 2016. In this review only general lighting data are considered.
All data presented in this section are based on the trends for 2016 from the comprehensive global lighting report by McKinsey and Company (2012). It is important to note that the values of refer to the producer price of new manufactured lamps for new installations or replacements in a specific year. They do not provide information about the installed nominal power and the number of existing lamps.
Figure 1. Global economic general lighting market: New installations and replacements 2016 [million EUR].
![Figure 1. Global economic general lighting market: New installations and replacements 2016 [million EUR].](/cms/asset/135d5adc-0887-4377-9329-869ce7c7e573/best_a_1370989_f0001_b.gif)
Figure 2. Global economic share for different application segments of the general lighting market 2016.

Figure 3. Global economic share for different application segments of the general lighting market for different regions of the world, 2016 [million EUR].
![Figure 3. Global economic share for different application segments of the general lighting market for different regions of the world, 2016 [million EUR].](/cms/asset/1285d7e3-48dc-4cca-a2ca-e3931bc3a0c0/best_a_1370989_f0003_b.gif)
Figure 4. Global newly installed or replaced light sources by technology in 2016 per unit share (left) and per economic share (right).
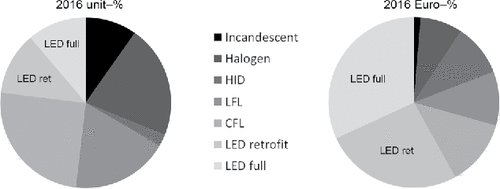
The general lighting sector can be divided into seven application categories. shows the producer price market share in 2016, depending on the application category and the share of LED-lamps and other lamp technologies in each category. The market of lighting control systems is included with an average share of 5.6% of the general lighting market and a growth rate anticipated at almost 20% per year.
With a value of almost 40%, the residential sector has the largest share of new installations and replacements. Based on , shows the percentage shares of different application segments of general lighting.
From a territorial point of view, Asia holds 42% of the global lighting market share, followed by Europe and North America. Russia has a market share of 8% of the European lighting market in 2016. compares the global lighting market for different regions of the world.
From these figures, it could be expected that the residential sector has the highest number of installed fixtures, but these values mainly seem to represent the high replacement rate in this segment. Because of the shorter lifetime of the used lamp types and technologies, it can be expected that the replacement rates of residential lamps are higher than in other application segments. Apart from that, the daily operation time of residential lighting might be shorter than for the other segments. Therefore, from the statistical data of and data on power and material consumptions, which are key factors for life cycle assessments, cannot be directly derived.
In total, the number of globally installed light sources for general lighting amounts to 32 billion units. The McKinsey lighting study estimates that 4.3 billion of these were to be newly installed or replaced in 2016. Thereof, 37% are LED light sources and 22% of these are retrofit LED-lamps. shows the shares of newly installed and replaced light sources in 2016 with respect to different lighting technologies. The McKinsey report covers the rare case that the global market situation is given both in economic conditions as well as in unit quantities, which makes it more meaningful for environmental assessments. The average conversion values per light source for the year 2016 are:
The values represent the producer price of the base light source without driver, fixture, and control systems. In absolute numbers, the general lighting market in 2016 consisted of new installations on the lamp/module level (8078 EUR m), the driver level (7323 EUR m), the fixture level (43,572 EUR m), the lighting system control level (4051 EUR m), and the light source replacement market (8888 EUR m). For comparison how statistics change between unit-% and Euro-% a second diagram is shown in with the same market situation in 2016 but displayed as economic share.
The different representations of the diagrams show that LED-lamps have a 23% market share from the point of view of units and 58% from the economic point of view. This shows the essential difference of these representations for resource and e-waste material and mass estimations.
Technologies, materials, and waste management of LED-lamps
Functional principle of LED-lamps
LED-dice are based on semiconductor technology (Dupuis and Krames, Citation2008; Nakamura, Citation2015). Semiconductors are solid state materials characterized by a small band gap (the energy difference between isolating and conducting states). With the help of so-called dopants, the activation energy and the charge carrier density can be controlled over a wide range.
Light-emitting diodes are made from two adjacent semiconductor layers. One layer has a surplus of electrons (n-layer) and the other layer has a shortage of electrons (p-layer). If a voltage is applied, electrons of the n-layer can diffuse to the p-layer, where they recombine with the holes. The related energy is released in form of visible light. The wavelength of the emitted light depends on the materials composition of the semiconductor layers.
Today the highest efficiencies, which is the photonic power divided by the applied electric power, are achieved with blue-light and red-light-emitting LEDs. To generate white light, either several LEDs with different emission wavelengths can be combined or a (UV or blue-light)-emitting LED can be coated with a color converter, typically a phosphor in a transparent matrix. Some part of the blue or UV radiation excites the phosphor particles, which again emit red/yellow light. The combination of these wavelengths results in a broadband spectral power distribution with a whitish impression. The spectrum can be tailored by the materials (phosphor) composition of the color converter.
Technologies and classifications of LED-lamps
The light source of an LED-lamp consists of one or more semiconductor LED-dice, which are assembled to a single or multi-chip (array) LED module. These modules are integrated into lamps, which are again connected to an electronic ballast and a heat sink. The design of the lamp has large influence on the technical performance of the lamp. For instance, so-called retrofit lamps have less favorable technical parameters in terms of thermal management and therefore lifetime, but they can fit into existing luminaires and sockets.
A new design for LED retrofit lamps is LED “filament” light bulbs (Patent, Citation2014). Here, LEDs are placed in series on a transparent substrate and are covered by a phosphor layer, mimicking a filament of an incandescent lamp. The lamp has no aluminium heat sink. The heat is dissipated via the surrounding gas and the glass bulb. These lamps are reported to have a high luminous efficacy of up to 130 lm/W at a color temperature of 2700 K, but the lifetime is limited to about 15,000 hr or less.
White light LED-packaging technologies
As mentioned, white light can be generated in different ways: combination of monochromatic LEDs with different colors (color mixing) or activation of the phosphor of a color conversion layer by blue or UV LEDs (phosphor-conversion). More sophisticated systems use several monochromatic LEDs and/or several phosphors. shows the most important technologies for LED-based white light generation (Kölper et al., Citation2011). The color conversion layer can be directly applied on the LED dice, see , or in some distance to the LED dice (remote phosphor, see ).
Figure 5. Typical white light LED generation technologies (Kölper et al., Citation2011).
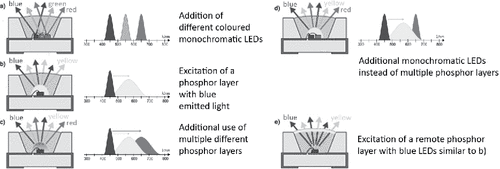
The transmission of blue light through a color converter is about 20% for LED-lamps with a color temperature of 3000 K and 30% for 4000 K (Hecht, Citation2016).
Classification and applications of LEDs
Depending on the application, LED-packages are classified into four different power ranges with different chip-luminous fluxes and package sizes (Navigant, Citation2012):
▪ | Low-power LED: SMD-package; < 100 mW; 4–15 lm; mobile phones, displays, signs | ||||
▪ | Mid-power LED: SMD-package; < 500 mW; 12–65 lm; TV backlight, automobile headlights, large displays, general lighting | ||||
▪ | High-power LED: power-package; 1–3 W; 70–120 lm; automobile headlights, projection, general lighting | ||||
▪ | LED-multichip arrays: power-package arrays; 1–3 W per LED; < 6000 lm; automobile headlights, projection, general lighting |
LED-lamps for general lighting can be classified into two different types:
▪ | Retrofit LED-lamps: replacement of incandescent lamps with conventional standard threads or plug connections with traditional bulb or spot design by LED technology; in a strict sense, LED-lamps in form of a linear fluorescent lamps are also retrofit lamps, but these are out of the scope of this work | ||||
▪ | Integrated/dedicated/full LED-lamps with free design opportunities; the housing is designed for optimal thermal management and is permanently attached to the LED-array |
Materials, rare earths, and strategic metals of LED-lamps
A LED-lamp consists of (a) the LED-array, (b) the electronic ballast, (c) the heat sink, (d) an optical element, and (e) the housing. shows typical materials that are commonly used (Navigant, Citation2012; Wilburn, Citation2012) in this regard. Materials and masses vary in a wide range, depending on the lamp design. Exemplarily, the total mass of retrofit LED-lamps varies between 83 g and 290 g (Navigant, Citation2012, Part 1). Integrated LED-lamps such as ceiling lamps have a mass between 520 g and 1.75 kg (Navigant, Citation2009; Principi and Fioretti, Citation2014; Tähkämö et al., Citation2013). Desk and floor lamps can weigh several kilograms. Streetlamps have a mass between 12 kg and 15 kg (Abdul Hadi et al., Citation2013; Tähkämö and Halonen, Citation2015).
Table 1. Components and materials of LED-lamps.
LED semiconductor materials
The material composition of a LED-die determines the color of the emitted monochromatic light. shows a selection of semiconductor compounds used for high brightness LEDs for different colors (Cangeloso, Citation2012; Khanna, Citation2014; Navigant, Citation2012). The blue light emitting LED-die consists of a substrate layer typically made of sapphire (Al2O3), silicon, or silicon carbide. The aluminium oxide is processed to high-purity of 99.9999%. Then, the semiconductor materials, such as alloys of GaAs, GaAsP, GaN, InGaN, GaP, SiC, are deposited on the substrate (Wilburn, Citation2012). Exemplarily, the amounts needed for 1 mm2 semiconductor material of an InGaN LED-die are 0.17 mg indium and 0.53 mg gallium. The mass calculation is based on seven pieces of 5-inch wafers (Fraunhofer/IZT, Angerer et al., Citation2009; Grandell et al., Citation2016).
LED color converter materials
The color conversion layer seems to be less frequently the subject in life cycle assessment studies. Therefore, more emphasis on this and the various possible material compositions will be put on this in this study.
The material composition of the color converter determines efficacy (Sommer et al., Citation2011), color rendering, and the color temperature, which can vary from “warm white” to “cold white.” The thermal conductivity of the matrix material is essential in order to keep the temperature of the color converter at a moderate level in spite of the unavoidable optical power losses within the converter.
The color conversion layer consists of a transparent matrix material, typically silicone, and embedded phosphor particles as well as filler particles. More recent color converter technologies use ceramics (Raukas et al., Citation2013) or glass-ceramics to overcome the problems of ageing and reliability (Bae et al., Citation2013). shows principle technologies and material compositions of color conversion layers (Cangeloso, Citation2012; Chang et al., Citation2016; Dong et al., Citation2015; George et al., Citation2013; Jüstel, Citation2013; Khanna, Citation2014; Li et al., Citation2016; McKittrick et al., Citation2013; McKittrick and Shea-Rohwer, Citation2014; Park et al., Citation2015; Patent, Citation2010; Tang et al., Citation2015; Wang et al., Citation2015; Ye et al., Citation2010; Yi et al., Citation2015). The purposes of filler particles like titanium dioxide are to increase the refractive index (Mont et al., Citation2008; Zheng et al., Citation2015) and the thermal conductivity of the entire matrix (Chung et al., Citation2012).
The phosphor content for a single retrofit LED-lamp with remote phosphor is approximately 1 g (Navigant, Citation2012). Exemplarily, 1g YAG:Ce remote phosphor includes 0.7 mg cerium and 450 mg yttrium (Grandell et al., Citation2016). For comparison, a CFL bulb contains about 0.9 g rare earths and FL lamps even 2.4 mg per cm2 (Pavel et al., Citation2016). The rare earths used in phosphors must be 99.999% pure, which means that LED phosphor materials require more processing and purification steps than phosphors for other applications (Wilburn, Citation2012).
Rare earths and strategic metals in LEDs
Rare earths and strategic metals are key elements of LED-lamps. They are included in very small quantities as high-tech (nano)materials in LED-dice and color converters. In existing life cycle assessment studies, these materials are neglected or inventoried in a general form. The variety of material compositions and manufacturing are not reflected in life cycle assessment studies.
Rare earth elements belong to the lanthanide group in the periodic table. They can be found in very small quantities as minerals or admixture to other minerals, which are geographically widely dispersed. Therefore, the main share in industrial exploitation of rare earths is obtained as by-product in the chemical processing of other metals from ores. It is estimated that China supplied about 64% of the global required rare earths in 2016 (ERECON, Citation2015).
Strategic metals are limited resources and therefore subject to financial speculations. shows a listing of rare earths and strategic metals, broken down according to their use in LED-components, depending on technology.
Table 2. Rare earths and strategic metals used in LEDs.
A study by Grandell et al. evaluated the market situation of metals which are crucial for green energy technologies by the year 2050. Besides silver, indium and lanthanum are the most critical materials for lighting technologies in the future (Grandell et al., Citation2016). Pavel et al. found eight highly critical elements for lighting applications based on market and geopolitical factors (Dy, Eu, Tb, Y, Pr, Nd, Ga, Te) and six of medium-to-high risk (graphite, Re, Hf, Ge, Pt, In) (Pavel et al., Citation2016).
shows the deposits of rare earths in the earth crust in ppm (Gunn, Citation2014). Comparing the color converter doping materials, it shows that from the resource point of view, europium, terbium, holmium, and thulium are limited materials. A further limited element is lutetium, which is used in carrier crystals.
The global demand and supply forecast of rare earth oxides for 2014 in tonnes (± 15%) is shown in . The total amount of global rare earth oxides consumption in 2012 was 189.000 tonnes.
Table 3. Demand forecasting of rare earth oxides for the year 2014 (Von Nauckhoff, Citation2011).
The global demand of the heavy rare earths terbium, yttrium, and of the light rare earth praseodymium is higher than the supply. This fact leads to a critical supply situation in the future. Due to the fact that the mining in other deposits are noneconomic and coupled with a high environmental impact this problem is unsolved yet. According to , the forecasted demand for europium can sufficiently be covered. Different results were found by Kingsnorth, who points out that in 2016 the demand for europium oxide will be 625–725 tonnes and the supply will be 450–550 tonnes (Kingsnorth, Citation2012).
Potential applications of rare earths and their respective proportions for use in a diversity of products are shown in (Binnemans et al., Citation2013). Europium is exclusively used for phosphors.
Table 4. Application of rare earths in [%] (Binnemans et al., Citation2013).
Wilburn forecasted for 2014 a rare earth use of 3.3 t Ce, 4.2 t Gd, 1.3 t Y, 50 kg Eu, 78 kg Ga, and 52 kg In (Wilburn, Citation2012) for LED production. The calculations are based on the study by Lim et al., (Citation2011).
Manufacturing of LED-packages
LED-packages can be fabricated by various methods. For example, the manufacturing of a specific LED-package designed by (Osram, Citation2009) is presented. The LED-chip consists of the following layers: carrier substrate wafer, solder layer, meta reflector, InGaN semiconductor, bond pads, and color converter. The chip is mounted in the package as depicted in .
Figure 7. Schematic drawing of an LED-package fabricated by Osram (Citation2009).
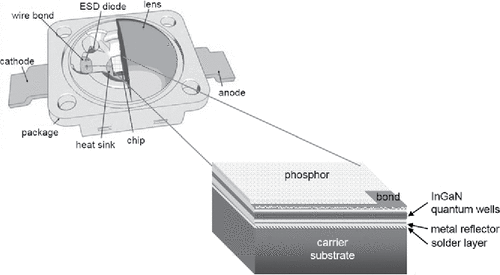
The main principle of the LED-fabrication includes following manufacturing steps (Navigant, Citation2012):
To fabricate the substrate tape wafer, ingots are grown through metal organic chemical vapor phase epitaxy (MOVPE). Then the ingots are cut into thin slices with a filigree steel wire, which is wet with a mixture of silicon carbide and polyethylene glycol (PEG). Subsequently, the single wafers are polished. The cutting wire has a length of multiple hundreds of kilometers and is placed in such a way that hundreds of wafers are cut simultaneously. The cutting losses are up to 50% of the wafer ingot, due to the thickness of the wire (Kübler, Citation2009).
The substrate is processed with several metallization and lithography steps to deposit structured barriers. After cutting the single LED-die, the components are mounted into a lead frame and the wires are bonded after plasma-cleaning. Finally, the color converter and lens are mounted. The LED-packages are individually tested and binned.
The phosphor or color conversion layer has a nearly negligible mass share of the LED-lamp. Nevertheless, the synthesis is expensive, energy-intensive, and requires chemical auxiliaries. Generally, the phosphor is fabricated by high-temperature solid-state reactions. The base materials have to be phase pure and of high quality. The materials are thoroughly mixed and homogenized for several hours up to five days. Then they are sintered, reground, and reannealed at temperatures between 1000°C to 1600°C in reduced atmosphere up to 20 hr, depending on the technology. The processes require chemical auxiliaries such as nitric acid, citric acid, ethylene glycol, isopropyl alcohol (Khanna, Citation2014; Pradal et al., Citation2013). Besides solid-state reaction also some other techniques are used for the fabrication of phosphors (Han et al., Citation2013). A lot of phosphor manufacturing processes can be also found in patent literature, exemplarily, e.g., the preparation by mixing the stoichiometric amounts of reactants alkaline earth metal carbonate, silica, and europium oxide. The mixture is sintered in reduced atmosphere at 1100°C–1400°C (Patent, Citation2010).
The entire fabrication process of the LED-package is carried out in clean room atmosphere (class 100–10,000) (Osram, Citation2009).
Fabrication tolerances
Both semiconductor die and color converter can only be fabricated within specific tolerances. Components for high quality LEDs with narrow tolerances of power consumption, color temperature, and luminosity, are binned and sold in a high quality market. There is no information about the market share of high quality components in comparison to the global medium quality and discount market. Unbinned LEDs have a much larger range of luminous efficacy variations, e.g., 30–70 lm/W. The International Energy Agency IEA 4E SSL Annex promotes an international harmonization of solid state lighting (SSL) standards, quality assurance, and testing methods (Bennich et al., Citation2015).
Quality-relevant and environmental parameters of LED-lamps
A high environmental performance of a LED-lamp includes a number of technological aspects and materials. The most important parameters are a high luminous efficacy combined with a large lifetime. They depend on chip, color converter materials, fabrication technologies, electronic ballast, and thermal management. The lighting quality is an indirect environmental parameter and is defined by the extent to which consumers will replace the lamp before its end-of-life, due to uncomfortable or impractical illuminance performance.
Luminous efficacy and further quality parameters of LED-lamps
There are different data concerning the luminous efficacy of LED-lamps. The basic value is the luminous efficacy of the LED-die. The assembly of the entire lamp consists of several components, which contribute to efficiency and energy losses in the operating state (Kölper et al., Citation2011):
All factors include thermal effects. In this case, the thermal efficiency depends on the operation temperature: as the junction temperature of the LED-chip increases fewer photons are emitted and the LED becomes less efficient. Intelligent thermal management can reduce this effect. In addition, during operation the color converter suffers from a large thermal load, which causes a temperature-dependent loss of the luminescence intensity of the phosphor (Fulmek et al., Citation2013; Wenzl et al., Citation2014).
In summary, the LED-lamp / luminaire system efficacy is reduced by 50%. Retrofit LED-lamps have a lower efficiency due to their limited space and design options for thermal management. Integrated LED-lamps have larger design opportunities for thermal management, because main parts of the luminaire can be included in the cooling design. The higher the technical features of drivers, e.g., dimming or color adjustment, the higher are the energy losses.
Warm white LED-lamps with low color temperature of about 2700 K are less efficient due to the higher color conversion losses of the blue or UV radiation. A commercial company provides data for realistically achievable final luminous efficacies of power LED-lamps, depending on the color temperature by the year 2011 (De Boer, Citation2011):
The current R&D Plan of the U.S. Department of Energy (U.S. DOE, Citation2016) reports typical luminous efficacy values for LED-lamps available on the market by the year 2015: 78 lm/W (2700 K), 94 lm/W (3500 K), 107 lm/W (4100 K), 96 lm/W (5000 K), which represents an increase of about 15% for high-end products compared to 2011.
A recent study conducted by Zissis (Citation2016) measured the luminous efficacy of 370 samples of warm white high-quality omnidirectional and spot LED-lamps for residential lighting from store shelves of the European Union, respectively. The study also measured the deviations from claimed values. shows the respective results from this study.
Figure 8. Distribution of measured luminous efficacies (lm/W). Light bars: LED bulbs, dark bars: LED spots (Zissis, Citation2016).
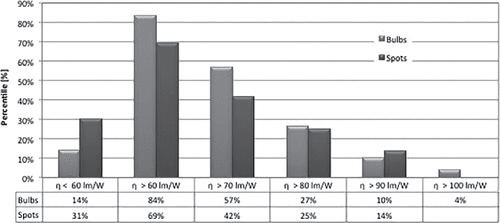
Besides the luminous efficacy and the color temperature, the following three lighting parameters determine the lighting quality of an LED-lamp: First, the color rendering index (CRI) is a measure for a lamp to reveal the colors of an object authentically. The CRI of LEDs depends on its color converter composition. Adding red or nitride-based phosphors increases the CRI but at the same time reduces the luminous efficacy of the lamp (Abdul Hadi et al., Citation2013; Tan et al., Citation2012). Second, a newer measure of the color rendering is the color quality scale (CQS), developed by the US National Institute of Standards and Technology (NIST). The difference to the CRI is that the test colors are all saturated and the value is not calculated from the arithmetical mean but from the root mean square.
Third, the lifetime of an LED is characterized by two further parameters: the light flux over the lifetime L and the probability of default B. The declaration [L70/B10], for example, indicates that the light flux L (light intensity), is at least 70% at the declared end-of-life. Additionally, B10 indicates that a maximum of 10% of the LED-chips have failed by the end-of-life.
The parameters CRI, CQS, L, and B do not have to be declared on LED-lamps in the European Union, however, the CRI is often specified on brand products.
Waste management of LED-lamps
At the end-of-life stage, LED-lamps become electronic waste which is generally classified as hazardous waste in the European Union. The European e-waste Directive (WEEE, Citation2012) requires recycling quotas for six categories of electronic equipment. The category “lamps” requires a recycling quota of 80% as of 2018. LED-lamps are collected in the category lamps, but are then sorted out in the recovery plant and transferred to the category “small equipment” (e.g., electric knifes, watches, Hi-fi equipment), which requires 75% recovery and 55% recycling (EAK, Citation2015).
At a global level, the waste disposal of LED-lamps can be performed in the following ways, depending on the regional regulations:
▪ | Hazardous / nonhazardous waste incineration | ||||
▪ | Hazardous / nonhazardous waste landfill | ||||
▪ | Formal recycling | ||||
▪ | Informal recycling | ||||
▪ | Uncontrolled landfill |
The Global e-Waste Monitor (Baldé et al., Citation2015) estimates that 1.0 Mt of waste lamps are generated worldwide. This corresponds to 2.4% of the total global e-waste. In developed countries, the recycling of e-waste takes place in controlled recovery plants. Informal recycling is established in China and India parallel to slowly developing recovery plants. The informal recycling is carried out by small enterprises, manually disassembling electronic waste, to gain precious materials under simple unhealthy conditions (Greenpeace, Citation2012). LED-lamps contain electronic ballast and it can be expected that they are also handled by the informal sector.
The European Union has a regulation for the restriction of hazardous substances in all kinds of electronic devices including lamps (RoHS 2, Citation2011) to minimize and control hazardous electronic waste, but it holds a lamp market share of only 25% of the global market (McKinsey and Company, 2012). In other countries the substance restrictions and waste treatment standards are very different. The India-RoHS and China-RoHS have similar restrictions. In India, small enterprises are excluded and in China restricted substances only have to be declared if they exceed the defined limits (China–RoHS, Citation2006; India–RoHS, Citation2014). US-American LED-lamps may contain lead (IEA, Citation2014). The study of Lim et al., (Citation2013) investigated the potential environmental impact from metals in LED-lamps and found that the U.S. regulatory limits for nonhazardous waste were exceeded in LED-bulbs for lead and copper.
Professional life cycle assessment databases are focused on Europe and have no data on uncontrolled informal recycling and uncontrolled landfilling. The end-of-life disposal is often neglected or even gains a benefit. Uncontrolled recovery produces dioxins, hazardous lead and tin vapor, isocyanates, chlorine gas, and sulfurous gas (BAN, Citation2002). There is no assessment of this situation.
LED-lamps include a small amount of valuable rare earths. A review article by Binnemann et al., (Citation2013) on recycling options found that there are no studies on recycling technologies for rare earths of LCD backlighting and LEDs. Concerning valuable materials, the study by Gassmann et al., (Citation2016) reports that a typical state-of-the-art LED-chip of 1 mm2 contains 90–200 μg color converter material and 3 μg rare earth doping elements. The corresponding semiconductor contains 17–25 μg of gallium and 28 ng of indium. The LED-package contains about 200 mg gold used for bonding wires. The same study proposes that the LED-lamp can be shredded by electrohydraulic fragmentation. They also propose that, as long as there is no proper recycling technology for these valuable substances, the LED-packages could be sorted out of the mixed LED-lamp fractions by UV-light irradiation detection and then be temporarily stored.
Review of life cycle assessment studies for LED-lamps
A life cycle assessment is a standardized methodology to evaluate the environmental impact of products or services, consisting of four stages: (1) The goal and scope definition defines the frame of the study including the system boundary, the functional unit, the considered life cycle stages, the breadth and depth of the manufacturing steps and supply chain, and the origin of data. Furthermore, simplifications, the assessment method, and considered impact categories are defined. (2) The life cycle inventory analysis (LCI) defines the system including the product trees and the reference flows. The input and output data are collected considering cut-off and allocation rules. In case of LED-lamps, this part includes large data uncertainties due to a number unknown proprietary manufacturing processes and company-dependent manufacturing variations in the same product category. (3) The life cycle impact assessment (LCIA) assigns the results of the life cycle inventory analysis to the selected impact categories. The LCA standard does not prescribe an assessment method or impact category to be considered. The calculated results are a relative expression of potential environmental impacts to the reference unit. (4) The life cycle interpretation includes the systematic analysis of the findings and qualifies the environmental conclusions according to the goal and scope definitions. A life cycle assessment does not include sustainability assessment and economic or social aspects (ISO, Citation2006a/Citation2006b).
The ideal life cycle assessment considers the following five life cycle phases: (a) raw material acquisition, (b) raw material processing, (c) manufacturing, (d) use phase, and (e) waste disposal. Besides the material composition of the product, all phases include proportional infrastructure, auxiliary materials and chemicals like water and detergents, packaging, waste, energy, and transport service. Due to the heterogenous and complex electronics technologies, supply chains, and data gaps, there is a large degree on simplifications necessary to model a specific electronic device, in this case LED-lamps, for a life cycle assessment.
Life cycle inventories for all lamp technologies
The environmental impact of LED-lamps has been assessed by several LCA studies in the last decade. In this review, 13 life cycle assessment studies are analyzed and their assumptions and outcomes are compared. Two of those studies are single case studies, including a full life cycle assessment of an indoor LED downlight luminaire (Tähkämö et al., Citation2013) and a gate-to-gate study for LED-chip manufacturing (Matthews et al., Citation2009). One supplementary study includes detailed process description for color converter manufacturing (Pradal et al., Citation2013). All other analyzed studies are comparative life cycle assessments, comparing the following lamp technologies in various configurations: (a) retrofit LED-lamp, (b) integrated LED-lamp, (c) incandescent lamp, (d) halogen lamp, (e) compact fluorescent lamp, (f) linear fluorescent lamp, (g) ceramic metal halide lamp, (h) high-intensity discharge lamp, (i) induction lamp, and (j) high-pressure sodium lamp. One of the studies does not cover LED-lamps, but it is included because of the poor comparative data situation for linear fluorescent lamps (Welz et al., Citation2011).
, continued by 5b, shows a detailed compilation of all relevant parameters and assumptions of the LCA studies in the order of their time of publication. It provides comparable information about (a) technical lamp parameters, (b) functional unit, (c) intended lamp application, (d) level of details concerning the LED-package, (e) light intensity measurements, (f) considered electricity mixes and waste management, (g) considered impact categories, assessment method, and data sources, and (h) nature and detail of the results.
Table 5a. Comparison of study settings, parameters, and transparency of the LCA studies.
Table 5b. Comparison of study settings, parameters, and transparency of the LCA studies.
, continued by 6b, refines comparable information about the LED-package in terms of (a) technical data, (b) considered manufacturing steps, (c) considered color converter, (d) novelty and details of inventory data, and (e) data source.
Table 6a. Comparison of parameters and manufacturing data of the LED-packages.
Table 6b. (Continued).
The life cycle assessment methods, software tools, and databases applied in the LCA studies are: (a) cumulative energy demand, (b) impact-oriented characterization CML (Institute of Environmental Sciences (CML), Univ. Leiden, The Netherlands, 2016), (c) Eco-indicator 99 (Baumann and Tillmann, Citation2004), (d) TRACI (Bare et al., Citation2002), (e) ILCD 2011 (Wolf et al., Citation2012), (f) GaBi (GaBi, Citation2016), (g) SimaPro (SimaPro, Citation2016), and (h) Umberto (Schmidt & Häuslein, Citation1996), including up to 16 impact categories each. Some of the studies solely include the primary energy demand (Navigant, Citation2012 Part 1) and the final electricity consumption in a gate-to-gate assessment (Matthews et al., Citation2009).
The LCA studies considered 39 different midpoint impact categories in various combinations. shows their application in the studies in alphabetical order. Sixteen of the impact categories are used by only one single study. The endpoint characterization method Eco-indicator 99 was applied in four studies (Abdul Hadi et al., Citation2013; Sangwan et al., Citation2014; Tähkämö and Halonen, Citation2015; Welz et al., Citation2011).
Table 7. Characterization categories used in LCA studies.
The life cycle inventory data are based on (a) the ecoinvent database, (b) the GaBi database, (c) the SimaPro database, (d) the European Reference Life Cycle Database (JRC, Citation2008), (e) the European Lamp Companies Federation, (f) the IDEMAT database, (g) ETH ESU 96, (h) Franklin USA 98, (i) USLCI, some further literature data, and new mass and energy measurements in the course of the respective study.
The studies applied following 20 different energy mixes for the electricity consumption: (a) America: US average, California mix, California with 33% renewable energy, NERC/RFC mix; (b) Europe: EU mix, EU UCTE mix, EU RER mix, UK mix, Swiss mix, French mix, Italy mix; (c) Asia: China, India mix; (d) others: 100% wind, 100% hydropower, wind/solar mix, 100% coal, natural gas, PV with battery recycling, PV without battery recycling. Almost all studies distinguish between manufacturing country and consumer country and/or calculate different energy source scenarios.
The intended application of the LED-lamps is in most cases indoor or not specified. LED-street lamps are investigated in four studies (Abdul Hadi et al., Citation2013; Dale et al., Citation2011; Hartley et al., Citation2009; Tähkämö & Halonen, Citation2015). One study investigated a specific LED downlight luminaire (Tähkämö et al., Citation2013), another one a specific working place lamp setting (Principi and Fioretti, Citation2014).
The chosen functional unit is an indicator for the comparability of LCIA results of different studies. The applied functional units are (a) n × (lm × h), (b) n × h, (c) 1 lm for n × h, (d) 1 lux for n × h, and (e) 1 km lit road, whereby n is a variable parameter.
The waste management is considered in all studies in terms of controlled formal disposal, including (a) household waste incineration, (b) recycling, and (c) landfilling. The disposal options are applied as single scenario or in a mixed form. One study discusses the environmental burden versus the environmental benefit of different waste treatments (Welz et al., Citation2011).
The assessed LED-lamps have largely varying technical parameters. The considered luminous efficacies, which are directly connected with the using phase impact, are in the range from 43 lm/W to 200 lm/W. Whereby the values of 134 lm/W is forecasted for 2017 (Navigant, Citation2012) and of 200 lm/W for 2020 (Tähkämö and Halonen, Citation2015).
shows the distribution of the assumed luminous efficacies and the respective lifetime of efficient lamps for all studies. The lifetime of LED-lamps is in the range from 15,000 hr to 59,000 hr. The HPS-lamp has a luminous efficacy of 73 lm/W with a lifetime of 32,000 hr. The linear fluorescent lamps have a luminous efficacy from 67 lm/W to 102 lm/W with a lifetime from 15,000 hr to 24,000 hr. The compact fluorescent lamps have a luminous efficacy from 43 lm/W to 76 lm/W with a lifetime from 6000 hr to 10,000 hr. The CFL-lamps show the lowest variance of the lamp parameters. CMH-lamps have a luminous efficacy from 77 lm/W to 104 lm/W with a lifetime from 12,000 hr to 20,000 hr.
LED-lamps show the largest variance of their assumed technical parameters. At present, the best practice available luminous efficacy with sufficient lifetime is about 100 lm/W for neutral white color (U.S. DOE, Citation2016).
Comparative results of all efficient lamp technologies
Comparative full life cycle results
The comparative LCA studies investigated the environmental compatibility of LED-lamps within efficient lamp technologies and in most cases also in relation to incandescent lamps. A direct comparison of absolute characterization impact values is not meaningful, because of different study settings in terms of (a) functional unit, (b) lamp parameters such as illuminance and nominal power, (c) energy mix, (d) considered characterization categories, and (e) exclusion of similar lamp parts. All studies assume comparable illuminance for the respective investigated lamps.
In terms of the environmental position of LED-lamps, the studies are examined for relative differences between the impact results of the lamp technologies. The results of comparative LCA studies are often close together. Therefore, the interpretation of the impact characterizations requires the definition of a significance threshold. The authors of the LCA Handbook discuss the need and character of a distinctness analysis applied to the respective case study to avoid a hasty “better-worse” result for a product (Klöpffer and Grahl, Citation2009). In practice, differences between impact characterization results of 10% are counted as significant (Friedel and Spindler, Citation2016). This value, a significance threshold of 0.1, is assumed for the further discussion. The study results are compared as they are, followed by critical comments on the assumptions.
shows a compiled diagram of two studies (Navigant, Citation2009, Citation2012), based on the assumption, that the reference values of 100% of the incandescent lamps are comparable in both studies. The environmental impact to a great extend depends on the luminous efficacy. LED-lamps with a forecasted luminous efficacy > 134 lm/W have the best environmental performance for all impact categories, because the manufacturing impact, which is consistently higher for LED-lamps, then has less significance. Considering commercially available lamps, the T5 fluorescent lamp shows better or equal impact values than all other technologies.
Figure 10. Compilation of the LCA results of 15 impact categories of Navigant (Citation2009) and Navigant (Citation2012). The reference values of the incandescent lamp are 100% for all impact categories (displayed 45%). The compared lamp types are: T5 fluorescent lamp, ceramic metal halide lamp CMH, compact fluorescent lamp CFL, and different LED-lamps.
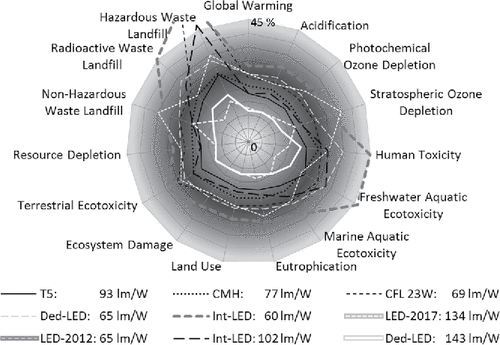
A further comparison can be made with the independently performed studies of Osram (Citation2009) and Navigant (Citation2009). They overlap in some impact categories and their absolute results are compared in the following: shows the comparison of the retrofit LED-lamps of both studies and the T5 lamp of the Navigant study. The values of Osram are also normalized to 1 M(lm × hr), with an assumed luminous flux of 345 lm. The reference value of 100% is related to the LED-lamp of Osram. The impact categories are divided in manufacturing phase and using phase.
Figure 11. Comparison of selected relative study results, divided into manufacturing phase indicated with ‘m’ and use phase indicated with ‘u’ (Navigant, Citation2009; Osram, Citation2009).
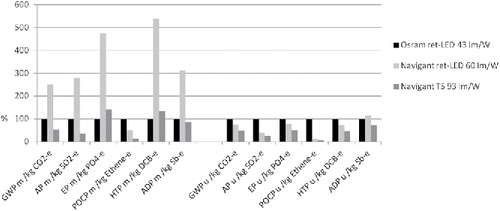
The bar graphs show that in the use phase (right), the impacts correlate with the luminous efficacies of the lamps and the deviations could be due to the differences of the EU energy mix and the UK energy mix. It should be noted that shows relative values. The impact results of the manufacturing phase are up to 98% smaller than the using phase.
The manufacturing phase (left) shows significant differences between the LED-lamps up to the factor 5. The T5 FL lamp has comparatively low impact values although the mass is 10 times higher.
shows a compilation of all studies, where the LED-lamps are compared with the other efficient lamp technologies according to the respective study. The left rows compare the entire life cycle impacts and the right rows the manufacturing impacts for all considered characterization categories. The index number of the lamps references to the luminous efficacy, if available. The comparative lamp technologies are written in italic. The brightness of the table cell (white/gray/dark gray) reflects if the LED-lamp has a higher or lower environmental impact than the comparative lamp. In the following, the main results between the lamp technologies are presented (see left table columns).
Table 8. Comparison of all lamp technologies per functional unit.
Compared to linear fluorescent lamps, LED-lamps ≤ 102 lm/W show a weaker performance for almost all impact categories (Navigant, Citation2009; Sangwan et al., Citation2014).
HPS lamps show in three cases a weaker performance for all impact categories than LED-lamps (Dale et al., Citation2011; Hartley et al., Citation2009; Tähkämö and Halonen, Citation2015). One scenario with a functional unit of 1 km-lit-road shows an equal environmental performance, because a larger number of LED-lamps are necessary to achieve equal illuminance (Tähkämö and Halonen, Citation2015).
The comparison with CMH/MH lamps shows a varied picture. One study shows different results throughout the impact categories for a LED-lamp with 102 lm/W: two categories show better results (GWP, ARD), four categories show worse results (HTP, FAETP, RWL, HWL), all other results are equal (Navigant, Citation2009). Another study show better results for EI99, equal results for energy/CO2, and worse results for water consumption (Abdul Hadi et al., Citation2013). Concerning MH lamps, two studies show a better environmental performance for LED-lamps for all impact categories (Dale et al., Citation2011; Hartley et al., Citation2009).
LED-lamps compared with induction lamps show for three impact categories a worse impact (HTPce, HTPnce, ODP) and for all other categories equal results (Hartley et al., Citation2009).
The last compared lamp type is the compact fluorescent lamp, which is considered in six studies. The results are contradictory for an LED luminous efficacy between 43 lm/W and 65 lm/W (Navigant, Citation2009, Citation2012; Osram, Citation2009; Quirk, Citation2009; Sangwan et al., Citation2014). Four studies show continually better impact results for LED-lamps with > 75 lm/W (Navigant, Citation2009, Citation2012; Principi and Fioretti, Citation2014; Quirk, Citation2009).
The applied electricity mix for the manufacturing and the using phase has a large influence on the amount of the final impact results and the relations between the impact categories. Coal has a higher GWP impact by 58% than the US average and the coal ETX impact is 60 times higher. Concerning the respiratory effects, the US average impact is three times higher than wind power and the coal impact is 20 times higher than the US average (Hartley et al., Citation2009). A further study found that the EU UCTE mix has a 3.8 times higher impact than the CH mix for EI99 (Welz et al., Citation2011). The Indian electricity mix shows a five times higher EI99 impact than the Swiss electricity mix (Sangwan et al., Citation2014). There is no comparison between the US and the EU electricity mixes.
One study compared the full life cycle GWP impact of grid electricity (natural gas) with photovoltaic electricity operated in island mode. The results show that PV with battery recycling has a 18% higher impact than natural gas. PV without battery recycling has a three times higher impact than natural gas grid electricity (Abdul Hadi et al., Citation2013).
Packaging and transport play a secondary role in all studies. The environmental impacts are in the range of 1% or less. The applied LCI data follow standardized packaging and transport assumptions with the focus on the final product. The transport may be underestimated. The U.S. Geological Survey reports, for example, that raw indium materials are extracted in one country, processed in a second location, and the LED-lamp is manufactured in a third location due to a few specialized companies (Wilburn, Citation2012). Osram reports that the LED-chip is fabricated in Germany and Malaysia. The LED-lamp is assembled in China (Osram, Citation2009).
Comparative manufacturing results
The manufacturing of lamps shows different impact relations than the full life cycle. LED-lamp manufacturing tends toward a significant weaker environmental performance than the full life cycle for all characterization categories. , right columns, shows the relative comparative impact results of lamp manufacturing compared to other efficient lamp technologies. The studies compare the manufacturing impacts per functional unit that is a different number of lamps per technology.
Compared to CFL, the manufacturing impacts of LED-lamps have contradictory results. Only LEDs with a luminous efficacy > 134 lm/W have a consistently lower impact (Navigant, Citation2009, Citation2012). Two studies including FLL show similar contradictory results (Navigant, Citation2009; Sangwan et al., Citation2014), this also applies to the comparison with CMH lamps (Abdul Hadi et al., Citation2013; Navigant, Citation2009).
LED-lamp manufacturing compared with HPS lamps shows in nearly all impact categories significant higher impacts (Hartley et al., Citation2009; Tähkämö and Halonen, Citation2015). One study found better values for GWP and RESP (Dale et al., Citation2011).
One study compared the manufacturing impacts per lamp unit. All LED-lamp results are worse or equal compared to induction, MH, and HPS lamps (Hartley et al., Citation2009).
Notable high manufacturing impacts for LED-lamps result in the categories human toxicity potential HTP and ecotoxicity ETX as well as by calculating with the EI99 method. The average manufacturing ecotoxicity of LED-lamps is four times higher than the compared other efficient lamps (Dale et al., Citation2011).
FL lamps are considered in three studies. The work by Navigant (Citation2009) includes the entire luminaire with two lamps with a mass of 3.79 kg. The study by Sangwan et al., (Citation2014) includes solely the lamp with a mass of 0.16 kg without ballast. The work by Welz et al., (Citation2011) considers the lamp including the external ballast with 0.23 kg. A comparison of the manufacturing impact results shows differences up to three orders. The lengths, diameters, and possible housings of FL lamps are very different and the material input is not necessarily proportional to the electrical parameters. All studies show that manufacturing of linear fluorescent lamps have the lowest impacts compared to the other efficient lamps. Furthermore, the recycling of rare earths of fluorescent lamps has already been commercialized unlike for LED-lamps (ERECON, Citation2015). Due to the close high efficiencies of FL lamps to current LED-lamps, the full life cycle impacts are highly dependent on the manufacturing and disposal impacts.
In conclusion, the higher the differences of the luminous efficacy and the lifetime of LED-lamps, the less relevant is the manufacturing phase.
Comments to study assumptions and statements
The following comments emphasize unique assumptions, results, and statements of the studies to be considered when interpreting the LCA results.
The work by Osram (Citation2009) calculated the primary energy consumption for manufacturing of one LED-lamp to 9.9 kWh. The fabrication of the LED-packages has a share of 30%. The LED-chip was fabricated in Germany (frontend) and Malaysia (backend) and the final production of the LED-lamp in China. It was assumed that the production of 1 kWh of electricity requires 3.3 kWh primary energy.
The study by Quirk (Citation2009) calculated the lamp assembly by adding 10% of the production data. Transportation was estimated with 8000 miles (12,875 km) by ocean-going freighter and 4000 miles (6437 km) by light duty truck.
The author states the problem that countries with increasing energy efficiency or renewable electricity production tend to outsource the manufacturing emissions to foreign countries with carbon intensive electricity mixes and there is no research about the imported embedded energy of products. This fact leads to distortion of the domestic emission inventories of up to 20%.
The primary energy demand of LED-lamp manufacturing is 65 kWh (± 30%), with about 50% associated with the printed circuit board and the other half to the LED-chip. The production of other lamp parts needs less than 2% energy each. CFL lamps have a primary energy demand of 38 kWh (± 30%). The variances result from uncertain energy data for printed circuit board and LED production.
The study by Navigant (Citation2009) provides detailed mass values for the lamp parts. Five of the investigated lamps have a mass between 30 g and 520 g. The T5 fluorescent lamp is specified with a considerable higher mass of 3.79 kg, of which the luminaire base and coverage have the largest share.
The printed circuit board of the LED-lamps was modeled as a 100 g block made of aluminium. Apart from that, the study states, that the ceramic halide and T5 fluorescent lamps do not score well in hazardous waste landfills, due to the contained printed circuit board.
The study by Hartley et al., (Citation2009) accompanied a pilot replacing program for the city of Pittsburgh, USA. The lamp samples met defined illumination specifications and the illuminance and the power of each lamp type were measured before and after replacement. These measurement results are not published in the study. The LED bulb was modeled using an average of different samples based on the input/output and ecoinvent database.
The study by Dale et al., (Citation2011) seems to base on similar data sources than Hartley et al. The paper defines the exclusion of the pole and the arm attaching the lamp to the pole due to identical inventory data. Life cycle inventory data of the housing were gained by disassembling and bulb data were taken from the US I/O database. The study further states that I/O models overestimate impacts, whereas process databases underestimate impacts. Concerning the color temperature, the study reports that higher color temperature can upset the circadian rhythm of plants, animals, and people. For this reason, the city of San Diego, for example, has set a maximum color temperature of 3500 K.
Welz et al., (Citation2011) conducted a comparative life cycle assessment study for four different lighting technologies. Although no LED-lamp was assessed, the study results are relevant for this review due to the fact that there are environmental impact results of linear fluorescent lamps. They are rarely compared in comparative life cycle assessment studies on LED-lamps despite of their good environmental performance. The external electronic ballast was included, but the luminaire was not considered (see Navigant, Citation2009). The results of the study show that the manufacturing impact of the linear fluorescent lamp has a share of 8% (Swiss mix) and 2% (European UCTE mix) of the total life cycle impact.
The study further investigated the effects of different lamp qualities on the environmental impact of compact fluorescent lamps. The manufacturing impacts differ between 2% for no-name products and 6% for premium products of the full life cycle impact (European UCTE mix). There is no information about similar studies of LED-lamps.
The study by Abdul Hadi et al., (Citation2013) compared two lamps with a lifetime-dependent luminous flux of 25,000 lm–17,500 lm (CMH lamp) and 19,000 lm–15,000 lm (LED lamp). These two lamps were considered as equally. The mean luminous flux of the LED-lamp with 17,000 lm is 15% lower than of the CMH lamp with 20,000 lm (GE Lighting, Citation2011). The study defines that the LED lamp matches the minimum luminous flux of the CMH lamp at the end of the life time. Increasing the calculation values of the LED-lamp by 15% to an equal mean level of the CMH lamp, the eco-indicator damage points are close to each other.
The aluminium content of the lamp housing was assumed to be equal and was therefore excluded from the calculations. The masses of the street lamps were scaled up from data of indoor CMH and LED-lamps, investigated by Navigant (Citation2009). LED-lamp inventory data were taken from Navigant (Citation2009), Matthews et al., (Citation2009), and Hartley et al., (Citation2009). The electricity scenarios were modeled with natural gas grid, stand-alone photovoltaic panels with and without battery recycling.
The study by Tähkämö et al., (Citation2013) provides detailed manufacturing results of an LED-downlight luminaire for 16 impact categories. The lifetime of one LED-lamp was modeled between 15,000 hr and 50,000 hr, which causes significant influences on the full life cycle impact. shows the relative manufacturing results of 16 different environmental characterization categories.
Figure 12. Manufacturing impacts of the LED downlight luminaire (Tähkämo et al., Citation2013).
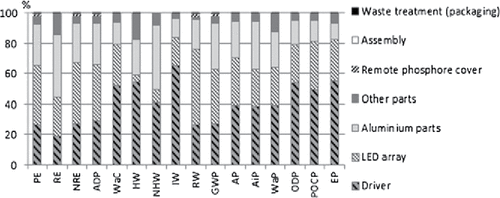
The driver and the LED-array show the highest values for all impact categories in variable relations, followed by the aluminium parts. The remote phosphor manufacturing has a visible impact only for PE, NRE, ADP, RW, and GWP.
The study by Principi and Fioretti (Citation2014) investigated a test office chamber with a working table in the center and four light sources on the ceiling. The light sources were chosen in such way that the measured total illuminance on the center desk was at least 750 lux. The study is an interesting case that the setting of LED-lamps needs 1/3 less luminous flux than the CFL-lamp set for equal lighting quality. The results show that besides the higher luminous efficacy, the LED-lamps provide better opportunities to control and focus the light flow.
The authors of the study further remark that mercury contained in CFL-lamps only covers 2% of the life cycle emission of mercury. 97% of the mercury emissions arise from the electricity production.
The study by Sangwan et al., (Citation2014) includes the linear fluorescent lamp without considering electronic ballast.
The work by Tähkämö and Halonen (Citation2015) compared streetlamps with three different functional units: per luminaire, per lm × hr, and per km-lit-road. The results of which have significant influence on the environmental impact classification of the lamp technologies. The technological standards for street lighting require a pole spacing of 50 m for HPS lamps and 36 m for LED-lamps. This results to an increased amount of 39% for installed LED-lamps. The study did not consider the poles. Therefore, in the case of the km-lit-road scenario the results for the LED-lamps have to be adapted by a plus of 39% for pole manufacturing. A further interesting result is that the cover of the LED-luminaire shows a manufacturing impact share of more than 90%. Depending on the impact category, only 1% to 6% is assigned to the LED production.
Comparison of characterization category results for LED-lamps
The previous results show the environmental impact relations between all lamp technologies. This section analyzes the bandwidth of impact characterization results only for LED-lamps. All LCA study results were compiled into the respective impact category. shows the relations between the life cycle stages for all considered characterization categories, which are dependent on the technical lamp parameters and energy mixes. The bar charts distinguish between manufacturing and use phase. Where available, the impacts for LED-package manufacturing (besides ballast and housing) and end-of-life treatment are shown separately.
The global warming potential GWP was investigated by the largest number of LCA studies. Therefore, it is the most representative comparison of the study results. The manufacturing share is between 2% and 82%. Basically, the differences depend on four variable parameters: The luminous flux and the lifetime influence the number of lamps to be produced, that is proportional to the share of the manufacturing impact. The luminous efficacy and the electricity mix affect the share of the using phase impacts. The LED-packaging fabrication share of the manufacturing phase is in four cases 50% to 60% (Navigant, Citation2009; Quirk, Citation2009), in one case 15% (Hartley et al., Citation2009), and in one further case 2% (Tähkämö and Halonen, Citation2015).
The manufacturing influence of the lifetime is illustrated by the comparison of 15,000 hr, 30,000 hr, and 50,000 hr. The manufacturing share increases from 15% to 42% with decreasing lifetime (Tähkämö et al., Citation2013). The influence of the selected energy mix is reflected in the comparison of the EU mix with hydropower electricity, where the using phase share decreases from 88% to 18% (Tähkämö and Halonen, Citation2015). Another electricity scenario shows that the end-of-life battery treatment of remote photovoltaic systems significantly increases the using phase impact compared to natural gas electricity generation (Abdul Hadi et al., Citation2013). Other variations of the impact share seem to depend on different lamp modeling, which is not only explainable by physical lamp parameters and electricity mixes (e.g., Osram, Citation2009; Quirk, Citation2009).
The relations between manufacturing and use phase highly vary between the studies. The lamp manufacturing has a low influence on the global warming potential compared to the other characterization categories. On the other hand, the (eco)toxicity potential, the photochemical oxidation potential, and the eutrophication potential are significantly influenced by manufacturing. Outstanding manufacturing impact results with a share of 82% and 96% in the category human toxicity potential shows the only study applying the input/output database for the life cycle inventory (Hartley et al., Citation2009).
The characterization categories hazardous and nonhazardous waste show the highest manufacturing impact shares. The end-of-life treatment is relevant for only a few number of impact categories. The most harmful influence is shown in the categories of freshwater ecotoxicity, human toxicity, and hazardous waste.
The only endpoint assessment method Ecoindicator 99 is applied by three LCA studies for LED-lamps. The manufacturing impact share is between 10% and 48%. These results correlate in line with the environmental damage of the electricity generation in the following order: hydropower, Swiss mix, natural gas, EU mix, and India mix. The end-of-life impact share amounts to 1.8% in case of hydropower and 0.6% in case of the India mix, which does not correlate to the other relations.
Life cycle inventories and assessment results for LED-packages
The LED-lamp consists of the light source, in this case the LED-package, and further electrical and mechanical parts. This section investigates how the various materials and technologies of LED-packages discussed in the previous chapter are represented in the life cycle inventories of the LCA studies. shows the considered materials, processes, and data sources of the respective studies.
The LED-package life cycle inventory data most commonly used are provided by the ecoinvent database. Six of the LCA studies applied this dataset on a mass basis directly or in a modified form. New LCI data were determined by four studies (Matthews et al., Citation2009; Navigant, Citation2012; Osram, Citation2009; Pradal et al., Citation2013), whereby the first three references considered the wafer manufacturing. Two studies applied cost-based LCI data (Dale et al., Citation2011; Hartley et al., Citation2009).
The investigated lamps include between 6 and 16 LEDs in case of indoor application and 92 to 180 LEDs for street lamps. The listed mass varies from 0.08 g to 2.00 g per LED. As far as specified, the single LED-dies have a size of 1 mm2 with a power of 1 W. The luminous flux varies from 50 lm to 71 lm per LED for commercial indoor lamps and 105 lm or 116 lm per LED for street lamps. The color converter layer is explicitly considered by three studies (Osram, Citation2009; Pradal et al., Citation2013; Tähkämö & Halonen, Citation2015). Two studies include a remote color converter (Navigant, Citation2012; Tähkämö et al., Citation2013). All studies applied conventional raw material data with no specific high-purity processes for the basic semiconductor and phosphor materials. In the following, specific assumptions, considerations, and results of the studies are summarized.
Ecoinvent LED-dataset, 2007/2010
The ecoinvent database provides life cycle inventory data for the component “light emitting diode, LED, at plant” (Hischier et al., Citation2007) based on a conventional 5-mm LED for through-hole mounting with a mass of 0.35 g and a luminous flux of 4 lm. The dataset is identical to “Diode, glass-, for through-hole mounting, at plant.” shows the material composition of the LED sample and the referring production efforts according to the dataset “production efforts, diodes.”
Figure 14. Life cycle inventory data of LEDs in % and production efforts, according to the ecoinvent dataset “light emitting diode, LED, at plant.”
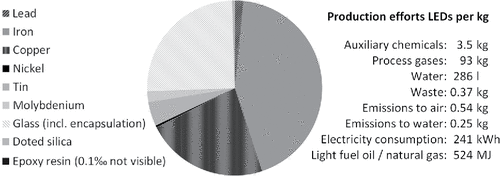
The material “doted silica” is defined by the dataset “silicon, electronic grade, at plant,” which is reported in the Photovoltaic Report of the ecoinvent database (Jungbluth et al., Citation2009). This dataset does not include wafer fabrication.
Osram-Siemens–study, 2009
The study conducted by Osram (Citation2009) provides new data for LED-chip manufacturing. shows the primary energy consumption for one LED-chip fabrication in Germany (frontend) and Malaysia (backend).
Figure 15. Primary energy demand for one single LED-package manufacturing (Osram, Citation2009).
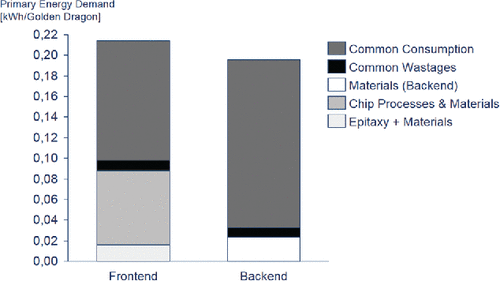
The total energy demand was 0.41 kWh. There are no details available of inventory data, used datasets for LED-chip and color converter materials, and their fabrications. The study assumed that there is no reject rate during LED-chip manufacturing. A worst case scenario increased the primary energy demand by 27%.
Quirk–study, 2009
Energy data for LED-chip fabrication were taken from Matthews et al., (Citation2009). The primary energy demand of the LED-chip fabrication was about 33 kWh (± 30%) or 119 MJ (± 30%) for a 6 W LED-lamp, without further information about the LED-array.
Navigant Consulting Europe–study, 2009
The life cycle inventory data for the LED-package were taken from the ecoinvent database. shows the full life cycle impact results of a dedicated LED-lamp, where the impact shares of the LED-package are outlined for all characterization categories. In the bar graph legend, the visible impacts are underlined and the “lamp” share (= LED-package) is shown hatched.
Figure 16. Full life cycle impacts of a dedicated LED luminaire system (Navigant, Citation2009) mod.
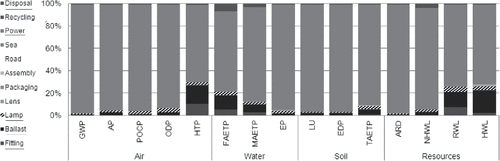
Matthews et al.–study, 2009
The study provides new life cycle inventory data for the manufacturing of 1 mm2 high brightness LED-packages in terms of the final electricity consumption from wafer growing to bonding and packaging. Energy data were collected from manufacturers and measured in two research scale LED-chip laboratories. Besides for the MOCVD reactor, the electricity consumption of auxiliary laboratory equipment was measured. Further processing steps of the LED-chips were calculated based on literature data of life cycle inventory data of the semiconductor logic chip production. The functional unit was a 300 mm silicon wafer with 50% yield rate, which results in 1000 pieces of LEDs per single wafer. The authors state that there is a lack of data for the color conversion layer and it was assumed that the energy consumption is very little. The final electricity consumption results are 0.02 kWh/LED on a laboratory scale and 0.07 kWh/LED on a production scale. It is notable that the electricity consumption of the production scale is more than three times higher than that of the laboratory scale.
The production scale results compared to those of the study by Osram (Citation2009) differ by a factor 2: 0.07 kWh × 3.3 = 0.23 kWh primary energy demand compared to 0.41 kWh. The factor 3.3 is the used conversion factor between the final electricity demand and the primary energy demand. It should be noted that in the study by Osram (Citation2009), the raw material acquisition is taken into account with no further detailed data.
Hartley et al.–study, 2009
The authors of the study modeled three different LED-streetlamp systems and calculated a mean value for the LED-lamp manufacturing. Two lamps included the LED type “Leds – 84 – Nichia 084B” with a mass of 50 g. The LED-chip and board/array inventory data were based on the ecoinvent database. It was assumed that each lamp contains 92 LEDs with a mass of 0.24 g per LED. The LED-bulb was modeled with the input/output LCA method using the “Semiconductors and related device manufacturing” sector. The estimated prices for one LED-bulb were $ 9.20, $ 250.00, and $ 322.00.
U.S.Department of Energy–study, 2012
Part 1 of the study by Navigant (Citation2012) evaluated and compared the results of the primary energy demand of LED-chip manufacturing of previously published LCA studies. The average result was then applied to the full life cycle assessment. Emissions during LED manufacturing were not considered. The authors discussed the problem of the comparability of LED-chip results regarding the illuminance and the number of LEDs. The manufacturing energy for one single LED-package is independent of the luminous flux and was multiplied by the number of used packages.
Part 2 of the study provides new manufacturing data for the LED-package. The assumed LED-technology was GaN on sapphire substrate with a remote YAG:Ce3+ color converter. More than 75 processing steps were considered from wafer and semiconductor fabrication to LED-packaging. All data relates to one 3 inch wafer. The wafer fabrication losses were assumed to be 63% of the raw material consumption. The number of LED-chips was assumed with 2438 units per wafer (3250 units in 2017). The (end-)energy consumption for the wafer and the LED-die fabrication is 60.87 kWh/wafer. Herein the wafer fabrication has a share of 30%. Including the assembly efforts, one single LED-package requires 0.055 kWh (0.2 MJ) electricity.
The remote color converter particles were specified with 0.192 mm3 phosphor per LED-lamp. The phosphor mass was assumed with 1.0 g and the plastic phosphor host with 11.1 g. For both materials, the dataset “rare earth concentrate, 70% rare earth oxide (REO), from bastnasite, at benefication” was used.
The study compared the environmental impacts of the ecoinvent LED-data with the outcomes of the new calculated LED manufacturing data on a per-lumen basis: The average impact of the new LED-package declined by 94.5%. The study provides only the final results of the comparison. Assuming that the ecoinvent-LED has a mass of 0.35 g with a luminous efficacy of 4 lm (Hischier et al., Citation2007) and the new explored LED-package has a mass of 0.09 g with a luminous efficacy of 70 lm (Navigant, Citation2012), the average relative impact can be recalculated on a per-kg basis: the average relative impact on ecoinvent basis has to be multiplied by the factor 3.75. For comparison, the study by Tähkämö et al., (Citation2013) assumed a correction factor of 5.
Finally, the study assumed that in a forecast scenario for 2017, the number of LEDs and driver electronics are reduced by 33% and all other materials and auxiliaries are reduced by 20% for the same light output.
Abdul Hadi et al.–study, 2013
The authors assumed that one LED corresponds to a system electricity consumption of 1 W and generates a resulting luminous flux between 83 lm and 106 lm. Life cycle inventory data were taken from the SimaPro database “Light emitting diode, LED, at plant”, modified by adding a fabrication energy of 0.045 kWh/diode.
Tähkämö et al.–study, 2013
The study includes an LCA case study of an LED downlight luminaire with a remote color converter. The authors state that the ecoinvent LED-data do not comply with the state of the art and considered that the data are highly underrated. Therefore, the life cycle inventory data for the LEDs were multiplied with a correction factor of 5. The LED-array consists of 16 LEDs (17%), a silicone product (12%), and aluminium (71%) with a mass of 32 g. In the manufacturing phase the LED-array had an environmental impact share of about 30%, depending on the impact category (see ).
The remote phosphor cover includes a YAG coating (2.7%), aluminium oxide (1.35%), organic chemicals (1.35%), and plastics (94.6%) with a total mass of 7.4 g. The electricity consumption for the color converter fabrication is 0.002 kWh. The impacts of the remote phosphor cover is visible in five categories, PE, NRE, ADP, RW, and GWP to a few percent.
Pradal et al.–study, 2013: Fabrication of a YAG:Ce color converter
The study includes the fabrication process of cerium doped nanogarnets as color converter material on basis of yttrium aluminium. In the following the life cycle inventory relevant processes are described. The YAG:Ce raw materials, Yttrium(III) acetate hydrate, cerium(III) acetate hydrate (∼0.5 wt%), and aluminium isopropoxide are mixed with 1,4-butanediol and diethylene glycol, which are stirred for 2.5 hr to a homogenous suspension under mild heating. Then the mixture is stirred in an autoclave for five days at 225°C and a pressure exceeding 10 bar. At the end of the process the mixture is cooled down to room temperature. Subsequently, the yellow colored translucent suspension is dried at different temperatures (80°C to 250°C) and then calcinated at different temperatures of up to 1100°C. The weight loss is about 17.5 wt%, corresponding to the organic additives of the mixture.
To gather life cycle inventory data, which was not in the scope of the study, there is no information about the initial wt% of the materials and chemicals, the temperature profile of the calcinating process, and the yield of LED color converters per fabrication cycle. It would be interesting for further research to get more information about the related mass and the energy consumption.
Tähkämö and Halonen–study, 2015
The study includes detailed LCI data for a streetlamp. The LED-array has a total mass of 214 g, which includes 98 LEDs (16%), aluminium (62.6%), ABS plastic (5.7%), and copper wires (15.7%). Besides the materials, the study includes also process data for aluminium, injection moulding, and wire drawing. The LEDs were calculated based on US DOE (Navigant, Citation2012). The array dataset includes transport data for transoceanic freight ship (0.284 tkm) and lorry (0.439 tkm).
Furthermore, the study provides a comparison of two LCI datasets for the LED-array. The one is based on the ecoinvent dataset “light emitting diode, LED, at plant” (Hischier et al., Citation2007) and the other includes LCI data from US DOE (Navigant, Citation2012). shows the environmental impact results normalized to the 100% values of US DOE. The range of deviation is from 58% to 180%, depending on the impact category. The impact categories GWP and ADP are equal. The ecoinvent dataset shows significant worse results for MAETP, ODP, and TETP.
Figure 17. Environmental impacts of the LED-array based on US DOE inventory data (gray) compared with the ecoinvent LED dataset (black) (Tähkämö and Halonen, Citation2015).
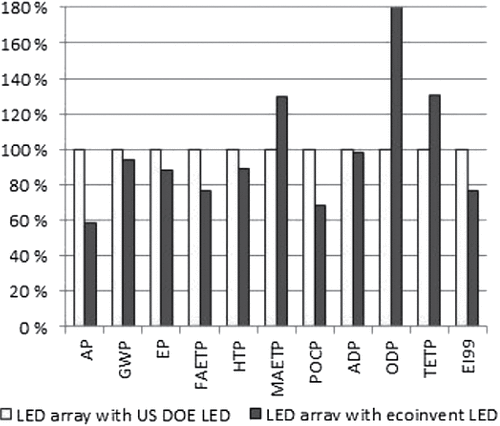
Primary energy demand for LED-package manufacturing
Part 1 of the study by the US DOE (Navigant, Citation2012) evaluated and compared the results of the primary energy demand of LED-chip manufacturing of previously published LCA studies. shows the compilation of the results, extended by the results of the same study, Part 2, and the ecoinvent production efforts.
Figure 18. Primary energy demand of the manufacturing of one LED-chip/package (Navigant, Citation2012–Part 1), including literature data of Matthews et al. (Citation2009), Quirk (Citation2009), Osram (Citation2009), Navigant (Citation2009), and extended by Navigant (Citation2012–Part 2), Hischier et al. (Citation2007).
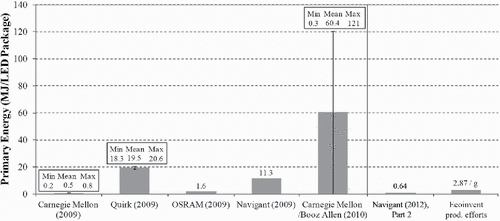
The Carnegie Mellon/Booz Allen (Citation2010) results are based on the semiconductor fabrication of Matthews et al., (Citation2009), but also include the energy consumption of substrate fabrication, and material extraction and processing (see Navigant, Citation2012). The end energy values for Navigant (Citation2012) and ecoinvent were multiplied by the factor 3.3 according to Osram (Citation2009). The ecoinvent energy consumption considers the production efforts per gram, which easily can be adapted by the LED masses of . The value of 2.87 MJ is a minor limit and does not include the energy efforts for the materials, see .
Life cycle inventories for rare earths
LED-packages contain a small amount of rare earth elements in their diverse application, which have been described in a previous section. All reviewed life cycle assessment studies only refer to a standardized dataset. However, life cycle inventory data of rare earth oxides show a big variance between the elements. A study published in 2014 investigated the energy consumption, water consumption, greenhouse gas emissions, and resource depletion of rare earth oxide manufacturing (Koltun and Tharumarajah, Citation2014). shows the environmental impact results for the production of 1 kg rare earth oxides, using price-based allocation. The widely used europium(III) oxide shows in nearly all impact categories a significantly higher environmental impact than the majority of the other oxides.
Table 9. Life cycle inventory and impact results for the production of 1 kg rare earth oxides using price-based allocation. (Koltun and Tharumarajah, Citation2014).
Discussion
The published life cycle assessments of LED-lamps vary between comparative and single case studies with very different theoretical and measured data, assumptions, simplifications, applied assessment methods, and data sources. A small number of comparative studies include measurements for the particular lighting situation which shows, that by solely equating the luminous efficacies the results do not necessarily represent an equal illumination condition.
The comparative study results show that the using phase for LED-lamps has the lowest environmental impact of all lamp technologies for a luminous efficacy > 104 lm/W without considering the lighting situation. In terms of the manufacturing phase, single linear fluorescent lamps have the lowest and LED-lamps have the highest environmental impact of efficient lamp technologies. The manufacturing impacts of LED-lamps vary between 1% and 96% of the total life cycle, dependent on the luminous efficacy, electricity mix, lifetime, and considered characterization category. The determined impacts of transport and packaging are < 1% each. The environmental impact of the waste disposal ranges from negligible to 27%, or even as raw materials or energy benefit due to recovery of the materials.
The general technical and environmental discussions of LED-lamps are within a mixture of different requirements for domestic, public, business, and outdoor applications, which is reflected in the study settings and their conclusions. All studies are single case studies or application-neutral theoretical studies and their results are readily generalized for the broad quality field of “LED-lamps.” For a particular lighting situation, due to the very specific assess scenarios the results of the life cycle assessment studies are not directly applicable for the selection of the best efficient lamp technology.
Compared to incandescent lamps with a linear daily operation time, LED-lamps are far superior with respect to the environmental impact of the use phase electricity consumption. Compared to other efficient lighting technologies, it is not possible to give a clear recommendation about the best environmental performance, which have, in some cases of LED-lamps, proven to be too optimistic. In specific fields of application, like architecture, exhibition, product lighting or spot lighting for working places, LED-lamps likely provide the most efficient lighting technology in combination with appropriate color rendering and lifetime. For other general lighting situations like residential, offices, industrial, or street lighting, there is no clear result of the lamp technology with the best environmental performance, considering equal lighting situations. Linear fluorescent lamps, ceramic metal halide lamps, induction lamps, and high-pressure sodium lamps have similar environmental performances. There are a few comparative studies between linear fluorescent lamps and LED-lamps. Due to the almost equal environmental impact, further studies with current lamp designs including measurements of the particular lighting situation would be recommendable.
The high environmental impact of the manufacturing phase of LED-lamps can be compensated by exceeding a high value of the luminous efficacy combined with a large lifetime, in comparison to other efficient lamp technologies.
The following summary describes which outcomes and aspects should be considered to untangle and differentiate the study settings and results.
Application of lamps
▪ | The public and business sector primary use energy efficient fluorescent lamps for general lighting. The same applies to street lighting, where fluorescent lamps, metal halide lamps, or sodium-vapor lamps are mainly used. None of the conducted life cycle assessments could proof significant better environmental performance for LED-lamps available on the market. | ||||
▪ | Incandescent, halogen, and LED-retrofit lamps are associated with the domestic sector and special applications. They have different requirements on the lighting quality, such as color temperature, color rendering, reflection angle, or glaring. | ||||
▪ | It is important to distinguish household, business, industrial, and street lighting, and to define, which function the respective light source should have. Even in households, lamps have to meet different requirements, such as illumination of hall, working place, or living space. | ||||
▪ | LED retrofit lamps, dedicated floor lamps, ceiling lamps, and street lamps have different electrical (e.g., lightning, dimming), mechanical and thermal requirements. Only the LED-chips are likely to be equal, all other parts vary and therefore consist of different materials. |
Lamp quality
▪ | Not all life cycle inventory data differentiate with respect to the quality of materials, components, and lamp parts, which has effects on manufacturing details and the lifetime of the product. Particularly LED-lamps require thermally well-designed components and high-quality LED-chips to meet the required and assumed properties. There is no information about the global ratio of high-quality LED-lamps with state-of-the-art luminous efficacy, color rendering, lifetime, etc., and the average LED market. One study found that in case of the investigated compact fluorescent lamps, there is a difference of the manufacturing impacts by 300%, depending on the manufacturer (Welz et al., Citation2011). | ||||
▪ | In comparative studies, equal lighting situations are rarely considered or not defined, such as light angle, color temperature, light density, etc., apart from the luminous efficacies. It is recommended to measure the particular lighting situation and to modify the assumed lamp parameters. | ||||
▪ | LED-lamps tend to change the color temperature with ageing. In the case of the illumination of conference halls or in similar largely connected illumination, it might be difficult to guarantee a stable quality for all lamps and equal replacement for a large lifetime of 50,000 hr or more. It is not only a technical, but also a commercial question. |
General statements on the study results
▪ | The calculation with different assessment methods leads to different impact results. Applying categories such as cumulative energy demand or global warming potential leads to an underestimation of the manufacturing phase for LED-lamps. In this case, comparative studies in fact compare the nominal power, the luminous efficacies, and the electricity mixes, respectively. Other impact categories, such as respiratory effect, human toxicity, ecotoxicity, and hazardous waste in landfills, have a high manufacturing and waste disposal impact. The manufacturing results for the eco-indicator 99 method are significant higher. | ||||
▪ | The life cycle assessment results provide no information about the lighting quality, such as color temperature, color rendering, lighting angle, disturbing glare, and long-term reliability of the lamp. These parameters are often described in the introduction of single case studies, but they do not have equivalents, neither as life cycle inventory data nor as results. The investigated LED-lamps are assumed to be of high quality and have the technically highest possible luminous efficacy. The state of the art and the representative market offer for efficient lamps are very diverse in the world. Therefore, the conclusions of the studies are not necessarily representative for the average global lighting market. | ||||
▪ | The characterization results of LED-lamps are very close to other high efficient lamp technologies. To clarify the differences, it is recommended to refine life cycle inventory data and to conduct comparative life cycle assessments of the same lamp technologies. These studies should consider no-name and premium products, and different applications scenarios. It is recommended to conduct measurements of the particular lighting situation, also including the individuals' perception of the light. |
Luminous efficacy
▪ | The luminous efficacy of the lamp is the key parameter for the environmental impact in the using phase. Warm white and cold white color temperatures, for technical reasons, have different luminous efficacies. Some comparative studies assume non-matching values between the lamp technologies, do not define the efficacy, or assume forecasted values, which are still not state of the market. | ||||
▪ | The luminous efficacies of the modeled LED-lamps vary between 43 lm/W and 143 lm/W. The material composition of the LED-lamp, their manufacturing, and life cycle inventory data are independent of the efficacy in a wide range. The currently available nominal luminous efficacies on the consumer market of warm white retrofit lamps are about 65 lm/W in the low price sector, and 80 lm/W to 100 lm/W in the medium price sector in Europe. A low number of high efficiency LED-lamps and LED filament light bulbs reach up to 115/130 lm/W, but with a significant shorter lifetime. Their average nominal lifetime varies between 10,000 hr and 25,000 hr and is far below the expectations of retrofit lamps in the studies (Market, Citation2016). | ||||
▪ | On a global level, LED-chip manufacturing is company dependent and does not have identical materials, processes, and qualities and has therefore different luminous efficacies. The decision between high efficient lamps is a decision between specific manufacturers (and price) and not necessarily between lamp technologies. | ||||
▪ | The final luminous efficacy of the LED-lamp is the product of the LED-chip efficacy and the efficiencies of the optical lens, the driver, and the thermal efficiency. Particularly for integrated LED-lamps with the technical potential of better values, it is not possible to define a universal value, due to the lack of production quality standards. It can be assumed that the international mass market is not oriented on the best manufacturing practice. This fact reduces the global mean value of the efficiency. |
Manufacturing of LED-lamps
▪ | Material input data differ significantly between the studies. Some provide a rough classification of five to six material groups, with corresponding mass data, others have a listing of 100 or more materials and production steps. LED-lamps can also contain hazardous materials. | ||||
▪ | Some comparative studies exclude lamp parts, materials, and processes, if they are equal for all compared lamp types. This becomes problematic, if the results are compared to the using phase, and if the results are compared with other studies. Life cycle inventory data are often taken from other studies. This can lead to unnoticed chains of errors for the particular assessment situations. | ||||
▪ | The distinction between lamp and luminaire should be taken more into account. Some lamp types are connected inseparable to the luminaire. It depends on the geographical location, in Europe for example, it is not common to install a lamp without a luminaire as a lamp is a design element and not only a functional light source. Integrated LED-lamps have the problem of a relatively large mass of the luminaire, which leads to a significant environmental burden for shorter lifetimes. | ||||
▪ | There is no life cycle assessment of LED filament light bulbs, which have no aluminium cooling. | ||||
▪ | General lighting consists of the lamp, the fixture, the driver, and the system control. The lighting statistics for the year 2016 (McKinsey and Company, 2012) show that the new installed or replaced lamps (without the other parts) have a share of only 25% of the economic general lighting market. The main share is the fixture level with 60% and the remaining part of 15% include the driver and system control level. These last 75% are connected with a significant but unknown material input, which is not reflected and differentiated in the life cycle assessment studies. | ||||
▪ | Cleaner electricity production and higher lamp efficiency leads to a significant reduction of the total impact of a lamp, but increases the share of the manufacturing phase. Compared with other lamp technologies, LED-lamp manufacturing has the highest environmental impact. From the manufacturing countries point of view, there is no relation between the manufacturing phase and the use phase for export lamps. This leads to the paradox situation that LED-lamps produced for the own national market, reduce the environmental burden of the country, but LED-lamps produced for the export market increase the country impact, compared to other efficient lamps. |
LED-package
▪ | The LED-chip is the key component of a LED-lamp. In the studies there is no clear distinction and definition between LED, LED-chip, LED-die, LED-package, LED-module, or LED-array. The die size of a LED-chip is assumed with 1 mm2 or not defined at all. The impact of the chip is dependent on the mass and the assumptions of the studies vary significantly. | ||||
▪ | Besides the LED-chip, the color converter is the second key component of an LED-lamp. The manufacturing of the high-tech color conversion layer is presumably not sufficiently considered in the life cycle assessment studies. It is often neglected or if considered, the manufacturing impact amounts up to a maximum of 2% in a few impact categories, in the case of a large remote phosphor layer. | ||||
▪ | The LED-package is composed of different materials than the databases includes. The raw materials for semiconductors and phosphor particles are simplified to aluminium oxide and silicon, which are provided in the databases. The aluminium oxide dataset of the ecoinvent database has a purity of 99%, which is not pure enough for semiconductors. There is no information, if these assumptions are sufficient for detailed comparative studies. |
Electricity consumption
▪ | The lifetime of LED-lamps is the key parameter for a good environmental performance. The average lifetime and reliability of LED-lamps on a broad level is an unclear issue. | ||||
▪ | A number of studies compare de facto wattages, and electricity mixes, due to the applied assessment method. The “greener” the electricity production, the more significant are the manufacturing impacts, which are shifted to Asia. | ||||
▪ | All studies differentiate between regional electricity mixes and their environmental impacts differ by the factor 5. The efficiency and the emissions to air of a fossil power plant, depend on the simultaneous thermal use and the standard of flue gas cleaning. These data strongly influence the environmental impact of the use phase. | ||||
▪ | The electronic ballasts and control systems of light sources are not always fully considered, which leads to unclear comparative results. | ||||
▪ | Some studies are not clear in the distinction of primary energy consumption, medium voltage energy consumption, and end-energy consumption. One study declares the conversion factor by 3.3 from primary energy to end-energy, but this factor depends on the regional energy supply. | ||||
▪ | The continuous increasing of lighting control systems becomes a not negligible factor in the using phase. For 2016 it is estimated at 5.6% of the general lighting market and has a growth rate of 20% per year. | ||||
▪ | It is recommended to provide additionally the final electricity consumption of the manufacturing processes for better comparison with other studies, due to different conversion factors of the considered energy mixes. |
Waste management
▪ | Waste management is neglected or standardized to European standards and should be further explored for other regions. A global environmental assessment need additional scenario settings, which include realities of standards, emissions, e-waste handling, and lighting habits, dependent on the (geographical) location. | ||||
▪ | LED-lamps are classified as hazardous waste in Europe and some regions in the USA. They are categorized to waste electronics and not to waste lamps, which have a lower recycling rate than lamps. Depending on the region, the waste management in Europe tends to recycling and incinerating, and in the USA the waste tends to be landfilled. In many regions of Asia and Africa electronic waste is treated by unhealthy recycling or uncontrolled landfilling, where the impacts are not reflected in the life cycle assessment studies. |
Health effects, effects on animals, light pollution
▪ | Streamlined life cycle assessment methods do not consider damage effects on the eyes and the circadian rhythm, due to their predefined boundary conditions. The study by the International Energy Agency states that LED lamps have no worse health effects than other lamp types (IEA, Citation2014). The study conducted by Jaadane et al. investigated retinal damage induced by commercial white and blue LED-lamps with different wave lengths on rats (Jaadane et al., Citation2015). The lamp distance was 25 cm and the exposure time was 6 to 72 hr. | ||||
▪ | An article published in Neuroscience compared in a very controlled manner the effects of different light sources. It was found that white, blue, and green LEDs provoke retinal damage while CFL and FL lamps do not. The authors highlight once more the toxicity of blue light and particularly of blue-LEDs (Krigel et al., Citation2016). | ||||
▪ | The article by Hecht summarizes findings by different scientists. There is evidence that the increasing blue content of light can worsen the biological impact on humans and wildlife. The eye has nonvisual receptors for blue light, which controls the diurnal cycles, that is, the sleep and wake rhythm. The impact of LED-lamps on the circadian rhythms is five times greater than on conventional street lamps. The negative impacts on bugs, bats, birds, and sea turtles are emphasized (Hecht, Citation2016). | ||||
▪ | The study by Zissis conducted a standardized light exposure test and found, that cold white LEDs with a luminous flux of 200 lm have a moderate eye damage risk by a maximum exposure time of 40 to 100 sec (Zissis, Citation2016). Warm white LEDs have no risk at 100 lm and 200 lm, and a low eye damage risk at a luminous flux of 500 lm at an exposure time of 100 sec. | ||||
▪ | A further study summarizes LED lighting impacts on health, considering glare from LED lighting, optical hazards from LEDs, LED flicker, night-time exposure to LED lighting, as well as LED content of toxic chemicals, skin exposure to LED lighting, and electric fields generated by LED lighting (Ticleanu and Littlefair, Citation2015). | ||||
▪ | The study by Lin et al. developed a new model to estimate discomfort glare particularly of LED road lighting expressed by the deBoer rating with the aim to guide practical illumination designs. The model addresses the illuminance from an LED light source falling onto the drivers' eyes, the contrast between light source and background, and the installed position of the road light (Lin et al., Citation2014). Villa et al. investigated pedestrian discomfort glare from urban LED lighting. The findings confirmed previous studies, which recommend frosted or opalescent lamp covers to limit the maximum luminance and therefore the discomfort glare (Villa et al, Citation2016). | ||||
▪ | Light pollution is connected with excessive and misdirected street lighting on a global level. It is suspected to disrupt the ecosystem and lead to adverse health effects. The study by Luginbuhl et al. investigated the sky brightness arising from street lights, dependent on the color temperature at the same luminous flux (Luginbuhl et al., Citation2014). It was found, that the sky brightness arising from light sources with high blue proportion more strongly decreases with the distance, but the visual sky glow remains significantly brighter than from warm white light sources. | ||||
▪ | The study by Dale et al., (Citation2011) states that the city of San Diego has limited the maximum color temperature of street lamps to 3500 K. The latest recommendation regarding the maximum color temperature of outdoor LED-lamps is 3000 K (Hecht, Citation2016). |
Rebound effect
▪ | The setting of a life cycle assessment is not suitable to recognize the rebound effect phenomenon. Energy statistics show that in the last decade the electricity consumption of general lighting did not decrease by the expected values and instead has partially even increased, although efficient lighting has been introduced to the market. | ||||
▪ | The equalization of the luminous flux of different lamp technologies does not sufficiently define equal illumination. In case of street lighting, single LED-lamps provide poorer illumination and therefore they have to be installed with less distance (Tähkämö and Halonen, Citation2015). |
The life cycle assessment studies for LED-lamps provide a detailed and heterogenous overview on varying aspects to be considered for a high environmental performance. The studies also show that there is a number of environmental and health aspects, which are not included in the system boundary of a life cycle assessment. There is no general recommendation possible for an environmentally best lamp technology. It is recommended that in case of large lighting projects, new measurements of the particular situation should be conducted, considering the technological state of the market, the light pollution, and a consultation of the affected user, hereby referring to light impression, glaring, and color temperature.
Conclusion
The life cycle assessment studies showed that LED-lamps have most likely the best environmental performance for indoor and outdoor spot light applications. For all other applications, it is not possible to make a certain statement of the most environmental friendly lamp technology including LED-lamps with an approximate luminous efficacy < 110 lm/W. Furthermore, the current lifetime specifications for LED-lamps on the market significantly decreased.
In the last years, serious concerns have occurred concerning health effects of blue light exposure and the problem of glaring, which have been confirmed in several studies. These aspects are not in the boundary of a streamlined life cycle assessment. An approach to solve the problems of glaring and blue light exposure could be a combination of blue and red-emitting LEDs combined with a new color converter technology and frosted or opalescent lamp covers.
The study settings of the investigated LCA studies do not meet current standards. There is lack of research on LED-lamps with a luminous efficacy between 80 lm/W and 120 lm/W with shorter lifetime. Further lack of research is life cycle inventory data for high pure raw materials for LED-chip and color converter manufacturing.
Acknowledgments
The authors thank F. Reil for carefully reading and revising the manuscript.
Additional information
Funding
References
- Abdul Hadi, S., Al Kaabi, M. R., Al Ali, M. O., and Arafat, H. A. (2013). Comparative life cycle assessment (LCA) of streetlight technologies for minor roads in United Arab Emirates. Energy for Sustainable Development 17, 438–450.
- Bae, S. R., Choi, Y. G., Im, W. B., Lee, K. S., and Chung, W. J. (2013). Rare earth doped silicate–oxifluoride glass ceramics incorporating LaF3 nano–crystals for UV–LED color conversion. Optical Materials 35, 2034–2038.
- Baldé, C. P., Wang, F., Kuehr, R., and Huisman, J. (2015). The global e-waste monitor–2014. Bonn, Germany: United Nations University, IAS – SCYCLE.
- BAN. (2002). Exporting Harm the High–Tech Trashing of Asia (Video). Basel Action Network. http://www.ban.org/ ( 07.2016).
- Bare, J. C., Norris, G. A., Pennington, D. W., and McKone, T. (2002). TRACI The tool for the reduction and assessment of chemical and other environmental impacts. Journal of Industrial Ecology 6(3–4), 49–78.
- Baumann, H., and Tillman, A. M. (2004). The Hitch Hiker's guide to LCA: An orientation in life cycle assessment methodology and applications. Lund, Sweden: Studentlitteratur AB.
- Bennich, P., Borg, N., and Scholand, M. (2015). IEA 4E SSL annex: Providing governments with the tools to accelerate market adoption of SSL products. Journal of Solid State Lighting 2, 2, DOI 10.1186/s40539-015-0021-1.
- Binnemans, K., Jones, P. T., Blanpain, B., Van Gerven, T., Yang, Y., Walton, A., and Buchert, M. (2013). Recycling of rare earths: A critical review. Journal of Cleaner Production 51, 1–22.
- Cangeloso, S. (2012). LED lighting: A primer to lighting the future. Sebastopol, California: O'Reilly.
- Carnegie Mellon University, Booz Allen Hamilton. (2010). Life–cycle energy consumption of solid-state lighting. Pittsburgh, Pennsylvania, USA: Carnegie Mellon University.
- Chang, M.-H., Das, D., Varde, P. V., and Pecht, M. (2012). Light emitting diodes reliability review. Microelectronics Reliability 52, 762–782.
- Chang, Y.-Y., Glorieux, B., Hsu, C.-H., Sun, C.-C., Yu, L.-Z., Yang, T.-H., and Chung, T.-Y. (2016). Influence of ZrO2particles on the optical properties of pc-LEDs. Optical Materials 55, 55–61.
- China-RoHS. (2006). AeA-Advancing the Business of Technology. Industrial Standard of the People's Republic of China SJ/T 11365–2006.
- Chung, P. T., Yang, C. T., Wang, S. H., Chen, C. W., Chiang, A. S. T., and Liu, C.-Y. (2012). ZrO2/epoxy nanocomposite for LED encapsulation. Materials Chemistry and Physics 13, 868–876.
- Commission Regulation (EC). (2009). Commission Regulation (EC) No 244/2009 of 18 March 2009 implementing Directive 2005/32/EC of the European Parliament and of the Council with regard to ecodesign requirements for non-directional household lamps.
- Dale, A. T., Bilec, M. M., Marriott, J., Hartley, D., Jurgens, C., and Zatcoff, E. (2011). Preliminary comparative life–cycle impacts of streetlight technology. Journal of Infrastructure Systems 17(4), 193–199.
- De Almeida, A., Santos, B., Paolo, B., and Quicheron, M. (2014). Solid state lighting review – Potential and challenges in Europe. Renewable and Sustainable Energy Reviews 34, 30–48.
- De Boer, R. (2011). Wissenswertes über LED. TKL Licht GmbH. http://tkl.ch/tkl/schulungsunterlagen_led.pdf ( 07.2016)
- Directive 2009/125/EC of the European Parliament and of the Council. (2009). Directive 2009/125/EC of the European Parliament and of the Council of 21 October 2009 establishing a framework for the setting of ecodesign requirements for energy–related products (recast).
- Dong, J., Lei, W., Cai'e, C., Yue, T., and Ping, H. (2015). Luminescence properties of Ce3+–doped and Ce3+–Tb3+ co–doped Na0.34Ca0.66Al1.66Si2.34O8 phosphor for UV–LED. Ceramics International 41, 1341–1346.
- Dupuis, R. D., and Krames, M. R. (2008). History, development, and applications of high-brightness visible light emitting diodes, Journal of Lightwave Technology 26, 1154–1171.
- EAK Elektroaltgeräte Koordinierungsstelle Austria GmbH. (2015). Personal communication.
- Jungbluth, N., Stucki, M., and Frischknecht, R. (2009). Photovoltaics. In R. Dones et al. (Ed.), Sachbilanzen von Energiesystemen: Grundlagen für den ökologischen Vergleich von Energiesystemen und den Einbezug von Energiesystemen in Ökobilanzen für die Schweiz. Ecoinvent report No. 6–XII. Dübendorf, CH: Swiss Centre for Life Cycle Inventories.
- ERECON. (2015). (eds.). Strengthening the European rare earths supply chain: Challenges and policy options. Kooroshy, J., G. Tiess, A. Tukker, and A. Walton A Report by the European Rare Earths Competency Network (ERECON).
- En.lighten. (2016). En.lighten: United Nations Environment Programme (UNEP)–Global Environment Facility (GEF) initiative; Global lighting map. http://map.enlighten-initiative.org/ ( 04.02.2016)
- Franz, M., and Nicolics, J. (2015). Environmental aspects of white LED lighting systems: Energy statistics, study parameters, rare earths. Proceedings of the 38th Int. Spring Seminar on Electronics Technology. IEEE Xplore, 396–402.
- Fraunhofer/IZT. Angerer, G., Marscheider-Weidemann, F., Lüllmann, A., Erdmann, L., Scharp, M., Handke, V., and Marwede, M. (2009). Rohstoffe für zukunftstechnologien. Einfluss des branchenspezifischen Rohstoffbedarfs in rohstoffintensiven Zukunftstechnologien auf die zukünftige Rohstoffnachfrage. Stuttgart, Germany: Fraunhofer IRB Verlag.
- Friedel, R., Spindler, E. A. (Eds.) (2016). Zertifizierung als erfolgsfaktor: nachhaltiges wirtschaften mit vertrauen. Wiesbaden, Germany: Springer Gabler.
- Fulmek, P., Nicolics, J., Nemitz, W., Schweitzer, S., Sommer, C., Hartmann, P., Schrank, F., and Wenzl, F. P. (2016). The impact of the thermal conductivities of the color conversion elements of phosphor converted LEDs under different current driving schemes. Journal of Luminescence 169, 559–568.
- Fulmek, P., Sommer, C., Hartmann, P., Pachler, P., Hoschopf, H., Langer, G., Nicolics, J., and Wenzl F. P. (2013). On the thermal load of the color conversion elements of phosphor based white light emitting diodes, Advance Optical Material 1, 753–762.
- GaBi. (2016). Life cycle assessment software. http://www.gabi-software.com/ ( 10/2016)
- Gassmann, A., Zimmermann, J., Gauß, R., Stauber, R., and Gutfleisch, O. (2016). LED Lamps Recycling Technology for a Circular Economy. LED professional Review. Trends & Technologies for Future Lighting Solutions, July/August 2016, Issue 56, pp. 74–81.
- GE Lighting. (2011). GE CMH chromafit lamps. Boston, Massachusetts, USA: General Electric Company.
- George, N. C., Denault, K. A., and Seshadri, R. (2013). Phosphors for Solid-state white lighting. Annual Review of Materials Research 43, 481–501.
- Grandell, L., Lehtilä, A., Kivinen, M., Koljonen, T., Kihlman, S., Lauri, and L. S.. (2016). Role of critical metals in the future markets of clean energy technologies. Renewable Energy 95, 53–62.
- Greenpeace. (2012). Greener Electronics. http://www.greenpeace.org/ ( 07.2016).
- Gunn, G. (Ed.) (2014). Critical metals handbook, New York: Wiley.
- Han, J. K., Choi, J. I., Piquette, A., Hannah, M., Anc, M., Galvez, M., Talbot, J. B., and McKittrick, J. (2013). Phosphor development and integration for near-UV LED solid state lighting, ECS Journal of Solid State Science and Technology 2, R3138–R3147.
- Hartley, D., Jurgens, C., and Zatcoff, E. (2009). Life cycle assessment of streetlight technologies. Mascaro Center for Sustainable Innovation, University of Pittsburgh, Pittsburgh, Pennsylvania, USA, 72 p. http://www.pitt.edu/news2010/Streetlight_Report.pdf ( 07.2016).
- Hecht, J. (2016). The early–adopter blues. pp. 44–50. North American: Spectrum IEEE.
- Hendrickson, C. T., Matthews, D. H., Ashe, M., Jaramilloand, P., and McMichael, F. C. (2010). Reducing environmental burdens of solid–state lighting through end–of–life design. Environmental Research Letters 5, 014016.
- Hischier, R., Classen, M., Lehmann, M., and Scharnhorst, W. (2007). Life cycle inventories of electric and electronic equipment: Production, use and disposal. Data v2.0. Ecoinvent report No. 18, St. Gallen / Dübendorf. http://www.ecoinvent.org/
- IEA International Energy Agency. (2006). Light's labour's lost. Policies for energy–efficient lighting. Paris, France: International Energy Agency (IEA).
- IEA International Energy Agency. (2014). Life cycle assessment of solid state lighting, Solid state lighting annex. France: International Energy Agency 4E Solid State Lighting Annex.
- India-RoHS. (2014). Government of India, Ministry of Environment, Forests & Climate Change HSM Division. No. 12–85/2014–HSMD. Guidelines for implementation of Reduction in the use of Hazardous Substances (RoHS) provision as prescribed in Rule 13 of e–waste Rules.
- ISO 14040. (2006a). Environmental management – Life cycle assessment – Principles and framework.
- ISO 14044. (2006b). Environmental management – Life cycle assessment – Requirements and guidelines.
- Jaadane, I., Boulenguez, P., Chahory, S., Carre, S., Savoldelli, M., Jonet, L., and Behar-Cohen, F. (2015). Retinal damage induced by commercial light emitting diodes (LEDs). Free Radical Biology and Medicine 84, 373–384.
- JRC (Joint Research Centre).. (2008). European reference life cycle database (ELCD), version 2.0. http://lca.jrc.ec.europa.eu/ ( 07.2016)
- Jungbluth, N., Stucki, M., and Frischknecht, R. (2009). Photovoltaics. In R. Dones et al. (Ed.), Sachbilanzen von Energiesystemen: Grundlagen für den ökologischen Vergleich von Energiesystemen und den Einbezug von Energiesystemen in Ökobilanzen für die Schweiz. Ecoinvent report No. 6–XII. Dübendorf, CH: Swiss Centre for Life Cycle Inventories.
- Jüstel, T. (2013). Optimale Leuchtstoffe für LED-Applikationen. 9. Tagung: LED in der Lichttechnik, Essen, 12.–13. March 2013. Germany: Münster University of Applied Sciences.
- Khanna, V. (2014). Fundamentals of solid-state lighting: LEDs, OLEDs, and their applications in illumination and displays. USA: CRC Press.
- Kingsnorth, D. J. (2012). Centre for Research in Energy and Minerals Economics, Curtin University, Western Australia, and Industrial Minerals Company of Australia Pty Ltd (IMCOA). The Global Rare Earths Industry: A Delicate Balancing Act. Berlin: Deutsche Rohstoffagentur.
- Klöpffer, W., and Grahl, B. (2009). Ökobilanz (LCA): Ein Leitfaden für Ausbildung und Beruf. Wiley–VCH. ( English version: Life cycle assessment (LCA): A guide to best practice. Wiley–VCH Verlag GmbH & Co. KGaA, Weinheim.
- Kölper, C., Bergbauer, W., Strassburg, M., Linder, N. (2011). Die Licht(r)evolution. Weiße LEDs für die Allgemeinbeleuchtung. Phys. Unserer Zeit. Weinheim: Wiley-VCH Verlag GmbH & Co. KGaA., p. 92–98.
- Koltun, P., and Tharumarajah, A. (2014). Life cycle impact of rare earth elements. London, UK: Hindawi Publishing Corporation ISRN Metallurgy.
- Krigel, A., Berdugo, M., Picard, E., Levy-Boukris, R., Jaadane, I., Jonet, L., Dernigoghossian, M., Andrieu-Soler, C., Torriglia, A., and Behar-Cohen, F. (2016). Light-induced retinal damage using different light sources, protocols and rat strains reveals LED phototoxicity. Neuroscience 339, 296–307.
- Kübler, R. (2009). Der perfekte Schnitt. Mediendienst der Fraunhofer–Gesellschaft Nr. 8–2009.
- LEDinside, a Business Division of TrendForce Corp. (2014). Global LED lighting market to reach US $25.7 billion in 2015, November. 6, 2014 – 18:14 – likchinlow. http://www.ledinside.com/intelligence/2014/11/global_led_lighting_market_to_reach_us_25_7_billion_in_2015 (02.05.2016).
- Li, J., Yan, J., Wen, D., Khan, W. U., Shi, J., Wu, M., Su, Q., and Tanner, P. A. (2016). Advanced red phosphors for white light-emitting diodes. Journal of Materials Chemistry C 4, 8611–8623.
- Lin, Y., Liu, Y., Sun, Y., Zhu, X., Lai, J., and Heynderickx, I. (2014). Model predicting discomfort glare caused by LED road lights. Optics Express 22, 18056.
- Lim, S. R., Kang, D., Ogunseitan, O. A., and Schoenung, J. M. (2011). Potential environmental impacts of light–emitting diodes (LEDs): Metallic resources, toxicity, and hazardous waste classification. Environmental Science & Technology 45, 320–327.
- Lim, S. R., Kang, D., Ogunseitan, O. A., and Schoenung, J. M. (2013). Potential environmental impacts from the metals in incandescent, compact fluorescent lamp (CFL), and light-emitting diode (LED) bulbs. Environmental Science & Technology 47, 1040–1047.
- Liu, L., Tan, X., Teng, D., Wu, M., and Wang, G. (2015). Simultaneously enhancing the angular-color uniformity, luminous efficiency, and reliability of white light-emitting diodes by ZnO@SiO2 modified silicones. IEEE Transactions on Components, Packaging and Manufacturing Technology 5, 599–605.
- Luginbuhl, C. B., Boley, P. A., and Davis, D. R. (2014). The impact of light source spectral power distribution on sky glow. Journal of Quantitative Spectroscopy & Radiative Transfer 139, 21–26.
- Market survey October. (2016). Short consumer market survey about retrofit LED–lamps in Amazon.de (Osram/Philips) and IKEA.
- Matthews, D. H., Matthews, H. S., Jaramillo, P., and Weber, C. L. (2009). Energy consumption in the production of high-brightness light-emitting diode, 2009. IEEE International Symposium on Sustainable Systems and Technology, 1–6. DOI: 10.1109/ISSST.2009.5156691.
- McKinsey & Company: Baumgartner, T., Wunderlich, F., Jaunich, A., Sato, T., Bundy, G., Grießmann, N., Kowalski, J., Burghardt, S., and Hanebrink, J. (Eds.) (2012). Lighting the way: Perspectives on global lighting market. Second edition. New York, USA: McKinsey & Company.
- McKittrick, J., Hannah, M. E., Piquette, A., Han, J. K., Choi, J. I., Anc, M., Galvez, M., Lugauer, H., Talbot, J. B., and Mishra, K. C. (2013). Phosphor selection considerations for near-UV LED solid state lighting. ECS Journal of Solid State Science and Technology 2, R3119–R3131.
- McKittrick, J., and Shea-Rohwer, L. E. (2014). Down conversion materials for solid-state lighting. Journal of the American Ceramical Society 97, 1327–1352.
- Mehr, M. Y., van Driel, W. D., and Zhang, G. Q. (2015). Progress in understanding color maintenance in solid-state lighting systems. Engineering 1, 170–178.
- Mont, F. W., Kim, J. K., Schubert, M. F., Schubert, E. F., and Siegel, R. W. (2008). High-refractive-index TiO2-nanoparticle-loaded encapsulants for light-emitting diodes. Journal of Applied Physics 103, 083120.
- Nakamura, S. (2015). Background story of the invention of efficient blue InGaN light emitting diodes (Nobel Lecture). Annalen der Physik 527, 335–349.
- Navigant Consulting Europe, Ltd.. (2009). Life–cycle assessment of ultra–efficient lamps. A research report completed for the Department for Environment, Food and Rural Affairs. Department for Environment, Food and Rural Affairs DEFRA, London, UK.
- Navigant U.S. Department of Energy. (2012). Life–cycle assessment of energy and environmental impacts of LED lighting products. Part I–II. U.S. Department of Energy, Washington D.C., USA.
- Neslen, A. (2015). Big brands ‘cheating’ consumers with false lightbulb efficiency claims. The Guardian, Thursday 17 December, 12.44 GMT (online). (04.02.2016)
- Osram, Siemens Corporate Technology. (2009). Life-cycle assessment of illuminants – A comparison of light bulbs, compact fluorescent lamps and LED lamps, Executive Summary. Osram Opto Semiconductiors GmbH, Regensburg, Germany.
- Park, H. A, Yl Kwon, L., Won, B. I., Jong, H., and Woon, J. C. (2015). Phosphor in glass with Eu3+ and Pr3+–doped silicate glasses for LED color conversion. Optical Materials 41, 67–70.
- Patent. (2010). Lichtquelle mit einem lichtemittierenden Element. Light source comprising a light–emitting element. EP 1 352 431 B1.
- Patent. (2014). Led light and filament thereof. US 20140369036 A1.
- Pavel, C. C., Marmier, A., Tzimas, E., Schleicher, T., Schueler, D., Buchert, M., and Blagoeva, D. (2016). Critical raw materials in lighting applications: Substitution opportunities and implication on their demand. Physics Status Solidi A213, 2937–2946.
- Pradal, N., Chadeyron, G., Potdevin, A., Deschamps, J., and Mahou, R. (2013). Elaboration and optimization of Ce–doped Y3Al5O12 nanopowders dispersion. Journal of the European Ceramic Society 33, 1935–1945.
- Principi, P., and Fioretti, R. (2014). A comparative life cycle assessment of luminaires for general lighting for the office – compact fluorescent (CFL) vs Light Emitting Diode (LED) – a case study. Journal of Cleaner Production 83, 96–107.
- Quirk, I. (2009). Life-cycle assessment and policy implications of energy efficient lighting technologies. http://nature.berkeley.edu/classes/es196/projects/2009final/QuirkI_2009.pdf ( 07.2016)
- Raukas, M., Kelso, J., Zheng, Y., Bergenek, K., Eisert, D., Linkov, A., and Jermann, F. (2013). Ceramic phosphors for light conversion in LEDs. ECS Journal of Solid State Science and Technology 2, R3168–R3176.
- RoHS 2. (2011). European Commission: Directive 2011/65/EU of the European Parliament and the Council of 8 June 2011 on the restriction of the use of certain hazardous substances in electrical and electronic equipment (recast).
- Sangwan, K. S., Bhakar, V., Naik, S., and Andrat, S. N. (2014). Life cycle assessment of incandescent, fluorescent, compact fluorescent and light emitting diode lamps in an Indian scenario. Procedia CIRP 15, 467–472.
- Schmidt, M., and Häuslein, A. (Eds.) (1996). Ökobilanzierung mit Computerunterstützung. Produktbilanzen und betriebliche Bilanzen mit dem Programm Umberto. Berlin Heidelberg New York: Springer-Verlag, pp. 242.
- SimaPro. (2016). Life cycle assessment software. https://www.pre-sustainability.com/ ( 10/2016)
- Sommer, C., Reil, F., Krenn, J. R., Hartmann, P., Pachler, P., Hoschopf, H., and Wenzl, F. P. (2011). The impact of light-scattering on the radiant flux of phosphor-converted high power white light-emitting diodes. Journal of Lightwave Technology 29, 2285–2291.
- Tähkämö, L., Bazzana, M., Ravel, P., Grannec, F., Martinsons, C., and Zissis, G. (2013). Life cycle assessment of light–emitting diode downlight luminaire – a case study; International Journal of Life Cycle Assessment 18, 1009–1018.
- Tähkämö, L., and Halonen, L. (2015). Life cycle assessment of road lighting luminaires – Comparison of light–emitting diode and high–pressure sodium technologies. Journal of Cleaner Production 93, 234–242.
- Tan, S. T., Sun, X. W., Demir, H. V., and DenBaars, S. P. (2012). Advances in the LED Materials and Architectures for Energy-Saving Solid-State Lighting Toward “Lighting Revolution”. IEEE Photonics Journal 4(2), 613–619.
- Tang, Y., Zhou, S., Chen, C., Yi, X., Feng, Y., Lin, H., and Zhang, S. (2015). Composite phase ceramic phosphor of Al2O3-Ce:YAG for high efficiency light emitting. Optics Express 23(14), 17923.
- Ticleanu, C., and Littlefair, P. (2015). A summary of LED lighting impacts on health. International Journal of Sustainable Lighting 1, 5–11.
- U.S. Department of Energy DOE. (2016). Solid–State Lighting R & D Plan. June 2016. DOE SSL Program, “R&D Plan,” edited by James Brodrick, Ph.D.
- Villa, C., Bremond, R., Saint-Jacques, E. (2016). Assessment of pedestrian discomfort glare from urban LED lighting. Lighting Research Technology 1–26.
- Von Nauckhoff, M. (2011). Strategische metalle und seltene erden. Munich, Germany: Finanzbuch Verlag.
- Wang, L., Dong, J., Cui, C., Tian, Y., and Huang, P. (2015). Luminescence properties of a single-component Na0.34Ca0.66Al1.66Si2.34O8:Ce3+, Sm3+ phosphor with tunable color tone for UV–pumped LEDs. Optical Materials 46, 373–377.
- WEEE. (2012). European Commission: Directive 2012/19/EU of the European Parliament and of the Council of 4 July 2012 on waste electrical and electronic equipment (WEEE) (recast).
- Welz, T., Hischier, R., and Hilty, L. M. (2011). Environmental impacts of lighting technologies – Life cycle assessment and sensitivity analysis. Environmental Impact Assessment Review. 31(3), 334–343.
- Wenzl, F. P., Fulmek, P., Sommer, C., Schweitzer, S., Nemitz, W., Hartmann, P., Pachler, P., Hoschopf, H., Schrank, F., Langer, G., and Nicolics, J. (2014). Impact of extinction coefficient of phosphor on thermal load of color conversion elements of phosphor converted LEDs. Journal of Rare Earths 32, 201–206.
- Wilburn, D. R. (2012). Byproduct metals and rare-earth elements used in the production of light–emitting diodes – Overview of principal sources of supply and material requirements for selected markets. U.S. Geological Survey Scientific Investigations Report 5215, pp. 15.http://pubs.usgs.gov/sir/2012/5215/.
- Wolf, M. A., Pant, R., Chomkhamsri, K., Sala, S., and Pennington, D. (2012). The international reference life cycle data system (ILCD) handbook. JRC Reference Reports, European Union, Ispra, Italy.
- Ye, S., Xiao, F., Pan, Y. X., Ma, Y. Y., and Zhang, Q. Y. (2010). Phosphors in phosphor-converted white light emitting diodes: Recent advances in materials, techniques and properties. Materials Science and Engineering R71, 1–34.
- Yi, S., Chung, W. J., and Heo, J. (2015). Phosphor-in-glasses composites containing light diffusers for high color uniformity of white-light-emitting diodes. Journal of Solid State Lighting 2, 8.
- Zheng, H., Liu, S., Zou, X., Lei, X., and Guo, X. (2015). Improvement of optical performances of LEDs by dual–layer structure of nano–TiO2 and phosphor. 2015 IEEE 16th International Conference on Electronic Packaging Technology, pp. 1051–1053.
- Zissis, G. (2016). Quality and health aspects of led lamps for the european residential lighting market. LED professional Review. Trends & Technologies for Future Lighting Solutions, July/August 2016, Issue 56, pp. 40–56.