Abstract
Aquatic environments are identified as an ideal setting for acquisition and dissemination of antibiotic resistance, and human exposure to antibiotic resistant bacteria (ARB) and antibiotic resistance genes (ARGs) in aquatic environments may pose an additional health risk. Quantitative microbial risk assessment (QMRA) has been suggested as a suitable method to evaluate and quantify this health risk. However, information about the exposure to ARB and ARGs in aquatic environments is lacking for many scenarios and dose-response models regarding the ARB infections are not developed yet. This review summarizes the current knowledge regarding the ARB and ARGs in aquatic environments and highlights the challenging questions remaining to be answered to better forecast the health risks caused by ARB and ARGs in water environments. The questions include what are the missing information needed to quantify the human health risks caused by exposing to ARB and ARGs in aquatic environments? what are the suitable markers to evaluate the ARB/ARGs contamination in aquatic environments? how frequently do the ARG selection and propagation occur in aquatic environments? and are there any unknown hot spots? Studies on the above topics will contribute to better management of antibiotic resistance dissemination in water environments and its risks on human health.
Abbreviations | ||
3GC | = | 3rd generation cephalosporins |
ARB | = | Antibiotic resistant bacteria |
ARG | = | Antibiotic resistance gene |
CFU | = | Colony forming unit |
DBP | = | Disinfection by-products |
eDNA | = | Extracellular DNA |
EPS | = | Extracellular polymeric substances |
HGT | = | Horizontal gene transfer |
ISCR | = | Insertion sequence common region |
MAR | = | Multiple antibiotic resistant |
MIC | = | Minimum inhibitory concentration |
MGE | = | Mobile genetic elements |
MSW | = | Municipal solid waste |
QMRA | = | Quantitative microbial risk assessment |
VBNC | = | Viable but non-culturable |
WWTP | = | Wastewater treatment plant |
Graphical Abstract
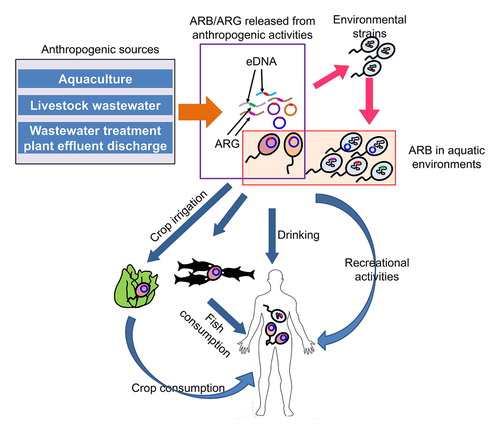
1. Introduction
Antibiotic resistance is the ability of bacteria to resist the effects of an antibiotic which they were previously sensitive. Bacteria become antibiotic resistant by either genetic mutations or by acquiring antibiotic resistance genes (ARGs). Antibiotic resistant bacterial (ARB) infections have higher mortality and morbidity rates and result in longer hospital stays (Cosgrove, Citation2006). ARB infections are estimated to cause 10 million deaths worldwide by year 2050 (O'Neill, Citation2016). Antimicrobial resistance poses a challenge in achieving the universal health coverage and hinder the achievement of sustainable development goals related to health, food security, clean water and sanitation (Interagency Coordination Group on Antimicrobial Resistance, Citation2019). In view of these facts, World Health Organization (WHO) published a list of antibiotic resistant priority pathogens. The list consists of 12 bacteria families which are considered to pose greatest threat to the human health (World Health Organization, Citation2017b). Since the drivers of antimicrobial resistance lie in humans, animals, plants, food and the environment, importance of One Health approach is emphasized in overcoming this threat (Interagency Coordination Group on Antimicrobial Resistance, Citation2019).
Aquatic environments are recognized as one of the reservoirs and transmission routes for the dissemination of antibiotic resistance (Karkman, Do, Walsh, & Virta, Citation2018; Suzuki, Pruden, Virta, & Zhang, Citation2017; Wellington et al., Citation2013). Drinking water and wastewater treatment processes are shown to be incapable of completely removing antibiotic resistance genes (ARGs) (Li, Cheng, Xu, Strong, & Chen, Citation2015; Pruden, Pei, Storteboom, & Carlson, Citation2006; Rodriguez-Mozaz et al., Citation2015). Wastewater treatment plant (WWTP) effluents containing ARB and ARGs can end up in aquatic environments like rivers and lakes. Usage of reclaimed wastewater in beneficial purposes like agricultural irrigation and recreational activities can introduce ARB and ARGs to the particular environment (Ben et al., Citation2017; Pruden et al., Citation2006; Rodriguez-Mozaz et al., Citation2015). Therefore, in addition to drinking, humans can be exposed to ARB and ARGs through activities like bathing, aquatic sports, occupational exposure during agricultural irrigation, and consumption of food produce irrigated with reclaimed water (Leonard, Yin, Zhang, Hui, & Gaze, Citation2018; Leonard, Zhang, Balfour, Garside, & Gaze, Citation2015; Leonard, Zhang, et al., Citation2018; O’Flaherty, Borrego, Balcázar, & Cummins, Citation2018; Søraas, Sundsfjord, Sandven, Brunborg, & Jenum, Citation2013).
However, potential human health risks caused by exposing to ARB and ARGs present in aquatic environments have not been completely evaluated yet, because specific information like the dose-response curves and exposure assessment data related to ARB and ARGs in different water usage scenarios needed to conduct a quantitative microbial risk assessment (QMRA) are scarce (Ashbolt et al., Citation2013; Pepper, Brooks, & Gerba, Citation2018). This review summarizes the information reported in recent studies on the human health risk assessment of ARB and ARGs, and highlight the required additional information to perform a refined QMRA for different scenarios. We further suggest important research questions related to the occurrence, proliferation and dissemination of ARGs and ARB in aquatic environments which needs to be answered for a better evaluation of human health risks caused by ARB and ARGs in aquatic environments.
2. Sources of antibiotics, ARB and ARG in water environments
2.1. Freshwater and wastewater related environments
Human and animal microbiota is a reservoir for ARGs (Salyers, Gupta, & Wang, Citation2004). Bacteria passing through the intestinal tract can acquire antibiotic resistance via conjugation and end up in human and animal feces (Anderson, Whitlock, & Harwood, Citation2006; Salyers et al., Citation2004). Consumption of antibiotics can provide a selective pressure (a biotic or abiotic factor which alters the behavior and fitness of an organism in a given environment) inside the gut, leading to the development of antibiotic resistance in enteric bacteria. Penicillin resistance was developed in dairy calves fed with milk containing penicillin G (0–50 µl/kg) in a dose dependent manner and persisted over the course of the experiment (Langford, Weary, & Fisher, Citation2003). Chicken manure compost collected from farms which use amoxicillin, kanamycin, gentamicin and cephalexin contained ARB between 21% and 66% of the total bacterial isolates. Among the ARB isolates, 8–20% were resistant to multiple antibiotics (Q. Yang et al., Citation2014).
Wastewater or other biological substances generated in hospitals, farms, homes and other facilities contain antibiotics, ARBs and ARGs. According to a recent study, tylosin administered sheep excreted the antibiotic in feces and urine up to four days. On average 11% of the administered tylosin was discharged in feces and urine (Ishikawa et al., Citation2018). A recent metagenomics analysis performed in 79 wastewater treatment plants (WWTPs) located in 60 countries reported that abundance and diversity of ARGs have differences between Asia/Africa/South America and North America/Europe and Oceania. North America/Europe and Oceania cluster contained limited number of ARGs encoding macrolide resistance in high abundance while Asia/Africa/South America cluster contained ARGs related to sulfonamides and phenicols. The study also reported Vietnam, India and Brazil having the most divergent ARG distribution and suggested them as possible hotspots for the emergence of new antibiotic resistance mechanisms (Hendriksen et al., Citation2019). provides a representative summary of ARGs and mobile genetic elements (MGEs) detected in different water environments.
Table1. Examples on various ARGs, MGEs and antibiotics found in different water environments.
Wastewater treatment plants (WWTPs) receive microbial and chemical loads from different sources. Inside WWTPs, under the selection pressure of antibiotics, metals, and other chemical substances, horizontal gene transfer (HGT) can occur (Karkman et al., Citation2018). Many studies have confirmed that WWTPs are not capable of completely removing antibiotics, ARB and ARGs (Ben et al., Citation2017). Therefore, WWTP effluent discharge to environments including surface water, groundwater, marine environments and soil where environmental microorganisms can interact with ARB and ARGs can lead to development of antibiotic resistance in the microorganisms from those environments. Detection of antibiotics, ARGs, and ARB in different aquatic environments are summarized in .
2.2. Marine environments
Occurrence of antibiotics, ARB and ARGs in marine environments can have different mechanisms than the fresh water and wastewater. According to a recent study, only 28% of the ARGs identified in marine environments were previously known (Hatosy & Martiny, Citation2015). Coastal runoff of ARB from terrestrial sources has been identified as one mechanism for antibiotic resistance occurrence in marine environments (Hatosy & Martiny, Citation2015). Secondly, anthropogenic antibiotic runoff related selection can occur in marine aquaculture related activities. For example, Chilean marine salmonid farms have used 363.4 tons of antibiotics in 2016 alone which can act as a selective pressure for antibiotics resistance development in marine environments (Miranda, Godoy, & Lee, Citation2018). According to a metagenomic study on the intestinal contents of fish from the farms located in Baltic Sea, ARGs found in fish intestines were same as the ARG enriched in farm sediments, suggesting fish feces contributes to the ARG enrichment even when there are no concurrent antibiotic treatments. Usage of antibiotics during hatching and rearing of juvenile fish before entering the sea farms is suggested as a possible reason for the presence of ARGs in fish intestines. However, it was also suggested the possibility of transferring ARG derived in water environment to the fish intestine (Muziasari et al., Citation2017). Selection for the antibiotic resistance as a result of the generation of antibiotics in marine environments by marine microorganisms is the third mechanism. (Baam, Gandhi, & Freitas, Citation1966; Rosenfeld & Zobell, Citation1947; Tortorella et al., Citation2018). Among 58 marine microorganisms tested by Rosenfeld & Zobell (Citation1947), 4 Bacillus species, 3 Micrococcus species, one Actinomyces and Serratia species demonstrated the antibiotic activity against non-marine forms. Among them, Bacillus and Micrococcus were the most active antagonistic marine genera (Rosenfeld & Zobell, Citation1947). Fluoroquinolone resistance (qnr) genes, qnrA, qnrB, qnrS, are thought to have originated from water-dwelling bacterial chromosome (Takasu, Suzuki, Reungsang, & Viet, Citation2011), which are transferred to other species. Recently discovered sulfonamide resistance gene (sul4) from Indian river bacteria is accompanied by class 1 integrons (Razavi et al., Citation2017). Thus, some ARGs are originated in aquatic environment and might have invaded to human environment.
In conclusion, major sources of ARB and ARGs should be human and veterinary clinical settings where intestinal bacteria are exposed to high concentrations of antibiotics, WWTPs, and related land wastes. However, recent reports presenting global antibiotic contamination in water environments (Fekadu, Alemayehu, Dewil, & Van der Bruggen, Citation2019; Segura et al., Citation2015; Shimizu et al., Citation2013) suggest development of ARB in water environments including open oceans (Hatosy & Martiny, Citation2015). Bacteria can evolve to be antibiotic resistant via mutations at very low antibiotics concentrations in natural aquatic environments (100-fold below minimum inhibitory concentration (MIC)) (Kohanski, DePristo, & Collins, Citation2010). Even though the fraction of resistant mutants is very low (10−4), selection of ARB can occur over generations. Sub-MIC selection contributes to prolonged persistence of ARB since low concentrations of antibiotics are enough to maintain the existing resistant bacteria (Gullberg et al., Citation2011) and attention should be paid on water environments as an ARB and ARGs risk origin.
3. Acquisition of antibiotic resistance by horizontal gene transfer and mutation
3.1. Antibiotic resistance acquisition via horizontal gene transfer (HGT)
Antibiotic resistance of environmental bacteria can be intrinsic, acquired via spontaneous mutations (de novo) or horizontal gene transfer (HGT) (J. Davies & Davies, Citation2010; Sharma, Johnson, Cizmas, McDonald, & Kim, Citation2016). Antibiotic resistance hotspots are the locations where susceptible bacteria can develop antibiotic resistance and disseminate (Marti, Variatza, & Balcazar, Citation2014). Horizontal gene transfer can occur through transformation, transduction and conjugation (Aminov, Citation2011).
It is shown that as high as 3% of the Pseudomonas aeruginosa genome may contribute to intrinsic resistome (Fajardo et al., Citation2008; Martinez, Citation2014). Spontaneous mutation rate of ciprofloxacin and rifampin resistance in Helicobacter pylori was 1 × 10−8 to 2 × 10−8 per cell per division (G. Wang, Wilson, Jiang, & Taylor, Citation2001).
In the transformation process, bacteria will uptake and integrate the extracellular DNA present in the environment (Thomas & Nielsen, Citation2005). Environmental ARG pool is formed under grazing pressure by protists, which is found to be constant (Bien, Thao, Kitamura, Obayashi, & Suzuki, Citation2017). A large number of naturally transformable prokaryotes in the environment have been reported (de Vries & Wackernagel, Citation2005; Johnsborg, Eldholm, & Håvarstein, Citation2007; Lorenz & Wackernagel, Citation1994). Natural transformation of Pseudomonas stutzeri strains isolated from soil and marine sediments to rifampicin resistance by chromosomal DNA have been observed (Stewart & Sinigalliano, Citation1991). Transformation of naturally competent Acinetobacter calcoaceticus by plasmid and chromosomal DNA adsorbed on sand and ground water/ground water aquifer microcosms was observed; chromosomal DNA transformation frequency was 103-104 times higher than plasmid DNA transformation frequency (Chamier, Lorenz, & Wackernagel, Citation1993). Transformation of environmental bacteria found in other aquatic environments like river water, spring water and groundwater have also been reported (Davison, Citation1999).
In the transduction process, cellular DNA packaged to the bacteriophages during their replication will be incorporated to the host bacterial genome after the infection by a transduced bacteriophage (Calero-Cáceres, Ye, & Balcázar, Citation2019). Extended spectrum beta-lactamase (ESBL) genes blaTEM, blaCTX-M, blaCMY-2, blaKPC, blaOXA-48, blaPSE, conferring resistance to β-lactam antibiotics were detected in DNA of bacteriophages isolated from canal water, urban sewage and river water samples collected from Washington State whereas blaNDM-1 gene was detected from bacteriophage DNA fraction isolated from river and wastewater samples (A. Zhang et al., Citation2019). Analysis of viromes from seven marine habitats including Arctic Ocean, Indian Ocean and Gulf of Mexico confirmed the presence of phage associated ARGs (Calero-Cáceres & Balcázar, Citation2019). Muniesa et al. (2013) have provided a detailed review on the contribution of environmental bacteriophages on spreading ARGs (Muniesa, Colomer-Lluch, & Jofre, Citation2013b).
During the conjugation process, MGEs like plasmids, transposons, integrons, insertion sequences, insertion sequence common region (ISCR) elements, genomic islands and integrating conjugative elements (ICE) are moved from the donor cell to the recipient cell (Marti et al., Citation2014).
3.2. Antibiotic resistance development by mutagenesis
Mutagenesis can lead to development of antibiotic resistance in environmental bacteria via antibiotic target alteration, increased drug efflux, gene amplification, reduced target expression and drug modification enzyme alteration (Andersson & Hughes, Citation2010). Mutagenic disinfectant by-products (DBPs), antibiotics, non-antibiotic microbials, antidepressants and biofilms have shown to play an important role in this regard (Ford, Citation2006; Jin et al., Citation2018; Lu, Jin, et al., Citation2018; Lv, Yu, Xu, & Ye, Citation2015; Savage, Chopra, & O'Neill, Citation2013). Sub-inhibitory concentrations of antibiotics can produce reactive oxygen species (ROS) which consequently induce mutagenesis. As a result, some mutant strains which are sensitive to the applied antibiotic may be resistant to other antibiotics (Kohanski et al., Citation2010). In the presence of DBPs at sub-inhibitory concentrations and near-inhibitory concentrations, resistance mechanism selections were different. Under high DBP concentrations, overexpressed efflux pump systems, impermeability of the membrane and depletion of replication error repair protein leading to increased mutation rate were the main mechanisms. Under low NaClO2 and IAA concentrations, substitution mutations in gyrA and deletion mutation in proS likely to contribute to ciprofloxacin resistance development (Li, Zeng, He, & Gu, Citation2016). After exposing to mutagenic DBPs, P. aeruginosa PAO1 and E. coli K12 displayed increased resistances to individual and multiple antibiotics (Lv, Jiang, Zhang, & Yu, Citation2014; Lv et al., Citation2015). E. coli K12 exposed to triclosan (0.2 mg/l) for 30 culture cycles induced oxidative stress leading to the development of mutations in major genes like frdD, marR, acrR and soxR. These mutations cause overexpression of adjoining genes or enhanced the expression of repressed genes like ampC, acrAB, soxS and marAB. This may have initiated the translation of AmpC and multidrug efflux pump AcrAB inducing multiple antibiotic resistance (MAR) (Lu, Jin, et al., Citation2018; Lu, Wang, et al., Citation2018). Antidepressant fluoxetine overproduced ROS which lead to transcriptional regulator gene mutation. These mutations increased the expression of efflux pump system and decreased abundance of outer membrane protein OmpF leading to resistance development (Jin et al., Citation2018). Antibiotic resistance development by mutation at sub-inhibitory levels of different selection pressures are summarized in . Comprehensive reviews on the enhancement of antibiotic resistance of Gram-negative and Gram-positive bacteria caused by biocidal agents are available elsewhere (Kampf, Citation2018, Citation2019).
Table 2. Sub-inhibitory concentrations of selection pressures induce antibiotic resistance.
3.3. Selective pressures for the antibiotic resistance acquisition by HGT
Disinfectants, antibiotics, metals and DBPs in water and wastewater, even at low concentrations, can affect the HGT efficiency (Karkman et al., Citation2018) (). Rusin and Gerba reviewed the earlier studies on the development of antibiotic resistance by chlorination of water and wastewater (Rusin & Gerba, Citation2001). Disinfection with free chlorine at low doses (0.05–0.2 mg/l) didn’t affect the transfer efficiency of RP4 plasmid while high doses (0.3–0.5 mg/l) caused a decrease in conjugative transfer efficiency. Reduced cell viability due to cell damage was the major contribution factor (Lin, Li, Zhang, & Yu, Citation2016). Chlorination enriched six out of 125 ARGs and one out of 13 MGEs present in a municipal WWTP secondary effluent (Lin, Zhang, Zhang, & Yu, Citation2016).
Accumulation of metals like Hg, Zn, Cu and Cd have been recognized as a major factor for the co-selection of antibiotic resistance in aquatic environments (Seiler & Berendonk, Citation2012). Abundance of MAR bacteria in drinking water was increased from 20.4% to 36.4% upon entering the distribution system after chlorine percolation treatment. A significant proportion of MAR bacteria isolated from drinking water distribution systems displayed tolerance to different combinations of Cu2+, Pb2+ and Zn2+ where 20.9% of isolates were tolerant to all three metals. A positive association between MAR and metal tolerance was observed in the point of use compared to point of entry suggesting that bacteria became antibiotic resistant inside the galvanized iron and concrete pipes during the distribution (Calomiris, Armstrong, & Seidler, Citation1984). Presence of Cu2+ ions and CuO nanoparticles (1–100 µmol/l) enhanced the conjugative transfer efficiency of ARGs across bacterial genera. Cu2+ ions and CuO nanoparticles caused over production of reactive oxygen species (ROS). Increased ROS induced the SOS response as shown by the upregulation of DNA damage related genes which ultimately lead to the conjugative transfer of ARG. In addition, Cu2+ ions and CuO nanoparticles increased the conjugative transfer by increased expression of pilin production genes, increased cell membrane permeability and by conjugative regulator alteration leading to accelerated plasmid replication (Shuai Zhang et al., Citation2019). V and Ca accelerated HGT by conjugation in experimental and field studies (Suzuki, Kimura, Agusa, & Rahman, Citation2012). In an activated sludge plant, concentration of heavy metals was correlated with the enrichment of several ARGs because heavy metals can induce efflux pumps (Mao et al., Citation2015). There was a significant correlation (p < .05) between ARGs and heavy metals (Zn, Cu and Co) in MSW landfill groundwater (Yu, He, Shao, Zhang, & Lü, Citation2016). Cu and Cr concentrations in mariculture drainage ditch sediments were positively correlated with the presence of fluoroquinolone resistance gene acc(6)-lb-cr, β-lactamase resistance genes imp5, imp12 and qnrS (Z. Zhao et al., Citation2017).
Number of studies have shown that antibiotics can act as a selective pressure for the antibiotic resistance development in aquatic environments. Gentamicin and sulfamethoxazole significantly increased the transfer frequencies of antibiotic resistance at sub-inhibitory antibiotic concentrations (1/16 for sulfamethoxazole and right below the MIC for gentamicin) (Jutkina, Marathe, Flach, & Larsson, Citation2018). Remaining ARG concentrations in the effluent were significantly correlated with the remaining antibiotics concentrations in an activated sludge plant (p < .05) (Mao et al., Citation2015). Raw wastewater tetracycline concentration correlated with the antibiotic resistance prevalence in the treated effluent at high temperatures (Novo, André, Viana, Nunes, & Manaia, Citation2013). There were significant correlations between sulfamerizine concentration and the proportion of tetracycline resistance genes tet(B) and tet(C) genes in a wastewater treatment plant (Li et al., Citation2015). Conjugative transfer efficiency of pB10 plasmid with P. aeruginosa PAKexoT and activated sludge increased at ppb (µg/l) levels of tetracycline and sulfamethoxazole (Kim et al., Citation2014). Number of kanamycin resistance (Kr) genes acquired by the sediments lacking Kr genes after 15 days were significantly higher (p < .05) in the presence of kanamycin compared to the control condition which lacked kanamycin (Mao et al., Citation2014). In mesocosms treated with different sub-inhibitory oxytetracycline concentrations, tet(M) and transposon Tn916/1545 concentrations were correlated for all antibiotic concentrations and the selection patterns for total tet genes and Tn916/1545 relative to the oxytetracycline levels were also similar (Knapp et al., Citation2008).
O-xylene, ethylbenzene, trioxymethylene, styrene, 2,4-dichloroaniline and malachite green found in a mixed wastewater (Domestic and dyeing) promoted the conjugative transfer of RP4 plasmid from Escherichia coli HB101 to E. coli NK5449 by 4 to 200-fold compared to the control (Jiao, Chen, Gao, Zhu, & Rensing, Citation2017). A 60-fold increase in RP4 plasmid transfer efficiency was observed in the presence of ionic liquid [BMIm][PF6] (Wang, Mao, Mu, & Luo, Citation2015). Carbamazepine also promoted the HGT of plasmid-borne MAR genes in a similar manner to Cu2+ ions and CuO nanoparticles (Wang et al., Citation2019).
4. Removal/enrichment of ARB and ARGs in water supply systems and wastewater treatment unit processes
Water and wastewater treatment plants are designed according to the multiple-barrier concept where a stipulated pathogen log reduction is achieved by a combination of different treatment unit processes (Sano, Amarasiri, Hata, Watanabe, & Katayama, Citation2016). Several studies report the achievable log reductions for different pathogens by different treatment unit processes (Amarasiri, Kitajima, Nguyen, Okabe, & Sano, Citation2017; Sano et al., Citation2016). Observed log reductions had intra-process and interprocess variations depending on the pathogen of concern (Amarasiri et al., Citation2017). However, currently there are no stipulated minimum reductions for antibiotics, ARB and ARGs in water/wastewater treatment guidelines (Hong et al., Citation2018).
Membrane bioreactor treatment achieved significantly higher removals of ARB and ARGs (range of removal: 2.57–7.06 log10) compared to conventional treatment methods like activated sludge, rotating biological contactor or oxidation ditch (range of removal: 2.37–4.56 log10) (p < .05)(Munir, Wong, & Xagoraraki, Citation2011; O’Flaherty & Cummins, Citation2017). Sand filtration and sedimentation effectively removed ARGs from drinking water (Su et al., Citation2018). Retention in the sand filter medium with low nutrient conditions may have caused the ARB starvation and permanent loss of antibiotic resistance due to plasmid degradation (Griffiths, Moyer, Caldwell, Ye, & Morita, Citation1990; Tan et al., Citation2019). Drinking water treatment by conventional (flocculation + sedimentation + sand filtration + chlorination) and advanced (preozonation + flocculation + sand filtration + postozonation + Granular Activated Carbon + chlorination) treatment plants achieved 0.03-2.4 ARG log reductions. However, different ARGs displayed different log reduction values. For example, class 1 integron-integrase gene (intI1) had 2.4 log reduction by conventional process whereas advanced process provided 1.8 log reduction. Macrolide-lincosamide-streptogramin B (MLSB) resistance gene ermB removal by conventional process was negligible and advanced process achieved 1.1 log reduction. Flocculation + sedimentation step in the conventional treatment process contributed to the ARG reduction by the removal of ARB (Hu, Zhang, Jiang, Luo, et al., Citation2019).
Chromosomally located β-lactamase resistance gene mecA and plasmid-borne tet(A) genes (105 copies/µl) could be removed below detection limit using free chlorine. However, tetA was able to tolerate double the concentration of free chlorine compared to mecA and similar results were obtained with UV. Differences in the distribution of adjacent thymine sites which can rapidly react with free chlorine is suggested as a possible reason for the observation. By using short and long amplicon detection, the study also confirmed that free chlorine doesn’t entirely destroy ARGs (M. Zhang, Chen, Yu, Vikesland, & Pruden, Citation2019). A study on the antibiotics, ARB and ARGs reduction by peroxymonosulphate (PMS) oxidation, UV irradiation and UV/PMS combination treatment revealed that sulfonamide resistant bacterial strain HLS-6 can be reduced by 5.3 log using UVC (100 μW/cm2)/PMS (1 mg/L) treatment. HO・ and SO4・─ radicals generated by breaking the PMS peroxide bond under UV radiation was suggested to be responsible for ARB inactivation. However, a higher PMS dose of UVC/PMS (100 μW/cm2)/(20 mg/L) was needed to achieve 2.9 ± 0.1 log reduction of sul1 and 3.4 ± 0.1 log reduction of intI1 within 30 mins (Hu, Zhang, Jiang, Yao, et al., Citation2019). Even though ARB were degraded immediately in the presence of sulfamethoxazole, ARGs reduction was inhibited after some time. Competitive consumption of free radicals by sulfamethoxazole and ARGs was suggested as the possible reason (Hu, Zhang, Jiang, Yao, et al., Citation2019).
Many studies have shown that treatment processes are incapable of completely eliminating ARB and ARGs from source water. Furthermore, in some cases, WWTPs are shown to increase the abundance of specific ARGs in the plant effluent (Chu et al., Citation2018; Karkman et al., Citation2018; Rizzo et al., Citation2013; Jian Xu et al., Citation2015). According to a metagenomic study, there were no significant differences in the ARGs detected in potable source water and in the treated water at the extraction point confirming that ARGs were not removed during the drinking water treatment (Garner et al., Citation2018). Wastewater treatment lead to the reductions of ermB and tet(W) relative concentrations while relative concentrations of blaTEM, sul1 and qnrS were increased. Spread of some ARGs among bacterial cells in activated sludge was suggested as a plausible reason (Rodriguez-Mozaz et al., Citation2015). Granular activated carbon filtration enhanced the abundance of ARGs in the filtered water. Formation of biofilms on the biological activated carbon surfaces where antibiotics and ARB can adhere to and grow is suggested as the possible reason. (Su et al., Citation2018; Tan et al., Citation2019; Xu et al., Citation2016). Twelve ARGs were discharged through the dewatered sludge and plant effluent at higher rates than influent values from two municipal WWTPs, indicating overall proliferation of resistant bacteria. There were statistically significant correlations (p < .05) between the effluent ARG concentration and residual antibiotic concentration, As, Zn, Pb and Hg concentration, suggesting the selective pressure for some ARG enrichment (Mao et al., Citation2015). Chlorination and ozone treatment increased the propagation of some ARGs in conventional and advanced drinking water treatment plants as a result of resistance development due to mutagenesis and cell damage repair mechanisms (Hu, Zhang, Jiang, Luo, et al., Citation2019).
In addition to WWTPs, sewer pipes containing inner surface biofilms are suggested as possible hotspots for accumulation and dispersal of ARGs. Auguet et al. (Citation2017) observed no significant differences in the concentration of ARGs in wastewater entering and leaving sewer pipes. However, concentrations of blaTEM, qnrS, sul1, sul2 and IntI1 in the outlet biofilm were significantly different from the inlet biofilm concentrations (Auguet et al., Citation2017). Sul1 and sul2 were the most abundant ARGs in the biofilm with an approximate resistance gene/16S rRNA gene ratio of 0.1.
As shown here, different water and wastewater treatment systems have shown differences in the ARG removal capability; depending on the unit process and ARG of concern. In some cases, same treatment unit process has shown different removal efficiencies of ARGs depending on the treatment flow. For example, sand filtration contributed to ARG reduction in the advanced drinking water treatment process while it led to ARG propagation during the conventional process. In the advanced process, reduction of organic matter by preozonation of water before sand filtration reduced the possibility of biofilm development and ARG dissemination (Hu, Zhang, Jiang, Luo, et al., Citation2019). Since, present drinking water treatment plants, WWTPs and collection systems act as ARG reservoirs and HGT hot spots, further technological advances are necessary in order to reduce the release and propagation of ARGs in water environments.
5. Challenging questions regarding the ARB and ARGs in aquatic environments
5.1 How to quantify the health risks caused by antibiotics, ARB and ARGs present in aquatic environments?
Quantitative microbial risk assessment (QMRA) approach combines the information on occurrence, exposure and dose-response to evaluate the potential health risks posed by a particular pathogen (). It has been applied in calculating the health risks caused by E. coli, Campylobacter, Legionella and other pathogens in different scenarios (Evers, Blaak, Hamidjaja, de Jonge, & Schets, Citation2016; Hamilton & Haas, Citation2016; Strachan, Dunn, Locking, Reid, & Ogden, Citation2006). QMRA has been suggested as a suitable method to quantitatively evaluate the additional human health risk caused by the ingestion of ARB in the environment (Ashbolt et al., Citation2013; Hong et al., Citation2018; Manaia, Citation2017), and Ashbolt et al. (Citation2013) analyzed the feasibility and complexities of applying QMRA framework to evaluate the health risks caused by ARB (Ashbolt et al., Citation2013).
Figure 1. Quantitative microbial risk assessment (QMRA) procedure for assessing the human health risks by ARB and ARGs in aquatic environment. The diagram briefly describes the process and provide summarizes the current knowledge.
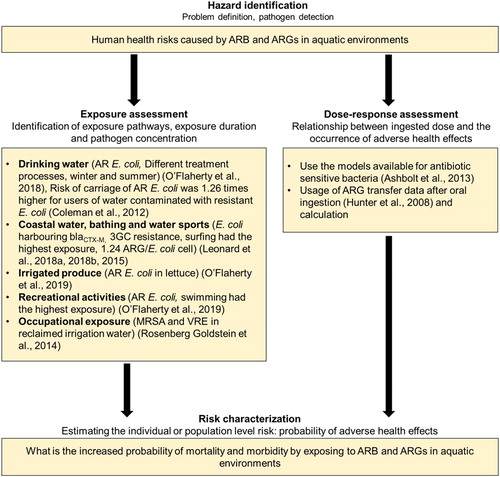
One major assumption in the approach was that pathogenic ARB will display similar behavior in the environment with nonpathogenic ARB and the pathogenicity will also be similar to antibiotic susceptible bacteria (Ashbolt et al., Citation2013; O’Flaherty, Solimini, Pantanella, De Giusti, & Cummins, Citation2019; Pepper et al., Citation2018). Based on a meta-analysis of E. coli log reduction by UV lamps (259 antibiotic sensitive E. coli data points and 44 antibiotic resistant E. coli data points), a level of 7.3 mJ/cm2 UV was required for antibiotic sensitive E. coli and 7.5 mJ/cm2 UV for antibiotic resistant E. coli with a medium- pressure lamp to achieve a 6 log reduction. For low-pressure lamps, 8.1 mJ/cm2 UV was required for antibiotic sensitive E. coli and 8.4 mJ/cm2 UV for antibiotic resistant E. coli. The study confirmed there was no statistical difference between the inactivation of antibiotic sensitive and resistant E. coli by UV and supports the idea that the behavior of ARB may be similar to antibiotic susceptible bacteria (O’Flaherty, Membré, & Cummins, Citation2018).
For the exposure assessment step of QMRA process, it is necessary to quantify the ARB or ARGs taken by an individual via ingestion, inhalation or contact during different processes like drinking or recreational activities (Manaia, Citation2017). A recent study evaluated the removal of antibiotic resistant E. coli using 5 different drinking water treatment process chain scenarios and calculated the human exposure level to antibiotic resistant E. coli by consuming drinking water treated by those systems in the summer and winter. According to the results, mean adult human exposure to antibiotic resistant E. coli from tap water consumption ranged between 3.44 × 10−7 and 2.95 × 10−1 CFU/day depending on the scenario and treatment method (O’Flaherty, Borrego, et al., Citation2018). Another study reported that the risk of carrying an antibiotic resistant E. coli is 1.26 times higher for the consumers of drinking water contaminated with resistant E. coli (Coleman et al., Citation2012). Human exposure potential to antibiotic resistant E. coli via the consumption of lettuce irrigated with surface water has been evaluated. Study design included river water from rural and urban areas and lake water from rural areas. Antibiotic resistant E. coli were categorized in to single antibiotic resistant, multiple antibiotic resistant and potentially pathogenic antibiotic resistant. Modeling process also included the influence of E. coli decay, postharvest processes, time of consumption and consumer washing on the quantity of antibiotic resistant E. coli internalized by consuming 100 g of lettuce irrigated with surface water. According to the results, antibiotic resistant E. coli quantities between 1.00 × 10−2 and 1.35 × 106 colony forming units (CFU) per 100 g of surface water irrigated lettuce can be internalized based on different scenarios. The study also revealed that postharvest treatments of washing with water, rapid cooling with water and washing with a chlorine solution can clean lettuce cultivated using irrigation water contaminated with antibiotic resistant E. coli up to 2.7, 3.1 or 4.8 log CFU/ml (O’Flaherty, Solimini, Pantanella, De Giusti, et al., Citation2019). Among the E. coli detected in coastal surface waters from England and Wales (average = 233.3 CFU/100 ml, SD = 409.5), 0.12% were third generation cephalosporins (3GCs) resistant. Quantity of 3GCs resistant E. coli ingested during water sports varied depending on the sport and the water quality of the particular location. Highest quantity of 3GCs resistant E. coli were ingested by surfers (Leonard et al., Citation2015; Leonard, Zhang, et al., Citation2018). Based on the abundance of resistance genes in bathing waters, a mean number of 1.24 resistance genes per E. coli has been estimated (Leonard, Yin, et al., Citation2018). Human exposure to antibiotic resistant E. coli by recreational activities performed in bathing sites located in close proximity to WWTPs were predicted to be between 0.45 and 345.09 CFU/100 ml (O’Flaherty, Solimini, Pantanella, & Cummins, Citation2019). ARB colonization by exposure to methicillin resistant Staphylococcus aureus and vancomycin resistant Enterococcus spp. was not detected among spray irrigation workers (Rosenberg Goldstein et al., Citation2014).
Hunter et al. (Citation2008) performed a meta-analysis and estimated that ARGs transfer rates vary from 10−2 to 10−9 related to either donor or recipient bacterial counts and calculated the probability of ARG transfer to potential pathogens following oral ingestion (Hunter, Wilkinson, Catling, & Barker, Citation2008). Based on infectious doses of different bacterial pathogens, in some cases, 10 E. coli particles are enough to infect an individual (Schmid-Hempel & Frank, Citation2007). By exposing to coastal waters in England and Wales, it is possible to ingest more than 10 3GCs resistant E. coli. There were 6.3 million water sport sessions resulting in exposure to 3GCs resistant E. coli. These data evince that the beachgoers are at risk of an ARB infection and if the exposed people have compromised immune systems, the risks will be elevated (Leonard et al., Citation2015). Moreover, colonization of ARB can occur even with a lesser number of bacteria than the infectious dose (Manaia, Citation2017). Therefore, even though information on the exact quantity of ARB necessary to colonize or infect the humans is unavailable, it will be reasonable to assume an extremely low dose of ARB can pose human health risks (Manaia, Citation2017).
Limited availability of exposure and dose-response data of ARB and ARGs for different scenarios hamper the usage of QMRA approach in quantitatively evaluating the additional human health risks posed by ARB and ARG in aquatic environments (Manaia, Citation2017; Pepper et al., Citation2018). For example, exposure to ARB via the spray irrigation of reclaimed water was found to be statistically insignificant compared to the control group, because of the smaller sample size (Rosenberg Goldstein et al., Citation2014). Further studies on exposure and dose-response assessment following the ingestion of antibiotics, ARGs and ARB will be of utmost importance since the exposure levels and HGT efficiencies can be different among different bacterial strains.
5.2. How frequently do the ARG selection and propagation occur in aquatic environments? Are there any unknown hot spots?
Transfer of resistance genes to environmental bacteria needs to overcome several bottlenecks including ecological connectivity, founder effect, fitness costs and maintenance of the resistance genes in the absence of selection pressures (Bengtsson-Palme, Kristiansson, & Larsson, Citation2018; Martínez, Citation2012; Melnyk, Wong, & Kassen, Citation2015). Therefore, presence of ARGs, ARB and MGEs in a particular aquatic environment may not provide conclusive evidence on the transfer of antibiotic resistance to environmental strains.
Acquisition of resistance plasmids or chromosomal mutations are expected to cost the fitness of bacterial cell while chromosomal mutations carry a larger fitness cost compared to the acquisition of antibiotic resistance via a plasmid as explained by the high frequency of plasmid acquired evolution of antibiotic resistance (Silva et al., Citation2011; Vogwill & Maclean, Citation2015). However, compensatory mutations are shown to arise and alleviate the negative effects of resistance mutations (Andersson & Hughes, Citation2010; Woodford & Ellington, Citation2007). Ecological connectivity bottleneck for resistance gene transfer can be eased by bacteriophages which can infect the bacteria not belonging to the same genetic exchange communities (Muniesa, Colomer-Lluch, & Jofre, Citation2013a). Bacteriophage mediated HGT is suggested to be important for transferring chromosomally located ARG from environmental bacteria to other susceptible strains when there is no selective pressure (Muniesa et al., Citation2013a).
The ability of environmental resistome to induce the antibiotic resistance in human pathogens needs to be confirmed by direct evidence. However, such studies are limited because the origin of ARGs identifiable in human pathogens may have been a taxonomically and ecologically distant bacterial population and they may have diversified within the environmental bacterial community over time (Aminov, Citation2013; Aminov & Mackie, Citation2007; Perry & Wright, Citation2013). A class-A blaCTX-M found in pathogenic bacteria was detected in environmental Kluyvera spp. whereas qnrA like genes were found in Shewanella algae (Humeniuk et al., Citation2002; Poirel, Kämpfer, & Nordmann, Citation2002; Poirel, Rodriguez-Martinez, Liard, Mammeri, & Nordmann, Citation2005). qnrA1, qnrB1, qnrS1, and aac(6’)-Ib-cr sequences isolated from marine bacteria in an aquaculture region were identical to the genes isolated from patients in neighboring hospitals with quinolone-resistant uropathogenic E. coli infections (Aedo, Ivanova, Tomova, & Cabello, Citation2014; Tomova, Ivanova, Buschmann, Godfrey, & Cabello, Citation2018; Tomova et al., Citation2015). Bacteria isolated from farmed fish contained tet genes which are 92–100% identical to the tet genes isolated from clinical bacteria suggesting the same origin (Furushita et al., Citation2003).
To confirm the selection and propagation of ARGs in aquatic environments, it is important to evaluate the transfer efficiency of ARGs by conjugation, transformation and transduction and the factors governing them. Inside a membrane bioreactor, average RP4 plasmid transfer frequency of 2.76 × 10−5/recipient was observed between E. coli K12 and indigenous bacteria. Number of transconjugants observed with Shewanella spp., Photobacterium spp., Pseudomonas spp., Proteus spp. and Vibrio spp. were higher than other genera, confirming these genra are more likely to acquire plasmid RP4 (D. Yang et al., Citation2013). Transfer rate of tet(M) gene from Gram negative marine bacteria to E. coli JM109 was 10−3, but to Enterococcus was below detection limit, whereas the tet(M) transfer rate between Gram positive marine bacteria and E. coli was below detection limit and that to Enterococcus was 10−5 (Neela, Nonaka, Rahman, & Suzuki, Citation2009). Integron mediated transfer frequency of oxytetracycline and tetracycline resistance genes between Shiga toxin-producing E. coli O157:H7 and E. coli K12 strain MG1655 CA32 in the stormwater at 28°C were 1.04 × 10−6 and 2.50 × 10−7 respectively (Nagachinta & Chen, Citation2008). Transfer frequency between the microbial community in a WWTP and two donor strains, namely E. coli MG1655 and Pseudomonas putida containing one of multidrug resistant plasmids pKJK5, pB4 or RP4 have shown to be between 3 × 10−5 and 5 × 10−4/recipient (Li et al., Citation2018). Maximum transfer efficiencies of trimethoprim resistant plasmid pKJK5 and activated sludge bacteria were in the range of 1 × 10−3–4.3 × 10−2/recipient, and transconjugants with Aeromonas, Acinetobacter and Enterobacter were dominant. Biofilm bacteria had a higher conjugative potential compared to suspended activated sludge samples (Li, Qiu, Zhang, Liang, & Huang, Citation2019). Multiple antibiotic and heavy metal resistance plasmid pSCL located in Pseudomonas fluorescens was transferred to marine bacteria in natural seawater. Conjugation efficiencies ranged from 10−4 in day one and reduced till 10−7 in day 18 when the experiment was continued (Chandrasekaran, Venkatesh, & Lalithakumari, Citation1998). Further studies on evaluating HGT frequencies in different scenarios related to aquatic environments to supplement the currently available limited knowledge will significantly contribute to the prediction of human health risks related to ARB and ARGs in water environments.
Natural transformation of environmental bacteria depends on the exposure to extracellular DNA (eDNA) (Blum, Lorenz, & Wackernagel, Citation1997; Thomas & Nielsen, Citation2005). eDNA is broadly defined as DNA that is not associated with living biomass and eDNA is assimilated in the environment due to bacterial transformation (Corinaldesi, Beolchini, & Dell’Anno, Citation2008; Mao et al., Citation2014). eDNA adsorbs to negatively charged particles like clay, quartz, feldspar and refractory organic molecules by phosphates and cation bridging (Torti, Lever, & Jørgensen, Citation2015). Reported eDNA concentrations in sediments are 3-4 orders higher than in water (Corinaldesi, Danovaro, & Dell’Anno, Citation2005; England, Vincent, Trevors, & Holmes, Citation2004; Torti et al., Citation2015). Due to the interaction with wastewater solids like clay and sand, eDNA degradation rates are lower than intracellular DNA (Torti et al., Citation2015). Microfiltration membranes commonly used for wastewater treatment are not capable of removing eDNA. However, eDNA attached to wastewater solids were removed efficiently by membrane filtration (Slipko et al., Citation2019). Report by Bien et al. (Citation2017) suggests that ARGs conveying eDNA is formed and remained by biological interaction within microbial loop (Bien et al., Citation2017). In a study performed in Haihe River basin in China, sul1, sul2, tet(W) and tet(T) concentrations in the sediment were significantly higher compared to water and these ARGs were mainly concentrated in eDNA. (Luo et al., Citation2010; Mao et al., Citation2014). By using kanamycin resistant gene located in pEASY plasmid as eDNA, Mao et al. (Citation2014) showed that eDNA has the potential to transform indigenous bacteria (Mao et al., Citation2014). Moreover, eDNA attached to sludge has shown to preferentially couple with competent cells rather than free eDNA (Dong, Wang, Fang, Wang, & Ye, Citation2019). However, studies analyzing the transformation of bacterial strains using the eDNA extracted from environmental samples are scarce. Since eDNA and more than 90 species of naturally transformable bacteria including human pathogens can be found in water environments, it is possible to expect the development of antibiotic resistance due to natural transformation in aquatic environments (Johnsborg et al., Citation2007; Mao et al., Citation2014). Future studies on quantifying the efficiency of environmental bacteria transformation by eDNA containing ARGs extracted from aquatic samples may provide valuable insights on the contribution of eDNA on the acquisition of antibiotic resistance.
Wastewater treatment plants are identified as hotspots for dissemination of antibiotic resistance (Karkman et al., Citation2018). However, it is possible to have unidentified hot spots for antibiotic resistance inside the wastewater treatment plant. One possible location is the surface of biofilms. Biofilm matrix mainly consists of extracellular polymeric substances (EPS) (Flemming, Citation2016). There are studies describing the adsorption of antibiotics to the EPS in activated sludge systems (Khunjar & Love, Citation2011; Juan Xu, Sheng, Ma, Wang, & Yu, Citation2013). It is possible that some fraction of the antibiotics gets degraded while adsorbed in the EPS. However, adsorption of antibiotics in the EPS may increase the concentration of antibiotics in the biofilm surface (. Increased concentrations of antibiotics in the biofilm surface layer may result in the selection for antibiotic resistance. However, this hypothesis has to be tested for conclusive evidence.
Figure 2. Hypothesis for new antibiotic resistance hotspots. (a) Attachment of antibiotics to EPS can increase the antibiotic concentration in the biofilm surface layer and can act as a selective pressure, (b) Elevated concentration of chemicals in the vicinity of ultrafiltration and reverse osmosis treatment systems membrane surface can act as mutagens and can lead to the development of antibiotic resistance in the bacteria in gel/cake layer and surroundings via mutation.
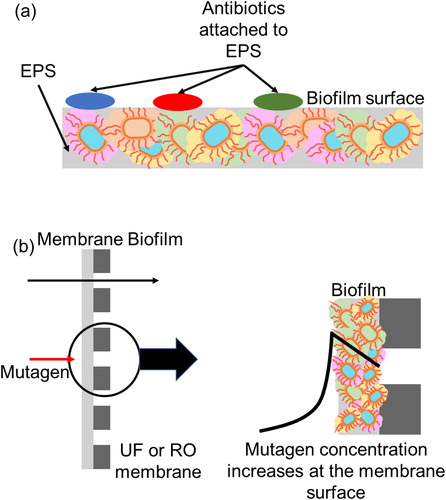
Another possible hot spot in wastewater treatment plants is the gel/cake layer in the ultrafiltration and reverse osmosis treatment systems used for tertiary treatment. Concentration of chemicals in the vicinity of the membrane surface can increase by 10 to 20 times compared to the bulk concentration due to concentration polarization. If the chemical is a mutagen, elevated concentrations of the chemical in the biofilm may act as a selective pressure to develop antibiotic resistance in the bacteria in gel/cake layer and surroundings via mutation (. Even if there is a breach of the membrane, ARB will be inactivated by disinfectants. However, if the genetic material containing ARGs which is shown to be not completely removable by disinfection are leaked to the aquatic environments, environmental bacteria can uptake the ARGs and become resistant. Therefore, in addition to monitoring membrane rupture, monitoring of ARG concentrations in reclaimed wastewater may be required in some cases.
5.3. What are the suitable markers for evaluation of ARB/ARG contamination?
Due to the large number of ARB, ARGs and antibiotics present in aquatic environments, quantification of each bacteria, gene or antibiotic concentration is time, cost and Labor intensive. A suitable ARB/ARG contamination marker can contribute to minimize the workload involved in evaluating the contamination. However, until now no consensus exists on the suitability of particular antibiotics, ARB and/or ARGs which can act as a molecular/chemical marker to evaluate the contamination of aquatic environments by antibiotics, ARB and ARGs. A recent review emphasizes there is no one-size-fits-all type of solution for this question (Huijbers, Flach, & Larsson, Citation2019). The study defines five different environmental surveillance objectives; risk of transmission, risk of evolution, risk of ecological effects, population-level resistance prevalence and population-level antibiotic use and critically discusses the suitability of markers like antibiotics, metals and biocides, known ARGs and MGEs, emerging ARGs, fecal contamination markers and ARB number to achieve the above objectives. It concludes that a combination of markers for fecal contamination and antibiotic resistance, together with selective agent measurement can be of use to achieve all five objectives (Huijbers et al., Citation2019).
By analyzing crAssphage genome sequence data obtained from wastewater samples and human samples (from 67 countries in 6 continents), Edwards et al. concluded that crAssphage is stable in individuals and common throughout the human population (Edwards et al., Citation2019). Karkman et al. (Citation2019) analyzed the correlations between crAssphage levels with ARG abundance levels in sewage (Karkman, Pärnänen, & Larsson, Citation2019). In an urban stream contaminated by human sewage, sul1, sul2, tet(O), tet(W) and ermF concentrations had significant correlations with crAssphage when quantified using digital droplet PCR (>0.8 from Spearman’s rank correlation coefficient) (Stachler, Crank, & Bibby, Citation2019). However, it has been suggested that the abundance of ARGs in sewage can be simply due to the fecal contamination instead of selection pressure (Karkman et al., Citation2019). Therefore, crAssphage quantification may only provide information about the risk of ARG transmission in aquatic environments (Huijbers et al., Citation2019).
Study specific results have been reported for the most abundant ARG in many studies conducted in different geographical locations. Sul genes were reported as the most detected ARGs in wastewater effluents from several countries including China, Sweden and USA (Ben et al., Citation2017; Berglund, Fick, & Lindgren, Citation2015; Mao et al., Citation2015; Munir et al., Citation2011). Others report tet(M) as the most commonly detected ARG in both Gram-positive and Gram-negative bacteria in wastewater lagoons, and cattle feedlots having different antibiotic usage strategies have complimented the result, suggesting tet(M) as a suitable candidate to confirm the contamination by antibiotics (Peak et al., Citation2007; Roberts, Citation2005). It is clear that the detectable ARGs and their quantities are location specific. It can be further confirmed by where different aquatic environments have shown the presence of different ARGs. However, detection of only ARGs may provide limited information regarding the risk of transmission, evolution and prevalence of resistance at population-level (Huijbers et al., Citation2019).
ARGs in aquatic environments can be transferred through mobile genetic elements (MGEs) and can later be assimilated by the environmental bacteria to become antibiotic resistant. MGEs and ARGs in aquatic environments have shown correlations (Park, Kim, Kim, & Seo, Citation2018; Pruden et al., Citation2006; Tao et al., Citation2016). IntI1 in groundwater had significant correlations (p < .01) with ermA, sul1, tet(O), and tet(W) (Szekeres et al., Citation2018). Positive correlations were observed between intI1 and qnrD, intI2 and sul3, intI3 and tet(X), Tn916/Tn1545 and sul2, and ISCR1 and sul3 in untreated hospital wastewater (Qiang Wang, Wang, & Yang, Citation2018). In a pharmaceutical WWTP, abundance of ARGs correlated with the abundance of MGEs (R2=0.52, p < .05). Among the ARGs, aminoglycoside and sulfonamide resistance genes displayed higher correlations with MGE abundance (R2 > 0.74) (Tao et al., Citation2016). In recreational water samples, IntI1 had a statistically significant positive correlation with sul1, blaTEM and tet(X) (Dong, Cui, Fang, Huang, & Wang, Citation2019). In a recent metagenomics analysis combined with proximity-ligation assay on wastewater, IntI1 exhibited links with 39 clusters within the Beta- and Gammaproteobacteria displaying the broad host range (Stalder, Press, Sullivan, Liachko, & Top, Citation2019). Therefore, detection of MGEs in aquatic environments may be considered as an indicator for the presence of ARGs. However, similar to ARGs, it may only provide limited information regarding the risk of transmission, evolution and population-level prevalence of resistance.
Antibiotics are identified as selective agents for antibiotic resistance. Based on the data from Mekong river delta and Jiulongjiang river basin, sulfamethazine (SMT) has been suggested as a molecular-marker for aquatic environment contamination by livestock sources while Haihe river basin data suggests sulfachloropyridazine (SCP) as a suitable marker for same purpose (Hongyou Jiang, Zhang, Xiao, Geng, & Zhang, Citation2013; Luo et al., Citation2011; Managaki, Murata, Takada, Bui, & Chiem, Citation2007). These results confirm that antibiotics also display the location specificity. Since apramycin is used only for swine and tylosin is exclusively used for animals (Kemper, Citation2008), detection of these antibiotics in a particular aquatic environment can lead to the conclusion of livestock related contamination. However, antibiotics like oxytetracycline is used on both animals and humans which makes it difficult to identify the contamination source if detected in municipal wastewater (Kemper, Citation2008; Shimizu et al., Citation2013). If it is possible to identify and regulate the antibiotics usable in particular situations (e.g. Swine farms only, aquaculture only, human consumption only etc.), there is a high possibility of identifying a molecular/chemical marker to identify antibiotic related contamination. These information can be of help in evaluating the risks of ecological effects and the evolution of antibiotic resistance (Huijbers et al., Citation2019).
Berendonk et al. (Citation2015) suggested an array of 6 bacterial groups (E. coli, Klebsiella pneumoniae, Aeromonas spp., P. aeruginosa, Enterococcus faecalis and Enterococcus faecium) and 13 ARGs (intI1, sul1, sul2, blaCTX-M, blaNDM-1, blaVIM, blaKPC, qnrS, aac-(6’)-lb-cr, vanA, mecA, ermB, ermF, tet(M) and aph) which can be used to assess the antibiotic resistance status in environmental settings (Berendonk et al., Citation2015). WHO suggests an integrated surveillance system under One health approach to monitor extended spectrum ESBL-E.coli in humans, food chain and the environment which can be performed in many countries with low or minimal resources (Tricycle project) and expects that the data will be comparable, statistically valid and robust (World Health Organization, Citation2017a, Citation2018). In a recent study, in vivo proximity ligation method Hi-C (physically linking ARGs, plasmids and integrons to their respective host chromosomes) was used to evaluate the plasmid-host association in a wastewater sample. According to the results, IncQ plasmids and class I integrons had the broadest host range. Among all the detected bacterial taxa, Aeromonadaceae was associated with the highest number of ARGs (Stalder et al., Citation2019). Therefore, it may be possible to use the bacteria from Aeromonadaceae as potential markers for the contamination of aquatic environments with ARB and ARGs.
Types and concentrations of antibiotics used in different countries and geographical regions vary and as a consequence, detectable antibiotics, ARB and ARGs in aquatic environments will also be location specific with varying compositions (Shimizu et al., Citation2013). A systematic review on the abundant ARB in different water environments can be of great help in narrowing down the candidate markers for antibiotic contamination. Proximity-ligation can be optimized using methods like abundance normalization to obtain the connections between the plasmids carrying ARGs and their hosts (Stalder et al., Citation2019). These narrow-down methods combined with qPCR-based quantification may provide valuable information regarding the ARB which can be used as contamination markers. In terms of evaluating the human health risks, the absolute number of ARB present in a particular aquatic sample is essential. Therefore, the usage of ARB contamination markers will significantly contribute to the evaluation of human health risks caused by antibiotic resistance in aquatic environments.
5.4. What is the effect of VBNC status on antibiotic resistance?
Majority of the bacterial strains including human enteric bacteria can’t be cultured, are obligate anaerobes or viable but non-culturable (VBNC) (Amann, Ludwig, & Schleifer, Citation1995; Miura et al., Citation2013). Up to now, most of the evaluations on ARB has been conducted with culturable fraction of bacteria. However, several studies have attempted to answer the contribution of VBNC state bacteria on the antibiotic resistance. Vancomycin-resistant enterococci maintained the antibiotic resistance during the VBNC state and the resuscitated cells also were resistant to vancomycin (Lleo, Bonato, Signoretto, & Canepari, 2003). According to Lin et al. (Citation2017), low dosage of free chlorine disinfection (0.5 mg/L) effectively reduced the cultivability of E. coli and induced a VBNC state. VBNC bacteria displayed higher persistence to 9 antibiotics compared to their culturable counterparts. Transcript expression levels of folA, acrA and acrB (multidrug efflux) genes were increased more than 2-fold in the VBNC E. coli compared to culturable bacteria (Lin, Ye, Chen, Zhang, & Yu, Citation2017). Even though E. coli became VBNC during the low nutrient and energy phase of carcass leachate treatment, other bacteria present in the leachate took up the plasmid indicating the nutrient deprivation and VBNC bacteria may not hinder the plasmid transfer (Salcedo & Kim, Citation2017). Plasmid transfer efficiency between VBNC P. fluorescens and marine bacteria was 10−5 at the initiation of VBNC state and decreased with the number of days passing. After 15 days from the beginning of VBNC state, transfer efficiency was stabilized at 10−7 range (Chandrasekaran et al., Citation1998). sul3 was detected only in the non-culturable fraction of bacteria in the water collected from Japanese marine aquaculture environments, Manila Bay and Taiwanese aquaculture water (Suzuki et al., Citation2013, Citation2019).
Since disinfection can induce VBNC state in bacteria, VBNC bacteria can be antibiotic resistant, and the VBNC bacteria carrying ARGs can survive and resuscitate after the removal of stress conditions, it is important to include the VBNC fraction of bacteria in evaluating the human health risks caused by ARB and ARGs. However, it is not always possible to separately quantify the culturable and VBNC bacterial fraction in each sample. To address this problem, several studies have evaluated and modeled the induction of VBNC state in culturable bacteria under disinfectants like chlorine, chloramine (S. Chen et al., Citation2019; Oliver, Dagher, & Linden, Citation2005) and UV (Shenghua Zhang, Ye, Lin, Lv, & Yu, Citation2015). As the majority of environmental bacteria belong to VBNC, these models combined with the data on culturable ARB quantities may be usable in approximating the VBNC fraction of ARBs in a sample and in turn in evaluating the health risks.
6. Conclusions
Ingestion of antibiotic resistant bacteria and antibiotic resistance genes present in aquatic environments can pose a human health risk. However, currently it is not possible to quantitatively evaluate this human health using QMRA, because of the limited availability of necessary information including exposure assessment and dose-response assessment data for different scenarios. Furthermore, there are unknown hotspots contributing to the propagation of antibiotic resistance in aquatic environments. Role of eDNA which is ubiquitous in water environments on the acquisition of antibiotic resistance by environmental bacteria through natural transformation needs to be further elucidated. Identification of suitable antibiotic resistance markers also is a challenge because there is no one-size-fits-all solution. However, identifying an ARB as a contamination marker will be beneficial in terms of human health risk evaluation. Contribution of the VBNC fraction of bacteria on the propagation of antibiotic resistance and their effects on the human health also is a point of concern.
Disclosure statement
No potential conflict of interest
Additional information
Funding
References
- Aedo, S., Ivanova, L., Tomova, A., & Cabello, F. C. (2014). Plasmid-related quinolone resistance determinants in epidemic Vibrio parahaemolyticus, uropathogenic Escherichia coli, and marine bacteria from an aquaculture area in Chile. Microbial Ecology, 68(2), 324–328. doi:10.1007/s00248-014-0409-2
- Ahmed, A. M., Maruyama, A., Khalifa, H. O., Shimamoto, T., & Shimamoto, T. (2015). Seafood as a reservoir of Gram-negative bacteria carrying integrons and antimicrobial resistance genes in Japan. Biomedical and Environmental Sciences, 28(12), 924–926. doi: 10.3967/bes2015.128
- Amann, R. I., Ludwig, W., & Schleifer, K. H. (1995). Phylogenetic identification and in situ detection of individual microbial cells without cultivation. Microbiological Reviews, 59(1), 143–169. doi: 10.1016/j.jip.2007.09.009
- Amarasiri, M., Kitajima, M., Nguyen, T. H., Okabe, S., & Sano, D. (2017). Bacteriophage removal efficiency as a validation and operational monitoring tool for virus reduction in wastewater reclamation: Review. Water Research, 121, 258–269. doi:10.1016/j.watres.2017.05.035
- Aminov, R. I. (2011). Horizontal gene exchange in environmental microbiota. Frontiers in Microbiology, 2, 158. doi:10.3389/fmicb.2011.00158
- Aminov, R. I. (2013). Evolution in action: Dissemination of tet(X) into pathogenic microbiota. Frontiers in Microbiology, 4, 192. doi:10.3389/fmicb.2013.00192
- Aminov, R. I., & Mackie, R. I. (2007). Evolution and ecology of antibiotic resistance genes. FEMS Microbiology Letters, 271(2), 147–161. doi:10.1111/j.1574-6968.2007.00757.x
- Anderson, M. A., Whitlock, J. E., & Harwood, V. J. (2006). Diversity and distribution of Escherichia coli genotypes and antibiotic resistance phenotypes in feces of humans, cattle, and horses. Applied and Environmental Microbiology, 72(11), 6914–6922. doi:10.1128/AEM.01029-06
- Andersson, D. I., & Hughes, D. (2010). Antibiotic resistance and its cost: Is it possible to reverse resistance? Nature Reviews Microbiology, 8(4), 260–271. doi:10.1038/nrmicro2319
- Ashbolt, N. J., Amézquita, A., Backhaus, T., Borriello, P., Brandt, K. K., Collignon, P., … Topp, E. (2013). Human health risk assessment (HHRA) for environmental development and transfer of antibiotic resistance. Environmental Health Perspectives, 121(9), 993–1001. doi:10.1289/ehp.1206316
- Auguet, O., Pijuan, M., Borrego, C. M., Rodriguez-Mozaz, S., Triadó-Margarit, X., Giustina, S. V. D., & … Utierrez, O. (2017). Sewers as potential reservoirs of antibiotic resistance. Science of the Total Environment, 605–606, 1047–1054. doi:10.1016/j.scitotenv.2017.06.153
- Azanu, D., Styrishave, B., Darko, G., Weisser, J. J., & Abaidoo, R. C. (2018). Occurrence and risk assessment of antibiotics in water and lettuce in Ghana. Science of the Total Environment, 622–623, 293–305. doi:10.1016/j.scitotenv.2017.11.287
- Baam, R. B., Gandhi, N. M., & Freitas, Y. M. (1966). Antibiotic activity of marine microorganisms: The antibacterial spectrum. Helgoländer Wissenschaftliche Meeresuntersuchungen, 13(1–2), 188–191. doi:10.1007/BF01612664
- Ben, W., Wang, J., Cao, R., Yang, M., Zhang, Y., & Qiang, Z. (2017). Distribution of antibiotic resistance in the effluents of ten municipal wastewater treatment plants in China and the effect of treatment processes. Chemosphere, 172, 392–398. doi:10.1016/j.chemosphere.2017.01.041
- Bengtsson-Palme, J., Kristiansson, E., & Larsson, D. G. J. (2018). Environmental factors influencing the development and spread of antibiotic resistance. FEMS Microbiology Reviews, 42(1), 68–80. doi:10.1093/femsre/fux053
- Berendonk, T. U., Manaia, C. M., Merlin, C., Fatta-Kassinos, D., Cytryn, E., Walsh, F., … Martinez, J. L. (2015). Tackling antibiotic resistance: The environmental framework. Nature Reviews Microbiology, 13(5), 310–317. doi:10.1038/nrmicro3439
- Berglund, B., Fick, J., & Lindgren, P.-E. (2015). Urban wastewater effluent increases antibiotic resistance gene concentrations in a receiving northern European river. Environmental Toxicology and Chemistry, 34(1), 192–196. doi:10.1002/etc.2784
- Bien, T. L. T., Thao, N. V., Kitamura, S.-I., Obayashi, Y., & Suzuki, S. (2017). Release and constancy of an antibiotic resistance gene in seawater under grazing stress by ciliates and heterotrophic nanoflagellates. Microbes and Environments, 32(2), 174–179. doi:10.1264/jsme2.ME17042
- Blum, S. A. E., Lorenz, M. G., & Wackernagel, W. (1997). Mechanism of retarded DNA degradation and prokaryotic origin of DNases in nonsterile soils. Systematic and Applied Microbiology, 20(4), 513–521. doi:10.1016/S0723-2020(97)80021-5
- Calero-Cáceres, W., & Balcázar, J. L. (2019). Antibiotic resistance genes in bacteriophages from diverse marine habitats. Science of the Total Environment, 654, 452–455. doi:10.1016/j.scitotenv.2018.11.166
- Calero-Cáceres, W., Ye, M., & Balcázar, J. L. (2019). Bacteriophages as environmental reservoirs of antibiotic resistance. Trends in Microbiology, 27(7), 570–577. doi:10.1016/j.tim.2019.02.008
- Calomiris, J. J., Armstrong, J. L., & Seidler, R. J. (1984). Association of metal tolerance with multiple antibiotic resistance of bacteria isolated from drinking water. Applied and Environmental Microbiology, 47(6), 1238–1242.
- Chamier, B., Lorenz, M. G., & Wackernagel, W. (1993). Natural transformation of Acinetobacter calcoaceticus by plasmid DNA adsorbed on sand and groundwater aquifer material. Applied and Environmental Microbiology, 59(5), 1662–1667.
- Chandrasekaran, S., Venkatesh, B., & Lalithakumari, D. (1998). Transfer and expression of a multiple antibiotic resistance plasmid in marine bacteria. Current Microbiology, 37(5), 347–351. doi:10.1007/s002849900390
- Chen, B., Yang, Y., Liang, X., Yu, K., Zhang, T., & Li, X. (2013). Metagenomic profiles of antibiotic resistance genes (ARGs) between human impacted estuary and deep ocean sediments. Environmental Science & Technology, 47(22), 12753–12760. doi:10.1021/es403818e
- Chen, C. Q., Zheng, L., Zhou, J. L., & Zhao, H. (2017). Persistence and risk of antibiotic residues and antibiotic resistance genes in major mariculture sites in Southeast China. Science of the Total Environment, 580, 1175–1184. doi:10.1016/j.scitotenv.2016.12.075
- Chen, Q.-L., Li, H., Zhou, X.-Y., Zhao, Y., Su, J.-Q., Zhang, X., & Huang, F.-Y. (2017). An underappreciated hotspot of antibiotic resistance: The groundwater near the municipal solid waste landfill. Science of the Total Environment, 609, 966–973. doi:10.1016/j.scitotenv.2017.07.164
- Chen, S., Zeng, J., Wang, Y., Ye, C., Zhu, S., Feng, L., … Yu, X. (2019). Modelling the effect of chlorination/chloramination on induction of viable but non-culturable (VBNC) Escherichia coli. Environmental Technology, 1–13. doi:10.1080/09593330.2019.1611939
- Chow, L., Waldron, L., & Gillings, M. R. (2015). Potential impacts of aquatic pollutants: Sub-clinical antibiotic concentrations induce genome changes and promote antibiotic resistance. Frontiers in Microbiology, 6(AUG), 1–10. doi:10.3389/fmicb.2015.00803
- Chu, B. T. T., Petrovich, M. L., Chaudhary, A., Wright, D., Murphy, B., Wells, G., & Poretsky, R. (2018). Metagenomics reveals the impact of wastewater treatment plants on the dispersal of microorganisms and genes in aqautic sediments. Applied and Environmental Microbiology, 84(5), e02168–17. doi:10.1128/AEM.02168-17
- Chung, S. S., Zheng, J. S., Burket, S. R., & Brooks, B. W. (2018). Select antibiotics in leachate from closed and active landfills exceed thresholds for antibiotic resistance development. Environment International, 115(January), 89–96. doi:10.1016/j.envint.2018.03.014
- Coleman, B. L., Salvadori, M. I., McGEER, A. J., Sibley, K. A., Neumann, N. F., Bondy, S. J., … Louie, M. (2012). The role of drinking water in the transmission of antimicrobial-resistant E. coli. Epidemiology and Infection, 140(4), 633–642. doi:10.1017/S0950268811001038
- Corinaldesi, C., Beolchini, F., & Dell’Anno, A. (2008). Damage and degradation rates of extracellular DNA in marine sediments: Implications for the preservation of gene sequences. Molecular Ecology, 17(17), 3939–3951. doi:10.1111/j.1365-294X.2008.03880.x
- Corinaldesi, C., Danovaro, R., & Dell'Anno, A. (2005). Simultaneous recovery of extracellular and intracellular DNA suitable for molecular studies from marine sediments. Applied and Environmental Microbiology, 71(1), 46–50. doi: 10.1128/AEM.71.1.46
- Cosgrove, S. E. (2006). The relationship between antimicrobial resistance and patient outcomes: Mortality, length of hospital stay, and health care costs. Clinical Infectious Diseases, 42(Supplement_2), S82–S89. doi:10.1086/499406
- Davies, J., & Davies, D. (2010). Origins and evolution of antibiotic resistance. Microbiology and Molecular Biology Reviews, 74(3), 417–433. doi:10.1128/MMBR.00016-10
- Davison, J. (1999). Genetic exchange between bacteria in the environment. Plasmid, 91, 73–91. doi:10.1006/plas.1999.1421
- de Vries, J., & Wackernagel, W. (2005). Microbial horizontal gene transfer and the DNA release from transgenic crop plants. Plant and Soil, 266(1-2), 91–104. doi:10.1007/s11104-005-4783-x
- Dong, P., Cui, Q., Fang, T., Huang, Y., & Wang, H. (2019). Occurrence of antibiotic resistance genes and bacterial pathogens in water and sediment in urban recreational water. Journal of Environmental Sciences (Sciences), 77, 65–74. doi:10.1016/j.jes.2018.06.011
- Dong, P., Wang, H., Fang, T., Wang, Y., & Ye, Q. (2019). Assessment of extracellular antibiotic resistance genes (eARGs) in typical environmental samples and the transforming ability of eARG. Environment International, 125(December 2018), 90–96. doi:10.1016/j.envint.2019.01.050
- Edwards, R. A., Vega, A. A., Norman, H. M., Ohaeri, M., Levi, K., Dinsdale, E. A., … Dutilh, B. E. (2019). Global phylogeography and ancient evolution of the widespread human gut virus crAssphage. Nature Microbiology, 4(10), 1727. doi:10.1038/s41564-019-0494-6
- England, L. S., Vincent, M. L., Trevors, J. T., & Holmes, S. B. (2004). Extraction, detection and persistence of extracellular DNA in forest litter microcosms. Molecular and Cellular Probes, 18(5), 313–319. doi:10.1016/j.mcp.2004.05.001
- Evers, E. G., Blaak, H., Hamidjaja, R. A., de Jonge, R., & Schets, F. M. (2016). A QMRA for the transmission of ESBL-producing Escherichia coli and Campylobacter from poultry farms to humans through flies. Risk Analysis, 36(2), 215–227. doi:10.1111/risa.12433
- Fahrenfeld, N., Ma, Y., O’Brien, M., & Pruden, A. (2013). Reclaimed water as a reservoir of antibiotic resistance genes: Distribution system and irrigation implications. Frontiers in Microbiology, 4, 130. doi:10.3389/fmicb.2013.00130
- Fajardo, A., Martínez-Martín, N., Mercadillo, M., Galán, J. C., Ghysels, B., Matthijs, S., … Martínez, J. L. (2008). The neglected intrinsic resistome of bacterial pathogens. PLoS One, 3(2), e1619–6. doi:10.1371/journal.pone.0001619
- Fang, T., Wang, H., Cui, Q., Rogers, M., & Dong, P. (2018). Diversity of potential antibiotic-resistant bacterial pathogens and the effect of suspended particles on the spread of antibiotic resistance in urban recreational water. Water Research, 145, 541–551. doi:10.1016/j.watres.2018.08.042
- Fekadu, S., Alemayehu, E., Dewil, R., & Van der Bruggen, B. (2019). Pharmaceuticals in freshwater aquatic environments: A comparison of the African and European challenge. Science of the Total Environment, 654, 324–337. doi:10.1016/j.scitotenv.2018.11.072
- Flemming, H.-C. (2016). EPS—Then and now. Microorganisms, 4(4), 41. doi:10.3390/microorganisms4040041
- Ford, T. (2006). Emerging issues in water and health research. Journal of Water and Health, 4(S1), 59–66. doi:10.2166/wh.2006.0044
- Furushita, M., Shiba, T., Maeda, T., Yahata, M., Kaneoka, A., Takahashi, Y., … Ohta, M. (2003). Similarity of tetracycline resistance genes isolated from fish farm bacteria to those from clinical isolates. Applied and Environmental Microbiology, 69(9), 5336–5342. doi:10.1128/AEM.69.9.5336-5342.2003
- Gao, Q., Li, Y., Qi, Z., Yue, Y., Min, M., Peng, S., … Gao, Y. (2018). Diverse and abundant antibiotic resistance genes from mariculture sites of China’s coastline. Science of the Total Environment, 630, 117–125. doi:10.1016/j.scitotenv.2018.02.122
- Garner, E., Chen, C., Xia, K., Bowers, J., Engelthaler, D. M., McLain, J., … Pruden, A. (2018). Metagenomic characterization of antibiotic resistance genes in full-scale reclaimed water distribution systems and corresponding potable systems. Environmental Science & Technology, 52(11), 6113–6125. doi:10.1021/acs.est.7b05419
- Griffiths, R. P., Moyer, C. L., Caldwell, B. A., Ye, C., & Morita, R. Y. (1990). Long-term starvation-induced loss of antibiotic resistance in bacteria. Microbial Ecology, 19(3), 251–257. doi:10.1007/BF02017169
- Gullberg, E., Cao, S., Berg, O. G., Ilbäck, C., Sandegren, L., Hughes, D., & Andersson, D. I. (2011). Selection of resistant bacteria at very low antibiotic concentrations. PLoS Pathogens, 7(7), e1002158. doi:10.1371/journal.ppat.1002158
- Guo, X., Yan, Z., Zhang, Y., Xu, W., Kong, D., Shan, Z., & Wang, N. (2018). Behavior of antibiotic resistance genes under extremely high-level antibiotic selection pressures in pharmaceutical wastewater treatment plants. Science of the Total Environment, 612, 119–128. doi:10.1016/j.scitotenv.2017.08.229
- Haller, L., Chen, H., Ng, C., Le, T. H., Koh, T. H., Barkham, T., … Gin, K. Y.-H. (2018). Occurrence and characteristics of extended-spectrum β-lactamase- and carbapenemase- producing bacteria from hospital effluents in Singapore. Science of the Total Environment, 615, 1119. doi:10.1016/j.scitotenv.2017.09.217
- Hamilton, K. A., & Haas, C. N. (2016). Critical review of mathematical approaches for quantitative microbial risk assessment (QMRA) of Legionella in engineered water systems: Research gaps and a new framework. Environmental Science: Water Research & Technology, 2(4), 599–613. doi:10.1039/C6EW00023A
- Hao, H., Shi, D-y., Yang, D., Yang, Z-W., Qiu, Z-G., Liu, W-L., … Jin, M. (2019). Profiling of intracellular and extracellular antibiotic resistance genes in tap water. Journal of Hazardous Materials, 365(July 2018), 340–345. doi:10.1016/j.jhazmat.2018.11.004
- Hatosy, S. M., & Martiny, A. C. (2015). The ocean as a global reservoir of antibiotic resistance genes. Applied and Environmental Microbiology, 81(21), 7593–7599. doi:10.1128/AEM.00736-15
- Hendriksen, R. S., Munk, P., Njage, P., van Bunnik, B., McNally, L., Lukjancenko, O., … Aarestrup, F. M. (2019). Global monitoring of antimicrobial resistance based on metagenomics analyses of urban sewage. Nature Communications, 10(1), 1124. doi:10.1038/s41467-019-08853-3
- Hong, P.-Y., Julian, T., Pype, M.-L., Jiang, S., Nelson, K., Graham, D., … Manaia, C. (2018). Reusing treated wastewater: Consideration of the safety aspects associated with antibiotic-resistant bacteria and antibiotic resistance genes. Water, 10(3), 244. doi:10.3390/w10030244
- Hu, Y., Zhang, T., Jiang, L., Luo, Y., Yao, S., Zhang, D., … Cui, C. (2019). Occurrence and reduction of antibiotic resistance genes in conventional and advanced drinking water treatment processes. Science of the Total Environment, 669(130), 777–784. doi:10.1016/j.scitotenv.2019.03.143
- Hu, Y., Zhang, T., Jiang, L., Yao, S., Ye, H., Lin, K., & Cui, C. (2019). Removal of sulfonamide antibiotic resistant bacterial and intracellular antibiotic resistance genes by UVC-activated peroxymonosulfate. Chemical Engineering Journal, 368(November 2018), 888–895. doi:10.1016/j.cej.2019.02.207
- Huang, H., Zeng, S., Dong, X., Li, D., Zhang, Y., He, M., & Du, P. (2019). Diverse and abundant antibiotics and antibiotic resistance genes in an urban water system. Journal of Environmental Management, 231, 494–503. doi:10.1016/j.jenvman.2018.10.051
- Huijbers, P. M. C., Flach, C.-F., & Larsson, D. G. J. (2019). A conceptual framework for the environmental surveillance of antibiotics and antibiotic resistance. Environment International, 130(May), 104880. doi:10.1016/j.envint.2019.05.074
- Humeniuk, C., Arlet, G., Gautier, V., Grimont, P., Labia, R., & Philippon, A. (2002). β-lactamases of Kluyvera ascorbata, probable progenitors of some plasmid-encoded CTX-M types. Antimicrobial Agents and Chemotherapy, 46(9), 3045–3049. doi:10.1128/AAC.46.9.3045-3049.2002
- Hunter, P. R., Wilkinson, D. C., Catling, L. A., & Barker, G. C. (2008). Meta-analysis of experimental data concerning antimicrobial resistance gene transfer rates during conjugation. Applied and Environmental Microbiology, 74(19), 6085–6090. doi:10.1128/AEM.01036-08
- Interagency Coordination Group on Antimicrobial Resistance. (2019). No time to wait: Securing the future from drug-resistant infections Report To the Secretary-General of the United Nations.
- Ishikawa, N. K., Touno, E., Higashiyama, Y., Sasamoto, M., Soma, M., Yoshida, N., … Umita, T. (2018). Determination of tylosin excretion from sheep to assess tylosin spread to agricultural fields by manure application. Science of the Total Environment, 633, 399–404. doi:10.1016/j.scitotenv.2018.03.216
- Jiang, H., Zhou, R., Yang, Y., Chen, B., Cheng, Z., Zhang, M., … Zou, S. (2018). Characterizing the antibiotic resistance genes in a river catchment: Influence of anthropogenic activities. Journal of Environmental Sciences (Sciences), 69, 125–132. doi:10.1016/j.jes.2017.08.009
- Jiang, H., Zhou, R., Zhang, M., Cheng, Z., Li, J., Zhang, G., … Yang, Y. (2018). Exploring the differences of antibiotic resistance genes profiles between river surface water and sediments using metagenomic approach. Ecotoxicology and Environmental Safety, 161(February), 64–69. doi:10.1016/j.ecoenv.2018.05.044
- Jiang, H., Zhang, D., Xiao, S., Geng, C., & Zhang, X. (2013). Occurrence and sources of antibiotics and their metabolites in river water, WWTPs, and swine wastewater in Jiulongjiang River basin, south China. Environmental Science and Pollution Research, 20(12), 9075–9083. doi:10.1007/s11356-013-1924-2
- Jiao, Y. N., Chen, H., Gao, R. X., Zhu, Y. G., & Rensing, C. (2017). Organic compounds stimulate horizontal transfer of antibiotic resistance genes in mixed wastewater treatment systems. Chemosphere, 184, 53–61. doi:10.1016/j.chemosphere.2017.05.149
- Jin, M., Lu, J., Chen, Z., Nguyen, S. H., Mao, L., Li, J., … Guo, J. (2018). Antidepressant fluoxetine induces multiple antibiotics resistance in Escherichia coli via ROS-mediated mutagenesis. Environment International, 120(July), 421–430. doi:10.1016/j.envint.2018.07.046
- Johnsborg, O., Eldholm, V., & Håvarstein, L. S. (2007). Natural genetic transformation: Prevalence, mechanisms and function. Research in Microbiology, 158(10), 767–778. doi:10.1016/j.resmic.2007.09.004
- Jutkina, J., Marathe, N. P., Flach, C.-F., & Larsson, D. G. J. (2018). Antibiotics and common antibacterial biocides stimulate horizontal transfer of resistance at low concentrations. Science of the Total Environment, 616–617, 172–178. doi:10.1016/j.scitotenv.2017.10.312
- Kampf, G. (2018). Biocidal agents used for disinfection can enhance antibiotic resistance in Gram-negative species. Antibiotics, 7(4), 110. doi:10.3390/antibiotics7040110
- Kampf, G. (2019). Antibiotic resistance can be enhanced in Gram-positive species by some biocidal agents used for disinfection. Antibiotics, 8(1), 13. doi:10.3390/antibiotics8010013
- Karkman, A., Do, T. T., Walsh, F., & Virta, M. P. J. (2018). Antibiotic-resistance genes in wastewater. Trends in Microbiology, 26(3), 220–228. doi:10.1016/j.tim.2017.09.005
- Karkman, A., Pärnänen, K., & Larsson, D. G. J. (2019). Fecal pollution can explain antibiotic resistance gene abundances in anthropogenically impacted environments. Nature Communications, 10(1), 80. doi:10.1038/s41467-018-07992-3
- Kemper, N. (2008). Veterinary antibiotics in the aquatic and terrestrial environment. Ecological Indicators, 8(1), 1–13. doi:10.1016/j.ecolind.2007.06.002
- Khunjar, W. O., & Love, N. G. (2011). Sorption of carbamazepine, 17α-ethinylestradiol, iopromide and trimethoprim to biomass involves interactions with exocellular polymeric substances. Chemosphere, 82(6), 917–922. doi:10.1016/j.chemosphere.2010.10.046
- Kim, S., Yun, Z., Ha, U. H., Lee, S., Park, H., Kwon, E. E., … Chandran, K. (2014). Transfer of antibiotic resistance plasmids in pure and activated sludge cultures in the presence of environmentally representative micro-contaminant concentrations. Science of the Total Environment, 468–469, 813–820. doi:10.1016/j.scitotenv.2013.08.100
- Knapp, C. W., Engemann, C. A., Hanson, M. L., Keen, P. L., Hall, K. J., & Graham, D. W. (2008). Indirect evidence of transposon-mediated selection of antibiotic resistance genes in aquatic systems at low level oxytetracycline exposures. Environmental Science & Technology, 42(14), 5348–5353. doi:10.1021/es703199g
- Kohanski, M. A., DePristo, M. A., & Collins, J. J. (2010). Sublethal antibiotic treatment leads to multidrug resistance via radical-induced mutagenesis. Molecular Cell, 37(3), 311–320. doi:10.1016/j.molcel.2010.01.003
- Langford, F. M., Weary, D. M., & Fisher, L. (2003). Antibiotic resistance in gut bacteria from dairy calves: A dose response to the level of antibiotics fed in milk. Journal of Dairy Science, 86(12), 3963–3966. doi:10.3168/jds.S0022-0302(03)74006-5
- Le, T.-H., Ng, C., Ngoc, H. T., Chen, H., & Gin, K. Y.-H. (2018). Removal of antibiotic residues, antibiotic resistant bacteria and antibiotic resistance genes in municipal wastewater by membrane bioreactor systems. Water Research, 145, 498–508. doi:10.1016/j.watres.2018.08.060
- Leonard, A., Yin, X. L., Zhang, T., Hui, M., & Gaze, W. H. (2018). A coliform-targeted metagenomic method facilitating human exposure estimates to Escherichia coli-borne antibiotic resistance genes. FEMS Microbiology Ecology, 94(3), fiy024. doi:10.1093/femsec/fiy024
- Leonard, A., Zhang, L., Balfour, A. J., Garside, R., & Gaze, W. H. (2015). Human recreational exposure to antibiotic resistant bacteria in coastal bathing waters. Environment International, 82, 92–100. doi:10.1016/j.envint.2015.02.013
- Leonard, A. F. C., Zhang, L., Balfour, A. J., Garside, R., Hawkey, P. M., Murray, A. K., … Gaze, W. H. (2018). Exposure to and colonisation by antibiotic-resistant E. coli in UK coastal water users: Environmental surveillance, exposure assessment, and epidemiological study (Beach Bum Survey). Environment International, 114, 326–333. doi:10.1016/j.envint.2017.11.003
- Li, B., Qiu, Y., Zhang, J., Liang, P., & Huang, X. (2019). Conjugative potential of antibiotic resistance plasmids to activated sludge bacteria from wastewater treatment plants. International Biodeterioration & Biodegradation, 138(September 2018), 33–40. doi:10.1016/j.ibiod.2018.12.013
- Li, D., Zeng, S., He, M., & Gu, A. Z. (2016). Water disinfection byproducts induce antibiotic resistance-role of environmental pollutants in resistance phenomena. Environmental Science & Technology, 50(6), 3193–3201. doi:10.1021/acs.est.5b05113
- Li, J., Cheng, W., Xu, L., Strong, P. J., & Chen, H. (2015). Antibiotic-resistant genes and antibiotic-resistant bacteria in the effluent of urban residential areas, hospitals, and a municipal wastewater treatment plant system. Environmental Science and Pollution Research, 22(6), 4587–4596. doi:10.1007/s11356-014-3665-2
- Li, L., Dechesne, A., He, Z., Madsen, J. S., Nesme, J., Sørensen, S. J., & Smets, B. F. (2018). Estimating the transfer range of plasmids encoding antimicrobial resistance in a wastewater treatment plant microbial community. Environmental Science & Technology Letters, 5(5), 260–265. doi:10.1021/acs.estlett.8b00105
- Li, X., Gu, A. Z., Zhang, Y., Xie, B., Li, D., & Chen, J. (2019). Sub-lethal concentrations of heavy metals induce antibiotic resistance via mutagenesis. Journal of Hazardous Materials, 369(September 2018), 9–16. doi:10.1016/j.jhazmat.2019.02.006
- Lin, H., Ye, C., Chen, S., Zhang, S., & Yu, X. (2017). Viable but non-culturable E. coli induced by low level chlorination have higher persistence to antibiotics than their culturable counterparts. Environmental Pollution, 230, 242–249. doi:10.1016/j.envpol.2017.06.047
- Lin, W., Li, S., Zhang, S., & Yu, X. (2016). Reduction in horizontal transfer of conjugative plasmid by UV irradiation and low-level chlorination. Water Research, 91, 331–338. doi:10.1016/j.watres.2016.01.020
- Lin, W., Zhang, M., Zhang, S., & Yu, X. (2016). Can chlorination co-select antibiotic-resistance genes? Chemosphere, 156, 412–419. doi:10.1016/j.chemosphere.2016.04.139
- Liu, A., Fong, A., Becket, E., Yuan, J., Tamae, C., Medrano, L., … Miller, J. H. (2011). Selective advantage of resistant strains at trace levels of antibiotics: A simple and ultrasensitive color test for detection of antibiotics and genotoxic agents. Antimicrobial Agents and Chemotherapy, 55(3), 1204–1210. doi:10.1128/AAC.01182-10
- Lleo, M. de M., Bonato, B., Signoretto, C., & Canepari, P. (2003). Vancomycin resistance is maintained in Enterococci in the viable but nonculturable state and after division is resumed. Antimicrobial Agents and Chemotherapy, 47(3), 1154–1156. doi:10.1128/AAC.47.3.1154-1156.2003
- Lorenz, M. G., & Wackernagel, W. (1994). Bacterial gene transfer by natural genetic transformation in the environment. Microbiological Reviews, 58(3), 563–602. Retrieved from
- Lu, J., Jin, M., Nguyen, S. H., Mao, L., Li, J., Coin, L. J. M., … Guo, J. (2018). Non-antibiotic antimicrobial triclosan induces multiple antibiotic resistance through genetic mutation. Environment International, 118(February), 257–265. doi:10.1016/j.envint.2018.06.004
- Lu, J., Wang, Y., Li, J., Mao, L., Nguyen, S. H., Duarte, T., … Guo, J. (2018). Triclosan at environmentally relevant concentrations promotes horizontal transfer of multidrug resistance genes within and across bacterial genera. Environment International, 121(October), 1217–1226. doi:10.1016/j.envint.2018.10.040
- Luo, Y., Mao, D., Rysz, M., Zhou, Q., Zhang, H., Xu, L., & Alvarez, P. J. J. (2010). Trends in antibiotic resistance genes occurrence in the Haihe River, China. Environmental Science & Technology, 44(19), 7220–7225. doi:10.1021/es100233w
- Luo, Y., Xu, L., Rysz, M., Wang, Y., Zhang, H., & Alvarez, P. J. J. (2011). Occurrence and transport of tetracycline, sulfonamide, quinolone, and macrolide antibiotics in the Haihe River basin, China. Environmental Science & Technology, 45(5), 1827–1833. doi:10.1021/es104009s
- Lv, L., Jiang, T., Zhang, S., & Yu, X. (2014). Exposure to mutagenic disinfection byproducts leads to increase of antibiotic resistance in Pseudomonas aeruginosa. Environmental Science & Technology, 48(14), 8188–8195. doi:10.1021/es501646n
- Lv, L., Yu, X., Xu, Q., & Ye, C. (2015). Induction of bacterial antibiotic resistance by mutagenic halogenated nitrogenous disinfection byproducts. Environmental Pollution, 205, 291–298. doi:10.1016/j.envpol.2015.06.026
- Managaki, S., Murata, A., Takada, H., Bui, C. T., & Chiem, N. H. (2007). Distribution of macrolides, sulfonamides, and trimethoprim in tropical waters: Ubiquitous occurrence of veterinary antibiotics in the Mekong Delta. Environmental Science & Technology, 41(23), 8004–8010. doi:10.1021/es0709021
- Manaia, C. M. (2017). Assessing the risk of antibiotic resistance transmission from the environment to humans: Non-direct proportionality between abundance and risk. Trends in Microbiology, 25(3), 173–181. doi:10.1016/j.tim.2016.11.014
- Mantilla-Calderon, D., Plewa, M. J., Michoud, G., Fodelianakis, S., Daffonchio, D., & Hong, P. Y. (2019). Water disinfection byproducts increase natural transformation rates of environmental DNA in Acinetobacter baylyi ADP1. Environmental Science & Technology, 53(11), 6520–6528. doi:10.1021/acs.est.9b00692
- Mao, D., Luo, Y., Mathieu, J., Wang, Q., Feng, L., Mu, Q., … Alvarez, P. J. J. (2014). Persistence of extracellular DNA in river sediment facilitates antibiotic resistance gene propagation. Environmental Science & Technology, 48(1), 71–78. doi:10.1021/es404280v
- Mao, D., Yu, S., Rysz, M., Luo, Y., Yang, F., Li, F., … Alvarez, P. J. J. (2015). Prevalence and proliferation of antibiotic resistance genes in two municipal wastewater treatment plants. Water Research, 85, 458–466. doi:10.1016/j.watres.2015.09.010
- Marti, E., Huerta, B., Rodríguez-Mozaz, S., Barceló, D., Marcé, R., & Balcázar, J. L. (2018). Abundance of antibiotic resistance genes and bacterial community composition in wild freshwater fish species. Chemosphere, 196, 115–119. doi:10.1016/j.chemosphere.2017.12.108
- Marti, E., Variatza, E., & Balcazar, J. L. (2014). The role of aquatic ecosystems as reservoirs of antibiotic resistance. Trends in Microbiology, 22(1), 36–41. doi:10.1016/j.tim.2013.11.001
- Martinez, J. L. (2014). General principles of antibiotic resistance in bacteria. Drug Discovery Today: Technologies, 11(1), 33–39. doi:10.1016/j.ddtec.2014.02.001
- Martínez, J. L. (2012). Bottlenecks in the transferability of antibiotic resistance from natural ecosystems to human bacterial pathogens. Frontiers in Microbiology, 2, 265. doi:10.3389/fmicb.2011.00265
- Melnyk, A. H., Wong, A., & Kassen, R. (2015). The fitness costs of antibiotic resistance mutations. Evolutionary Applications, 8(3), 273–283. doi:10.1111/eva.12196
- Miranda, C. D., Godoy, F. A., & Lee, M. R. (2018). Current status of the use of antibiotics and the antimicrobial resistance in the Chilean salmon farms. Frontiers in Microbiology, 9(JUN), 1–14. doi:10.3389/fmicb.2018.01284
- Miranda, C. D., & Zemelman, R. (2001). Antibiotic resistant bacteria in fish from the Concepcion Bay, Chile. Marine Pollution Bulletin, 42(11), 1096–1102. doi:10.1016/S0025-326X(01)00093-5
- Miura, T., Sano, D., Suenaga, A., Yoshimura, T., Fuzawa, M., Nakagomi, T., … Okabe, S. (2013). Histo-blood group antigen-like substances of human enteric bacteria as specific adsorbents for human noroviruses. Journal of Virology, 87(17), 9441–9451. doi:10.1128/JVI.01060-13
- Muniesa, M., Colomer-Lluch, M., & Jofre, J. (2013a). Could bacteriophages transfer antibiotic resistance genes from environmental bacteria to human-body associated bacterial populations? Mobile Genetic Elements, 3(4), e25847. doi:10.4161/mge.25847
- Muniesa, M., Colomer-Lluch, M., & Jofre, J. (2013b). Potential impact of environmental bacteriophages in spreading antibiotic resistance genes. Future Microbiology, 8(6), 739–751. doi:10.2217/fmb.13.32
- Munir, M., Wong, K., & Xagoraraki, I. (2011). Release of antibiotic resistant bacteria and genes in the effluent and biosolids of five wastewater utilities in Michigan. Water Research, 45(2), 681–693. doi:10.1016/j.watres.2010.08.033
- Muziasari, W. I., Pitkänen, L. K., Sørum, H., Stedtfeld, R. D., Tiedje, J. M., & Virta, M. (2017). The resistome of farmed fish feces contributes to the enrichment of antibiotic resistance genes in sediments below Baltic sea fish farms. Frontiers in Microbiology, 7(Jan), 2137. doi:10.3389/fmicb.2016.02137
- Nagachinta, S., & Chen, J. (2008). Transfer of class 1 integron-mediated antibiotic resistance genes from shiga toxin-producing Escherichia coli to a susceptible E. coli K-12 strain in storm water and bovine feces. Applied and Environmental Microbiology, 74(16), 5063–5067. doi:10.1128/AEM.00517-08
- Neela, F. A., Nonaka, L., Rahman, M. H., & Suzuki, S. (2009). Transfer of the chromosomally encoded tetracycline resistance gene tet(M) from marine bacteria to Escherichia coli and Enterococcus faecalis. World Journal of Microbiology and Biotechnology, 25(6), 1095–1101. doi:10.1007/s11274-009-0004-8
- Novo, A., André, S., Viana, P., Nunes, O. C., & Manaia, C. M. (2013). Antibiotic resistance, antimicrobial residues and bacterial community composition in urban wastewater. Water Research, 47(5), 1875–1887. doi:10.1016/j.watres.2013.01.010
- O’Flaherty, E., Borrego, C. M., Balcázar, J. L., & Cummins, E. (2018). Human exposure assessment to antibiotic-resistant Escherichia coli through drinking water. Science of the Total Environment, 616–617, 1356–1364. doi:10.1016/j.scitotenv.2017.10.180
- O’Flaherty, E., & Cummins, E. (2017). Antibiotic resistance in surface water ecosystems: Presence in the aquatic environment, prevention strategies, and risk assessment. Human and Ecological Risk Assessment: An International Journal, 23(2), 299–322. doi:10.1080/10807039.2016.1247254
- O’Flaherty, E., Membré, J.-M., & Cummins, E. (2018). Meta-analysis of the reduction of antibiotic-sensitive and antibiotic-resistant Escherichia coli as a result of low- and medium-pressure UV lamps. Water Science and Technology, 2017(2), 612–620. doi:10.2166/wst.2018.183
- O’Flaherty, E., Solimini, A. G., Pantanella, F., De Giusti, M., & Cummins, E. (2019). Human exposure to antibiotic resistant-Escherichia coli through irrigated lettuce. Environment International, 122(November 2018), 270–280. doi:10.1016/j.envint.2018.11.022
- O’Flaherty, E., Solimini, A., Pantanella, F., & Cummins, E. (2019). The potential human exposure to antibiotic resistant-Escherichia coli through recreational water. Science of the Total Environment, 650, 786–795. doi:10.1016/j.scitotenv.2018.09.018
- O'Neill, J. (2016). Tackling drug-resistant infectiong slobally: Final report and recommendations. The review on antimicrobial resistance.
- Ohore, O. E., Addo, F. G., Zhang, S., Han, N., & Anim-Larbi, K. (2019). Distribution and relationship between antimicrobial resistance genes and heavy metals in surface sediments of Taihu Lake, China. Journal of Environmental Sciences (Sciences), 77, 323–335. doi:10.1016/j.jes.2018.09.004
- Oliver, J. D., Dagher, M., & Linden, K. (2005). Induction of Escherichia coli and Salmonella typhimurium into the viable but nonculturable state following chlorination of wastewater. Journal of Water and Health, 3(3), 249–257. doi:10.2166/wh.2005.040
- Park, J.-H., Kim, Y.-J., Kim, B., & Seo, K.-H. (2018). Spread of multidrug-resistant Escherichia coli harboring integron via swine farm waste water treatment plant. Ecotoxicology and Environmental Safety, 149(July 2017), 36–42.
- Peak, N., Knapp, C. W., Yang, R. K., Hanfelt, M. M., Smith, M. S., Aga, D. S., & Graham, D. W. (2007). Abundance of six tetracycline resistance genes in wastewater lagoons at cattle feedlots with different antibiotic use strategies. Environmental Microbiology, 9(1), 143–151. doi:10.1111/j.1462-2920.2006.01123.x
- Pepper, I. L., Brooks, J. P., & Gerba, C. P. (2018). Antibiotic resistant bacteria in municipal wastes: Is there reason for concern? Environmental Science & Technology, 52(7), 3949–3959. doi:10.1021/acs.est.7b04360
- Perry, J. A., & Wright, G. D. (2013). The antibiotic resistance “mobilome”: Searching for the link between environment and clinic. Frontiers in Microbiology, 4, 138. doi:10.3389/fmicb.2013.00138
- Poirel, L., Kämpfer, P., & Nordmann, P. (2002). Chromosome-encoded ambler class a β-lactamase of Kluyvera georgiana, a probable progenitor of a subgroup of CTX-M extended-spectrum β-lactamases. Antimicrobial Agents and Chemotherapy, 46(12), 4038. doi:10.1128/AAC.46.12.4038-4040.2002
- Poirel, L., Rodriguez-Martinez, J.-M., Liard, A., Mammeri, H., & Nordmann, P. (2005). Origin of plasmid-mediated quinolone resistance determinant qnrA. Antimicrobial Agents and Chemotherapy, 49(8), 3523–3525. doi:10.1128/AAC.49.8.3523-3525.2005
- Proia, L., Anzil, A., Subirats, J., Borrego, C., Farrè, M., Llorca, M., … Servais, P. (2018). Antibiotic resistance in urban and hospital wastewaters and their impact on a receiving freshwater ecosystem. Chemosphere, 206, 70–82.
- Pruden, A., Pei, R., Storteboom, H., & Carlson, K. H. (2006). Antibiotic resistance genes as emerging contaminants: Studies in Northern Colorado. Environmental Science & Technology, 40(23), 7445–7450. doi:10.1021/es060413l
- Razavi, M., Marathe, N. P., Gillings, M. R., Flach, C. F., Kristiansson, E., & Joakim Larsson, D. G. (2017). Discovery of the fourth mobile sulfonamide resistance gene. Microbiome, 5(1), 160. doi:10.1186/s40168-017-0379-y
- Rizzo, L., Manaia, C., Merlin, C., Schwartz, T., Dagot, C., Ploy, M. C., … Fatta-Kassinos, D. (2013). Urban wastewater treatment plants as hotspots for antibiotic resistant bacteria and genes spread into the environment: A review. Science of the Total Environment, 447, 345–360. doi:10.1016/j.scitotenv.2013.01.032
- Roberts, M. C. (2005). Update on acquired tetracycline resistance genes. FEMS Microbiology Letters, 245(2), 195–203. doi:10.1016/j.femsle.2005.02.034
- Rodriguez-Mozaz, S., Chamorro, S., Marti, E., Huerta, B., Gros, M., Sànchez-Melsió, A., … Balcázar, J. L. (2015). Occurrence of antibiotics and antibiotic resistance genes in hospital and urban wastewaters and their impact on the receiving river. Water Research, 69, 234–242. doi:10.1016/j.watres.2014.11.021
- Rosenberg Goldstein, R., Micallef, S., Gibbs, S., He, X., George, A., Sapkota, A., … Sapkota, A. (2014). Occupational exposure to Staphylococcus aureus and Enterococcus spp. among spray irrigation workers using reclaimed water. International Journal of Environmental Research and Public Health, 11(4), 4340–4355. doi:10.3390/ijerph110404340
- Rosenfeld, W. D., & Zobell, C. E. (1947). Antibiotic production by marine microorganisms. Journal of Bacteriology, 54(3), 393–398.
- Rusin, P., & Gerba, C. P. (2001). Association of chlorination and UV irradiation to increase antibiotic resistance in bacteria. In G. W. Ware (Ed.), Reviews of environmental contamination and toxicology 171 (pp. 1–52). New York: Springer-Verlag.
- Salcedo, D. E., & Kim, S. (2017). Fate of tetracycline resistance in synthetic livestock carcass leachate for two years. Journal of Environmental Management, 187, 220–228. doi:10.1016/j.jenvman.2016.11.048
- Salyers, A. A., Gupta, A., & Wang, Y. (2004). Human intestinal bacteria as reservoirs for antibiotic resistance genes. Trends in Microbiology, 12(9), 412–416. doi:10.1016/j.tim.2004.07.004
- Sano, D., Amarasiri, M., Hata, A., Watanabe, T., & Katayama, H. (2016). Risk management of viral infectious diseases in wastewater reclamation and reuse: Review. Environment International, 91, 220–229. doi:10.1016/j.envint.2016.03.001
- Savage, V. J., Chopra, I., & O'Neill, A. J. (2013). Staphylococcus aureus biofilms promote horizontal transfer of antibiotic resistance. Antimicrobial Agents and Chemotherapy, 57(4), 1968–1970. doi:10.1128/AAC.02008-12
- Schmid-Hempel, P., & Frank, S. A. (2007). Pathogenesis, virulence, and infective dose. PLoS Pathogens, 3(10), e147. doi:10.1371/journal.ppat.0030147
- Segura, P. A., Takada, H., Correa, J. A., El Saadi, K., Koike, T., Onwona-Agyeman, S., … Yargeau, V. (2015). Global occurrence of anti-infectives in contaminated surface waters: Impact of income inequality between countries. Environment International, 80, 89–97. doi:10.1016/j.envint.2015.04.001
- Seiler, C., & Berendonk, T. U. (2012). Heavy metal driven co-selection of antibiotic resistance in soil and water bodies impacted by agriculture and aquaculture. Frontiers in Microbiology, 3(DEC), 1–10. doi:10.3389/fmicb.2012.00399
- Sharma, V. K., Johnson, N., Cizmas, L., McDonald, T. J., & Kim, H. (2016). A review of the influence of treatment strategies on antibiotic resistant bacteria and antibiotic resistance genes. Chemosphere, 150, 702–714. doi:10.1016/j.chemosphere.2015.12.084
- Shimizu, A., Takada, H., Koike, T., Takeshita, A., Saha, M., Rinawati, … Reungsang, A. (2013). Ubiquitous occurrence of sulfonamides in tropical Asian waters. Science of the Total Environment, 452–453, 108–115.
- Silva, R. F., Mendonça, S. C. M., Carvalho, L. M., Reis, A. M., Gordo, I., Trindade, S., & Dionisio, F. (2011). Pervasive sign epistasis between conjugative plasmids and drug-resistance chromosomal mutations. PLoS Genetics, 7(7), e1002181. doi:10.1371/journal.pgen.1002181
- Slipko, K., Reif, D., Wögerbauer, M., Hufnagl, P., Krampe, J., & Kreuzinger, N. (2019). Removal of extracellular free DNA and antibiotic resistance genes from water and wastewater by membranes ranging from microfiltration to reverse osmosis. Water Research, 164, 114916. doi:10.1016/j.watres.2019.114916
- Søraas, A., Sundsfjord, A., Sandven, I., Brunborg, C., & Jenum, P. A. (2013). Risk factors for community-acquired urinary tract infections caused by ESBL-producing Enterobacteriaceae - A case-control study in a low prevalence country. PLoS One, 8(7), e69581. doi:10.1371/journal.pone.0069581
- Stachler, E., Crank, K., & Bibby, K. (2019). Co-occurrence of crAssphage with antibiotic resistance genes in an impacted urban watershed. Environmental Science & Technology Letters, 6(4), 216–221. doi:10.1021/acs.estlett.9b00130
- Stalder, T., Press, M. O., Sullivan, S., Liachko, I., & Top, E. M. (2019). Linking the resistome and plasmidome to the microbiome. The ISME Journal, 13, 2437–2446. doi:10.1038/s41396-019-0446-4
- Stewart, G. J., & Sinigalliano, C. D. (1991). Exchange of chromosomal markers by natural transformation between the soil isolate, Pseudomonas stutzeri JM300, and the marine isolate, Pseudomonas stutzeri strain ZoBell. Antonie Van Leeuwenhoek, 59(1), 19–25. doi:10.1007/BF00582115
- Strachan, N. J. C., Dunn, G. M., Locking, M. E., Reid, T. M. S., & Ogden, I. D. (2006). Escherichia coli O157: Burger bug or environmental pathogen?. International Journal of Food Microbiology, 112(2), 129–137. doi:10.1016/j.ijfoodmicro.2006.06.021
- Su, H.-C., Liu, Y.-S., Pan, C.-G., Chen, J., He, L.-Y., & Ying, G.-G. (2018). Persistence of antibiotic resistance genes and bacterial community changes in drinking water treatment system: From drinking water source to tap water. Science of the Total Environment, 616–617, 453–461. doi:10.1016/j.scitotenv.2017.10.318
- Suzuki, S., Kimura, M., Agusa, T., & Rahman, H. M. (2012). Vanadium accelerates horizontal transfer of tet(M) gene from marine Photobacterium to Escherichia coli. FEMS Microbiology Letters, 336(1), 52–56. doi:10.1111/j.1574-6968.2012.02653.x
- Suzuki, S., Nakanishi, S., Tamminen, M., Yokokawa, T., Sato-Takabe, Y., Ohta, K., … Virta, M. (2019). Occurrence of sul and tet(M) genes in bacterial community in Japanese marine aquaculture environment throughout the year: Profile comparison with Taiwanese and Finnish aquaculture waters. Science of the Total Environment, 669, 649–656. doi:10.1016/j.scitotenv.2019.03.111
- Suzuki, S., Ogo, M., Miller, T. W., Shimizu, A., Takada, H., & Siringan, M. A. T. (2013). Who possesses drug resistance genes in the aquatic environment?: Sulfamethoxazole (SMX) resistance genes among the bacterial community in water environment of Metro-Manila, Philippines. Frontiers in Microbiology, 4, 102. doi:10.3389/fmicb.2013.00102
- Suzuki, S., Pruden, A., Virta, M., & Zhang, T. (2017). Editorial: Antibiotic resistance in aquatic systems. Frontiers in Microbiology, 8, 14. doi:10.3389/fmicb.2017.00014
- Syrova, E., Kohoutova, L., Dolejska, M., Papezikova, I., Kutilova, I., Cizek, A., … Palikova, M. (2018). Antibiotic resistance and virulence factors in mesophilic Aeromonas spp. from Czech carp fisheries. Journal of Applied Microbiology, 125(6), 1702–1713. doi:10.1111/jam.14075
- Szekeres, E., Baricz, A., Chiriac, C. M., Farkas, A., Opris, O., Soran, M.-L., … Coman, C. (2017). Abundance of antibiotics, antibiotic resistance genes and bacterial community composition in wastewater effluents from different Romanian hospitals. Environmental Pollution, 225, 304–315. doi:10.1016/j.envpol.2017.01.054
- Szekeres, E., Chiriac, C. M., Baricz, A., Szőke-Nagy, T., Lung, I., Soran, M.-L., … Coman, C. (2018). Investigating antibiotics, antibiotic resistance genes, and microbial contaminants in groundwater in relation to the proximity of urban areas. Environmental Pollution, 236, 734–744. doi:10.1016/j.envpol.2018.01.107
- Takasu, H., Suzuki, S., Reungsang, A., & Viet, P. H. (2011). Fluoroquinolone (FQ) contamination does not correlate with occurrence of FQ-resistant bacteria in aquatic environments of Vietnam and Thailand. Microbes and Environments, 26(2), 135–143. doi:10.1264/jsme2.ME10204
- Tan, L., Li, L., Ashbolt, N., Wang, X., Cui, Y., Zhu, X., … Luo, Y. (2018). Arctic antibiotic resistance gene contamination, a result of anthropogenic activities and natural origin. Science of the Total Environment, 621, 1176–1184. doi:10.1016/j.scitotenv.2017.10.110
- Tan, Q., Li, W., Zhang, J., Zhou, W., Chen, J., Li, Y., & Ma, J. (2019). Presence, dissemination and removal of antibiotic resistant bacteria and antibiotic resistance genes in urban drinking water system: A review. Frontiers of Environmental Science and Engineering, 13(3), 36. doi: 10.1007/s11783-019-1120-9
- Tao, W., Zhang, X.-X., Zhao, F., Huang, K., Ma, H., Wang, Z., … Ren, H. (2016). High levels of antibiotic resistance genes and their correlations with bacterial community and mobile genetic elements in pharmaceutical wastewater treatment bioreactors. PloS One, 11(6), e0156854. doi:10.1371/journal.pone.0156854
- Thomas, C. M., & Nielsen, K. M. (2005). Mechanisms of, and barriers to, horizontal gene transfer between bacteria. Nature Reviews Microbiology, 3(9), 711–721. doi:10.1038/nrmicro1234
- Tomova, A., Ivanova, L., Buschmann, A. H., Godfrey, H. P., & Cabello, F. C. (2018). Plasmid-mediated quinolone resistance (PMQR) genes and class 1 integrons in quinolone-resistant marine bacteria and clinical isolates of Escherichia coli from an aquacultural Area. Microbial Ecology, 75(1), 104–112. doi:10.1007/s00248-017-1016-9
- Tomova, A., Ivanova, L., Buschmann, A. H., Rioseco, M. L., Kalsi, R. K., Godfrey, H. P., & Cabello, F. C. (2015). Antimicrobial resistance genes in marine bacteria and human uropathogenic Escherichia coli from a region of intensive aquaculture. Environmental Microbiology Reports, 7(5), 803–809. doi:10.1111/1758-2229.12327
- Tong, J., Tang, A., Wang, H., Liu, X., Huang, Z., Wang, Z., … Zhang, Y. (2019). Microbial community evolution and fate of antibiotic resistance genes along six different full-scale municipal wastewater treatment processes. Bioresource Technology, 272(September 2018), 489–500. doi:10.1016/j.biortech.2018.10.079
- Torti, A., Lever, M. A., & Jørgensen, B. B. (2015). Origin, dynamics, and implications of extracellular DNA pools in marine sediments. Marine Genomics, 24, 185–196. doi:10.1016/j.margen.2015.08.007
- Tortorella, E., Tedesco, P., Esposito, F. P., January, G. G., Fani, R., Jaspars, M., & De Pascale, D. (2018). Antibiotics from deep-sea microorganisms: Current discoveries and perspectives. Marine Drugs, 16(10), 355. doi:10.3390/md16100355
- Vogwill, T., & Maclean, R. C. (2015). The genetic basis of the fitness costs of antimicrobial resistance: A meta-analysis approach. Evolutionary Applications, 8(3), 284–295. doi:10.1111/eva.12202
- Wang, G., Wilson, T. J. M., Jiang, Q., & Taylor, D. E. (2001). Spontaneous mutations that confer antibiotic resistance in Helicobacter pylori. Antimicrobial Agents and Chemotherapy, 45(3), 727–733. doi:10.1128/AAC.45.3.727-733.2001
- Wang, Q., Wang, P., & Yang, Q. (2018). Occurrence and diversity of antibiotic resistance in untreated hospital wastewater. Science of the Total Environment, 621, 990–999. doi:10.1016/j.scitotenv.2017.10.128
- Wang, Q., Mao, D., Mu, Q., & Luo, Y. (2015). Enhanced horizontal transfer of antibiotic resistance genes in freshwater microcosms induced by an ionic liquid. PLoS One, 10(5), e0126784. doi:10.1371/journal.pone.0126784
- Wang, Y., Lu, J., Mao, L., Li, J., Yuan, Z., Bond, P. L., & Guo, J. (2019). Antiepileptic drug carbamazepine promotes horizontal transfer of plasmid-borne multi-antibiotic resistance genes within and across bacterial genera. The Isme Journal, 13(2), 509–522. doi:10.1038/s41396-018-0275-x
- Wellington, E. M., Boxall, A. B. A., Cross, P., Feil, E. J., Gaze, W. H., Hawkey, P. M., … Williams, A. P. (2013). The role of the natural environment in the emergence of antibiotic resistance in Gram-negative bacteria. The Lancet Infectious Diseases, 13(2), 155–165. doi:10.1016/S1473-3099(12)70317-1
- Winkworth, C. L. (2013). Antibiotic resistance genes in freshwater biofilms along a whole river. Journal of Water and Health, 11(2), 186–198. doi:10.2166/wh.2013.223
- Wistrand-Yuen, E., Knopp, M., Hjort, K., Koskiniemi, S., Berg, O. G., & Andersson, D. I. (2018). Evolution of high-level resistance during low-level antibiotic exposure. Nature Communications, 9(1), 1599. doi:10.1038/s41467-018-04059-1
- Woodford, N., & Ellington, M. J. (2007). The emergence of antibiotic resistance by mutation. Clinical Microbiology and Infection, 13(1), 5–18. doi:10.1111/j.1469-0691.2006.01492.x
- World Health Organization. (2017a). Global antimicrobial resistance surveillance system (GLASS) report: Early implementation 2016-2017. Geneva.
- World Health Organization. (2017b). List of bacteria for which new antibiotics are urgently needed. Retrieved March 9, 2018, from .
- World Health Organization. (2018). Global antimicrobial resistance surveillance system (GLASS) report: Early implementation 2017-2018. Geneva. ISBN978-92-4-151344-9
- Xu, J., Xu, Y., Wang, H., Guo, C., Qiu, H., He, Y., … Meng, W. (2015). Occurrence of antibiotics and antibiotic resistance genes in a sewage treatment plant and its effluent-receiving river. Chemosphere, 119, 1379–1385. doi:10.1016/j.chemosphere.2014.02.040
- Xu, J., Sheng, G. P., Ma, Y., Wang, L. F., & Yu, H. Q. (2013). Roles of extracellular polymeric substances (EPS) in the migration and removal of sulfamethazine in activated sludge system. Water Research, 47(14), 5298–5306. doi:10.1016/j.watres.2013.06.009
- Xu, L., Ouyang, W., Qian, Y., Su, C., Su, J., & Chen, H. (2016). High-throughput profiling of antibiotic resistance genes in drinking water treatment plants and distribution systems. Environmental Pollution, 213, 119–126. doi:10.1016/j.envpol.2016.02.013
- Yang, D., Wang, J., Qiu, Z., Jin, M., Shen, Z., Chen, Z., … Li, J.-W. (2013). Horizontal transfer of antibiotic resistance genes in a membrane bioreactor. Journal of Biotechnology, 167(4), 441–447. doi:10.1016/j.jbiotec.2013.08.004
- Yang, F., Zhang, K., Zhi, S., Li, J., Tian, X., Gu, Y., & Zhou, J. (2019). High prevalence and dissemination of β-lactamase genes in swine farms in northern China. Science of the Total Environment, 651, 2507–2513. doi:10.1016/j.scitotenv.2018.10.144
- Yang, Q., Ren, S., Niu, T., Guo, Y., Qi, S., Han, X., … Pan, F. (2014). Distribution of antibiotic-resistant bacteria in chicken manure and manure-fertilized vegetables. Environmental Science and Pollution Research, 21(2), 1231–1241. doi:10.1007/s11356-013-1994-1
- You, X., Wu, D., Wei, H., Xie, B., & Lu, J. (2018). Fluoroquinolones and β-lactam antibiotics and antibiotic resistance genes in autumn leachates of seven major municipal solid waste landfills in China. Environment International, 113, 162. doi:10.1016/j.envint.2018.02.002
- Yu, Z., He, P., Shao, L., Zhang, H., & Lü, F. (2016). Co-occurrence of mobile genetic elements and antibiotic resistance genes in municipal solid waste landfill leachates: A preliminary insight into the role of landfill age. Water Research, 106, 583–592. doi:10.1016/j.watres.2016.10.042
- Yuan, J., Ni, M., Liu, M., Zheng, Y., & Gu, Z. (2019). Occurrence of antibiotics and antibiotic resistance genes in a typical estuary aquaculture region of Hangzhou Bay, China. Marine Pollution Bulletin, 138, 376–384. doi:10.1016/j.marpolbul.2018.11.037
- Zhang, A., Call, D. R., Besser, T. E., Liu, J., Jones, L., Wang, H., & Davis, M. A. (2019). β-lactam resistance genes in bacteriophage and bacterial DNA from wastewater, river water, and irrigation water in Washington State. Water Research, 161, 335–340. doi:10.1016/j.watres.2019.06.026
- Zhang, M., Chen, S., Yu, X., Vikesland, P., & Pruden, A. (2019). Degradation of extracellular genomic, plasmid DNA and specific antibiotic resistance genes by chlorination. Frontiers of Environmental Science & Engineering, 13(3), 38. doi:10.1007/s11783-019-1124-5
- Zhang, S., Ye, C., Lin, H., Lv, L., & Yu, X. (2015). UV disinfection induces a VBNC state in Escherichia coli and Pseudomonas aeruginosa. Environmental Science & Technology, 49(3), 1721–1728. doi:10.1021/es505211e
- Zhang, S., Wang, Y., Song, H., Lu, J., Yuan, Z., & Guo, J. (2019). Copper nanoparticles and copper ions promote horizontal transfer of plasmid-mediated multi-antibiotic resistance genes across bacterial genera. Environment International, 129(April), 478–487. doi:10.1016/j.envint.2019.05.054
- Zhang, Y., Gu, A. Z., Cen, T., Li, X., He, M., Li, D., & Chen, J. (2018). Sub-inhibitory concentrations of heavy metals facilitate the horizontal transfer of plasmid-mediated antibiotic resistance genes in water environment. Environmental Pollution, 237, 74–82. doi:10.1016/j.envpol.2018.01.032
- Zhang, Y., Gu, A. Z., He, M., Li, D., & Chen, J. (2017). Subinhibitory concentrations of disinfectants promote the horizontal transfer of multidrug resistance genes within and across genera. Environmental Science & Technology, 51(1), 570–580. doi:10.1021/acs.est.6b03132
- Zhang, Y., Niu, Z., Zhang, Y., & Zhang, K. (2018). Occurrence of intracellular and extracellular antibiotic resistance genes in coastal areas of Bohai Bay (China) and the factors affecting them. Environmental Pollution, 236, 126–136. doi:10.1016/j.envpol.2018.01.033
- Zhao, R., Feng, J., Liu, J., Fu, W., Li, X., & Li, B. (2019). Deciphering of microbial community and antibiotic resistance genes in activated sludge reactors under high selective pressure of different antibiotics. Water Research, 151, 388–402. doi:10.1016/j.watres.2018.12.034
- Zhao, Z., Wang, J., Han, Y., Chen, J., Liu, G., Lu, H., … Chen, S. (2017). Nutrients, heavy metals and microbial communities co-driven distribution of antibiotic resistance genes in adjacent environment of mariculture. Environmental Pollution, 220, 909–918. doi:10.1016/j.envpol.2016.10.075
- Zhu, Y.-G., Zhao, Y., Li, B., Huang, C.-L., Zhang, S.-Y., Yu, S., … Su, J.-Q. (2017). Continental-scale pollution of estuaries with antibiotic resistance genes. Nature Microbiology, 2(4), 16270. doi:10.1038/nmicrobiol.2016.270