Abstract
Engineered nanomaterials (NMs) are increasingly released into the environment with the rapid development of nanotechnology. In this review, we present the current understanding on the safety of NMs in the environment from the existing literature. For the four typical NMs selected (i.e., TiO2, Ag, CuO, graphene), available data on both environmental concentrations and ecotoxicity (e.g., predicted no effect concentrations [PNECs]) were collected from the published literature. All the four typical NMs did not pose a high risk by comparing environmental concentrations and PNECs. Toxicity of NMs as affected by environmental factors was further discussed. It can be concluded that nanotoxicity could be further lowered under environmental conditions for most scenarios. Generally, the environmental risk of NMs is of low concern currently, while the risk in specific scenarios such as surface water and soil near the point sources (e.g., wastewater effluent, sewage sludge) should be paid more attention. Moreover, the NM-induced toxicity could be changed by various environmental factors such as sunlight irradiation, natural organic matter, and mineral particles. In view of the uncertainties on environmental concentrations and ecotoxicity, the challenges on comprehensive understanding on the environmental risks of NMs, and the future research perspectives were addressed and presented.
Graphical abstract
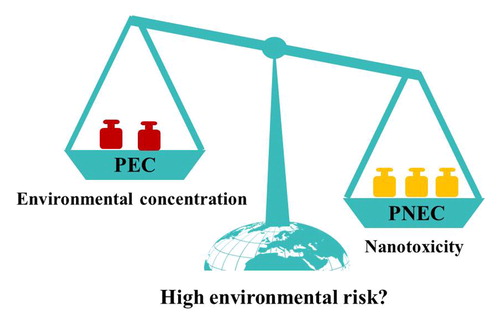
1. Introduction
As nanotechnology rapidly develops, the categories and numbers of nano-enabled products are drastically increasing in the last two decades. Based on the data in “The Nanodatabase” in 2019, there are 3111 nano-enabled products, which could be divided into seven categories including “Health and Fitness,” “Home and Garden,” “Automotive,” “Food and Beverage,” “Electronics and Computers,” “Appliances,” and “Goods For Children” () (The Nanodatabase, Citation2019). “Nanotechnology Products Database” includes 8902 nano-enabled products in 2019, and the top eight categories were “Cosmetics,” “Textile,” “Construction,” “Medicine,” “Automotive,” “Environment,” “Renewable Energies,” and “Petroleum” (Statnano, Citation2019). Among these categories, Ag, Ti, and C-based nanomaterials (NMs) are the most commonly-used NMs for these products (). As a result, NMs are releasing into air, water, and soil during the production, consumption, and disposal of nano-enabled products (Giese et al., Citation2018; Sun et al., Citation2016). It is of importance to assess the environmental risk of NMs, and possible threats to the health of ecosystems.
Figure 1. Nano-enabled product categories in 2019. (a) Main application fields of nano-enabled products; (b) number of nano-enabled products with different materials. Data from “The Nanodatabase” (December 1, 2019).
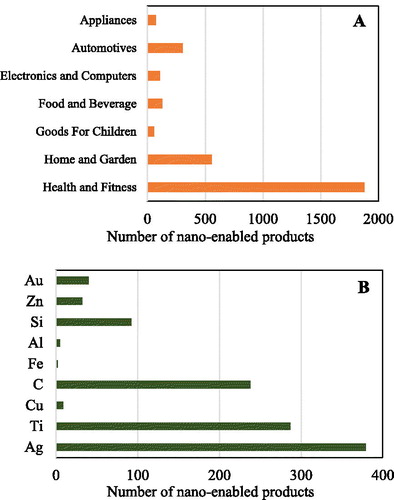
Concerns over the toxicity of NMs have been raised since the early 2000s. At the first decade, the toxicological researches mainly focused on (1) the toxicity of different NMs to both model and unique native organisms; (2) the acquisition of various toxicity parameters, such as no observable effect concentration (NOEC), the lowest observed effect concentration (LOEC), median effect concentration (EC50), and lethal concentration (LC50); (3) the effects of texture, size, surface properties on NM toxicity; and (4) the toxicity of NMs at organismal and cellular levels (Auffan et al., Citation2009; Mahmoudi et al., Citation2011; Moore, Citation2006; Nel et al., Citation2006). At the second decade, the following aspects are further addressed: (1) prediction and measurement of the concentrations of NMs in different environmental compartments; (2) toxicity of NMs as affected by environmental factors; and (3) mechanistic investigations of NM (especially the novel NMs) toxicity at molecular levels. To date, there are already numerous of works on toxicological effects of NMs. However, the understanding of the environmental risks of NMs still lags behind the release of new nano-enabled products and the developments of new applications (Giese et al., Citation2018). There is still no consensus on the ecotoxicity of NMs, and a number of data and knowledge gaps remain apparent. The environmental scientists attempted to answer the question: are the NMs safe to use? Two aspects: (1) the actual environmental concentrations, and (2) the toxicity of NMs in realistic environments, are essential to answer this question. But there are still many different views and opinions, including the validation of both the prediction and measurement of environmental concentrations in different environmental compartments, the accuracy on the toxicological investigation approaches; and the reliability for the toxicity of NMs in environments (Arvidsson, Citation2018; Musee, Citation2018). Therefore, this work will focus on the above two aspects to improve the understanding on the environmental risks of NMs.
In this review, four typical NMs including TiO2 (high production and environmental concentrations), Ag (high production and toxicity), CuO (high toxicity), and graphene (rapidly increasing production) are mainly considered. For environmental concentrations, the predicted and measured concentrations of NMs in different environmental compartments will be discussed and summarized; For NM toxicity, the toxicological data will be mainly from the newly-released publications in the past five years because there are several important critical reviews that summarized the ecotoxicity of NMs by 2014 (Bour et al., Citation2015).The toxicity mechanism of NMs will not be discussed in detail in the present work because it has been recently well-reviewed (Buchman et al., Citation2019). Instead, the effects of environmental relevant conditions on NM toxicity will be emphasized.
2. Environmental concentrations of NMs
Environmental concentrations of NMs could be obtained by both prediction and measurement (Wimmer et al., Citation2019). This section summarizes the predicted environmental concentrations (PECs) and measured environmental concentrations (MECs) of NMs. PECs and MECs of four typical NMs in a same environmental compartment are compared.
2.1. Model prediction of NMs concentration in water, sediment, and soil
Mathematical modeling is currently one of the most important tools to obtain the environmental concentrations of NMs in different environmental compartments. PEC of NMs was first calculated a decade ago (Gottschalk et al., Citation2009; Mueller & Nowack, Citation2008). Afterwards, PECs of various types of NMs in different regions/countries were reported. In this review, we summarized reported PECs of TiO2, Ag, CuO, and carbon based NMs (e.g., CNTs) in water, sediment, and soil (Table S1), and the PEC results are presented as a box and whiskers plot ().
Figure 2. Box and Whisker plots for modeled environmental concentrations of TiO2, Ag, CuO, and carbon based NMs in water (a), sediment (b), and soil (c). The diagrams were generated based on the modeled concentration values of NMs published in previous studies (Table S1).
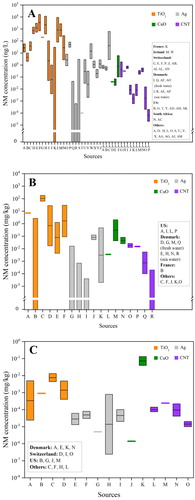
For PECs in waters, TiO2, Ag, CuO, and CNT NMs lie in the ranges at 0–16,000, 0–619, 0.02–6, and 2 × 10−5–1.82 ng/L, respectively (, Table S1). The ranges are quite wide, which are due to (1) the fitting data from different models; (2) the data from the surface waters in different regions/countries; and (3) different distribution properties of NMs between freshwater and seawater (Table S1). Generally, TiO2 NMs had higher PECs than the other three NMs, probably due to their highest production volume and widespread applications in the world (Arvidsson et al., Citation2018; Holden et al., Citation2014). CNTs showed the lowest PECs in surface water, which could be explained by the lowest production volume among the four NMs (Arvidsson et al., Citation2018). The high surface hydrophobicity of CNTs could induce strong aggregation and sediments (Zhao et al., Citation2012), which may be another reason for the low PECs in surface water. In addition, all the PEC data are from European countries, US and South Africa, and there is no information from the surface waters in Asian countries (). Considering that the nano-product volumes in Asian countries (e.g., China) are rapidly increasing (The Nanodatabase, Citation2019), more efforts are needed to predict the environmental concentrations in the rivers and lakes in these countries. Moreover, the PEC information from seawaters is more limited than freshwater (). NMs in seawater are mainly transported from terrestrial and coastal areas (Matranga & Corsi, Citation2012). The PECs of NMs in seawater are generally lower than those in freshwater, which could be attributed to (1) high ionic strength-induced aggregation and sedimentation of NMs in seawater; and (2) high mass transport and diffusion of NMs from coastal seawater to the ocean.
In sediments, TiO2, Ag, CuO, and CNT NMs are ranged at 0–186, 0–0.47, 3.5 × 10−3–2.1, and 1 × 10−4–2.66 × 10−2 mg/kg, respectively (, Table S1). For all the four NMs, PECs in sediments are higher than those in surface waters. The reason is that NMs released into waters could quickly adsorb to the suspended minerals, finally depositing into the sediment environments (Wimmer et al., Citation2019). Actually, sediment is the compartment in which most NMs entering surface water end up (Sun et al., Citation2016). In addition, PECs in seawater sediments are lower than those in freshwater sediments, which is likely due to lower PECs in seawater than freshwater ().
In soils, PECs for all the NMs are 2–4 orders of magnitude lower than that in sediments (). TiO2 NMs generally show the highest PECs in soils among the NMs, which is consistent with those in sediments and surface water. However, a high PEC for CuO NMs was detected in soil in Denmark, with the concentration at 0.039–0.13 mg/kg (). The high PECs of CuO NMs were explained by the wide usage of CuO and CuCO3 NMs as wood preservatives which could be released into the soils (Gottschalk et al., Citation2015). It is noted that the available PEC data in soil are relatively fewer than the other two compartments. All the PECs in soils are obtained from Europe and US ().
For all the environmental compartments (surface water, sediment, and soil), there is no PEC information on graphene NMs. Further modeling investigations on graphene-based NMs including few-layer graphene, graphene oxide (GO), reduced GO are suggested. It is reported that the total input production volume of graphene is 6–7 times higher than CNTs (Arvidsson et al., Citation2018). Considering that the surface properties, applications and environmental fates of graphene are similar to CNTs, PECs of graphene NMs are speculated to be at a similar concentration level with (or even higher than) CNTs.
Sun et al. (Citation2016) predicted the NM concentrations in both waste streams and environmental compartments in the EU in 2014. It was found that the highest PECs (“mg/kg” level) were in wastewater treatment plant (WWTP) sludge, followed by solid waste, bottom ash of waste incineration plant (WIP), and WIP fly ash. For the soil compartments, WWTP sludge-treated soil had much higher PECs than untreated soil. For TiO2 NMs, PECs of “WIP bottom ash,” “WIP fly ash,” and “sludge treated soil” were higher than that those in sediments. For Ag NMs, PECs of “solid waste to landfill,” “WIP bottom ash,” “WIP fly ash” were higher than that in sediments. In another report, for all the test NMs (TiO2, Ag and CNTs), PECs in WWTP effluent were higher than that in surface water (Gottschalk et al., Citation2010). The same finding was also observed in another case (Gottschalk et al., Citation2009). PECs in WWTP sludge were very high, with the concentrations at 211 mg/kg for TiO2, 0.069 mg/kg for CNT, and 1.88 mg/kg for Ag NMs (Gottschalk et al., Citation2010), which are higher than those in sediments as shown in . TiO2 NMs showed higher PECs in WWTP effluent and WWTP sludge than Ag and CNT NMs (Gottschalk et al., Citation2010), and this is consistent with the order of NMs in environmental compartments (surface water, soil, and sediments) (). These findings suggest that more attention should be paid to the potential environmental risk of NMs in WWTP effluents and WWTP sludges, as well as the surface waters near the discharge of WWTP effluents and the soils after sludge treatment.
2.2. Measurements of NMs concentration in surface water, sediment, and soil
The released NMs in the environment were first detected by transmission electron microscopy/energy dispersive X-ray analysis (TEM/EDX) and inductively coupled plasma-mass spectrometry (ICP-MS) (Kägi et al., Citation2008). Currently, electrothermal atomic absorption spectrometry (ET-AAS) (Li et al., Citation2016), asymmetric-flow field flow fractionation with inductively coupled plasma–mass spectrometry (AF4-ICP-MS) (Hoque et al., Citation2012), and especially, single particle inductively coupled plasma-mass spectrometry (sp-ICP-MS) (Loula et al., Citation2019; Peters et al., Citation2018; Wu et al., Citation2020; Xiao et al., Citation2019) have been developed for characterization and quantification of NMs in natural environments. summarized the MECs of NMs in surface water, and sediment based on the published data (Table S2).
Figure 3. Box and Whisker plots for measured concentrations of nanoparticulate Ti, Ag, and Cu in surface waters (a) and sediments (b). The diagrams were generated based on the measured concentration values of NMs published in previous studies (Table S2).
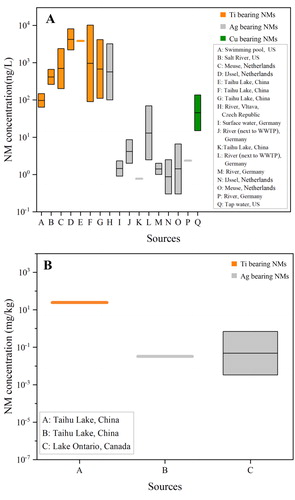
In surface waters, MECs of Ti-, Ag-, and Cu-based NMs are ranged at 64–10,200, 0.3–3200, and 15–136 ng/L (tap water), respectively (). Ti-based NMs had the highest MECs among the three NMs, consistent with the PECs (). MECs of Ti- and Ag-based NMs are at the upper level of their PEC ranges. It is noted that there is only one report having the measured mass concentrations of Cu-based NMs in water (tap water), and the obtained MEC exceeded the maximum value of the PEC range (Venkatesan et al., Citation2018). The collected MEC data in surface water are fewer than PECs for all the NMs. The waters were sampled from rivers, lakes, swimming pool, and tap water (Table S2). There is only one report focusing on the MECs of NMs in seawater, and nanoparticulate Ti and Ag concentrations in seawater were 0.65 × 107 and 0.54 × 107 particles/mL (Xu et al., Citation2020). In addition, the MECs are not available for CNT, graphene or other carbon NMs. This is because (1) it is hard to distinguish specific carbon-based NMs from natural carbonaceous materials, and (2) there is no detection technique that could satisfy the accuracy and detection limits of carbon-based NMs at environmentally relevant concentrations (ERCs) (Goodwin et al., Citation2018). In addition, the MECs data are from European countries, North America, and China (Table S2), while no information is found from African countries and Australia ().
Few data for MECs in sediments were reported (). Ti- and Ag-based NMs were at 24.7, and 0.0033–0.2 mg/kg, respectively (Metcalfe et al., Citation2018; Xiao et al., Citation2019). MECs of Ti- and Ag-based NMs are in the range of PECs, but tend to be at the upper level of the ranges, which could be attributed to the strong backgrounds of Ti and Ag, and the presence of naturally occurring nanoparticles (Garner et al., Citation2017). To date, there are very few data on the MECs in soils due to the limitations on NM extraction and separation methods: (1) the extraction process and combined separation techniques (e.g., filtration, centrifugation) could change the physicochemical properties (e.g., dissolution, aggregation) of NMs; (2) the interactions of NMs with soil particles limit the tracking and detecting of low-concentration NMs; and (3) soil organic matter, particularly at high content, could highly impact the feasibility of the NM extraction approach for sp-ICP-MS analysis (Li et al., Citation2019; Mahdi et al., Citation2017). Mahdi et al. (Citation2017) extracted the spiking Ag NMs from soils by using the combined methods of prewetting and aqueous extraction, and the recovery of Ag NMs (60 nm) was only 32% for sandy soil and 46% for clayey soil. Li et al. (Citation2019) optimized the extraction approach, and analyzed Ag NMs in soils (Changsha, China) using sp-ICP-MS. It is found that the recovery for Ag NMs was 59.6%–87.4%, and the MECs of Ag NMs (>30 nm) were in the range of (1–3) ×107 particles/g soil.
Li et al. (Citation2016) examined the MECs of Ag-based NMs in wastewater influent and effluent from a WWTP in Germany, in which 10.1–357, and 0.7–11.1 ng/L of Ag-based NMs were detected, demonstrating that most of Ag-based NMs could be removed in the WWTP. However, the effluent of Ag-based NMs still resulted in a high concentration range (2.0–8.6 ng/L) in the river near the WWTP, which was higher than the nearby uncontaminated surface waters (Li et al., Citation2016). In another report, Ag-based NMs from the water samples near WWTP were determined to be 2.47–69.08 ng/L, much higher than the level of the river water (1–2 ng/L) (Wimmer et al., Citation2019). The above findings suggested that WWTPs could act as point sources for the NMs in surface water and sediments. Tou et al. (Citation2017) sampled the sewage sludge from Shanghai (China) for sp-ICP-MS analysis, and found that the metal-based NMs (Ti, Fe, Zn, Sn, and Pb) were at the range of 107–1011 particles/g, and Ti-based NMs were at 1.51 × 109 particles/g. Although there is no available information on the concentrations of NMs in sludge-treated soils, the MECs are speculated to be much higher than the pristine soils.
3. Environmental risk of NMs
3.1. Risk assessment of NMs in different environmental compartments
To better understand the environmental risk, a classic risk assessment by comparing PECs with predicted no effect concentrations (PNECs) was commonly used (Arvidsson, Citation2018; Coll et al., Citation2016). We thus collected NOEC or LOEC values of TiO2, Ag, CuO and graphene NMs from the latest ecotoxicological literature (2014–2019) (Tables S3–S6), and obtained the PNECs from the species sensitivity distribution (SSD). Based on the SSD analysis, the calculated PNECs for TiO2, Ag, CuO, and graphene-based NMs in aquatic environments were 1.92 × 10−1, 4.85 × 10−5, 9.06 × 10−3, and 1.15 × 10−2 mg/L, respectively (), clearly suggesting the lowest toxicity for TiO2 NMs, and the highest toxicity for Ag NMs in aquatic environments. Coll et al. (Citation2016) reported the PNECs from the most sensitive to least sensitive in the order of Ag (1.7 × 10−5 mg/L) ≪ TiO2 (15.7 × 10−3 mg/L) ≪ (CNT 55.6 × 10−3 mg/L), and Chen et al. (Citation2018) reported the PNECs by following an order: Ag (0.0036 mg/L) < CuO (0.049 mg/L) < TiO2 (3.1 mg/L), which are consistent with this work (). The reported PNECs of these four NMs in WWTP effluent, sediment and soil compartments were also collected from the published literature (Table S7), and these PNECs were in a wide range for each NMs, indicating the high uncertainties during ecotoxicological investigations. In addition, most toxicological works focused on PNECs in aqueous and soil environments. The PNEC information in WWTP effluent and sediment environments is scarce.
Figure 4. Predicted no effect concentrations (PNECs) of TiO2 (a), Ag (b), CuO (c), and graphene (d) NMs in aquatic environments. Based on probabilistic species sensitivity distributions (PSSD) analysis, the calculated PNECs for TiO2, Ag, CuO, and graphene NMs are 1.92 (0.32–6.41) × 10−1, 4.85 (0.13–53.87) × 10−5, 9.06 (0.39–65.40) × 10−3, 1.15 (0.03–10.6) × 10−2 mg/L, respectively.
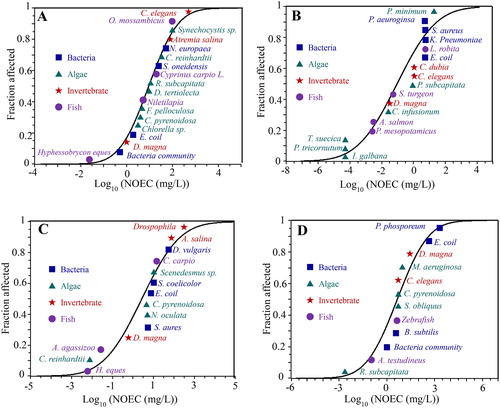
Risk characterization ratios (RCRs), i.e., the ratio of PEC to PNEC, are shown in Table S8. For most scenarios in surface water, RCR values were lower than 1, indicating low risk in aqueous environments. Only one report observed that the RCRs of Ag NMs in the surface waters of Europe were a bit higher than 1 (i.e., 1.1, 1.03) (Gottschalk et al., Citation2009). For soil compartments, RCRs for all the four NMs were much lower than 1, demonstrating that NMs would pose very low or no risk in soils. Only a few works focused on the environmental risk in sediments, in which the RCRs were all much lower than 1 (Table S8). Considering that RCR between 0.1 and 1 could be ranked as “medium risk,” Table S8 shows that most of RCRs were lower than 0.01, suggesting low risk in these environmental compartments. However, NMs in WWTP effluents may pose significant risk. For example, in some scenarios, the RCR values were higher than 1 in wastewater (effluent) for TiO2, Ag, and CuO NMs (Gottschalk et al., Citation2009; Kjølholt et al., Citation2015).
In addition to assessing the environmental risks of NMs using RCRs, other approaches were also employed. For example, besides NOEC at HC5 (5% of species will be harmed), LOEC, NOEC, LC50, EC50, and NOEC for single species are also able to be used for the risk assessment. Garner et al. (Citation2017) reported that under all release scenarios in fresh water, CuO NMs did not exceed the NOEC HC5, and TiO2 occasionally exceeded the NOEC HC5 in all scenarios (especially for the highest release scenario). In agricultural and urban soils, neither TiO2 nor CuO would exhibit any toxicity even under the most extreme release scenarios (Garner et al., Citation2017). Moreover, Arvidsson et al. (Citation2018) assessed seven NMs using the two proxy measures: production volume and short-term aquatic ecotoxicity. It was found that Ag NMs ended up in the high ecotoxicity-low production volume quadrant; graphene, TiO2 and SiO2 NMs ended up in the low ecotoxicity-high production volume quadrant; CeO2, ZnO, and CNT ended up in the low ecotoxicity-low production volume. There are no NMs (mentioned above) located in the high ecotoxicity-low production volume quadrant, suggesting that none of NMs are of high environmental concern currently.
Sewage sludges also contained NMs, with much higher concentrations than those in the environmental compartments (Tou et al., Citation2017). The application of sewage sludges in agricultural soils could pose NM-related risk. Schlich et al. (Citation2013) assessed the risk of Ag NMs in sewage sludge-treated soil, and found that the PNEC (0.05 mg/kg soil) would be exceeded by applying Ag NM-containing sewage sludge in soil for 50 years. In another study, long-term (20 years) field applications of sewage sludge to soil resulted in an increase of Ag content to 51 mg/kg. Ag contents in the vegetable crops (red beet, carrot, and sugar beet) were generally lower than 0.7 mg/kg after soil-plant transfer when grown in sludge-applied soil, much lower than the safety threshold for the use by animals and humans, suggesting that environmental risk of Ag transferred to food chain is low (Wang et al., Citation2018). Although low risk to food chain, Ag (51 mg/kg) in the sewage sludge-treated soils may still have adverse effect to soil organisms (especially soil bacteria) according to the PNEC value of Ag NMs (0.05 mg/kg soil) (Schlich et al., Citation2013). No report on the risk assessment of other NMs was found in sewage sludge-treated soils.
3.2. Toxicity of NMs at ERCs
For risk assessment of NMs, both PECs and PNECs are modeled/calculated based on many assumptions which often lack of direct evidence for the toxicological information at ERCs. Currently, the research on the toxicity of NMs is still increasing, with more than 30,000 articles in the past two decades (). However, we only found around 30 articles claiming that their toxicological investigations toward TiO2, Ag, CuO, and graphene-based NMs are at ERCs (). The following discussion in this section is based on these toxicological investigations, and the ERCs used for nanotoxicological studies are shown in . The ERCs of TiO2, Ag, CuO, and graphene-based NMs used for toxicological investigations () are within or slightly higher the ranges of PECs and MECs ( and ). Most of these investigations focused on aquatic environments, followed by wastewater, soils, and sediments. All the four NMs were investigated at ERCs. For graphene-based NMs, only one report was found, in which GO at 1–100 μg/L was exposed to zebrafish embryos (Zhang et al., Citation2017).
Figure 5. The number of published papers on the toxicity of NMs from 2000 to 2019 (a), and current toxicological investigations that considered environmentally relevant concentrations of NMs (b). Data in panel (a) were collected from “Web of Science.” The environmental concentration data in panel (b) were collected from all the available publications ().
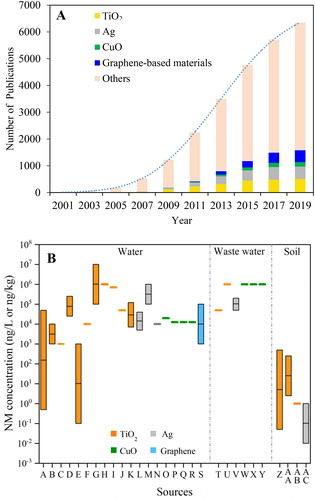
Table 1. Current investigations on the impact of nanomaterials (NMs) at environmentally relevant concentrations.
In surface water, TiO2 NMs at ERCs (700 μg/L) had no significant effect on the structure and distribution of microbial communities, and the eukaryotic communities were not changed either (Londono et al., Citation2017). In another report, TiO2 NMs at 250 μg/L were introduced into the mesocosms, and the phytoplankton were unaffected (Jovanović et al., Citation2016). For other aquatic organisms such as crustaceans (Daphnia magna) (Fouqueray et al., Citation2012), juvenile rainbow trout (Oncorhynchus mykiss) (Boyle et al., Citation2013), marine scallop (Azumapecten farreri) (Xia et al., Citation2017), and blood clam (Tegillarca granosa) (Guan et al., Citation2018), no mortality was observed during TiO2 NM exposure at ERCs. But other biological stresses such as oxidative damage, neurotoxicity, and/or decrease in growth and reproduction were observed during exposure. Ag NMs in aquatic phase caused the growth inhibition on and stress responses to bacteria (Khan et al., Citation2015; Wilke et al., Citation2016). Although Ag NMs at ERCs induced the up- and down-regulation of specific genes, the analyzed gene transcription profiles were unchanged in the gills of mussels (Mytilus galloprovincialis) after 15-day exposure (Bebianno et al., Citation2015). For GO, it is reported that the development of zebrafish embryos was impaired due to DNA modification, protein carbonylation and oxidative stress at its ERC (1–100 μg/L) (Zhang et al., Citation2017). In wastewater, the changes in bacterial community composition and abundances were observed after exposure to TiO2, Ag, or CuO NMs (Huang, Cao, et al., Citation2019; Londono et al., Citation2019; Miao et al., Citation2019; Zhang et al., Citation2018). The treatment performance of constructed wetlands, and the nitrogen removal efficiency in the wastewater reactor were thus decreased. However, no significant effect on algal cell density, chlorophyll-a, or periphyton biomass was observed after TiO2 NM exposure (Wright et al., Citation2018).
In soil compartment, TiO2 NMs at ERCs exhibited insignificant effect on bacterial abundance or C-mineralization potential activity (Simonin et al., Citation2015), while showed negative impact on the nitrification process in soil (Simonin et al., Citation2017). After Ag NM exposure, actino- and alpha-Proteobacteria in soil were unaffected after 1-year exposure, but the abundances of Acidobacteria, Bacteroidetes and beta-Proteobacteria were significantly decreased after exposure. The nitrification, organic carbon transformation and chitin degradation in soil may be decreased by Ag NMs (Grün & Emmerling, Citation2018). It should be noted that the ERCs (“mg/kg” level) of NMs used in soil compartments were higher than their PECs or MECs. The actual negative effect of NMs on soil microbial communities may be not obvious. The microbial communities in sewage sludge-treated soil are likely easier to be affected by NMs, which should receive more attention. Currently, related information in sediment compartment is scarce. Savić-Zdravković et al. (Citation2018) reported that TiO2 NMs at ERCs did not affect the mortality or emergence ratio of freshwater midge (Chironomus tentans), but morphological changes (e.g., mentum teeth, mandibles) in larvae were observed. Welz et al. (Citation2018) found that low content of Ag NMs (4 μg/kg) could modify the bacterial community structure in sediments. Environmental concentrations of NMs in sediments were much higher than soil, the effect of NMs at ERCs in sediments thus deserves further study.
4. Toxicity of NMs as affected by environmental factors
4.1. Nanotoxicity mechanisms
Nanotoxicity mechanism is a main emphasis for nanotoxicological investigations, which could be divided into three stages: external toxicity, interface interaction and intracellular response (). At “external toxicity” stage, NMs could cause both direct and indirect toxicity to cells/organisms (). The direct toxicity of NMs can be triggered at external surface. The physical contact could lead to membrane damage, and some of NMs are able to be taken up by cells during interface interaction (e.g., endocytosis) with cell membrane. Indirect toxicity is also important at the “external toxicity” stage, including shading effect, extracellular ROS generation, nutrient depletion and ionic toxicity (). Shading effect by graphene-based NMs was reported to cause indirect toxicity to both algae and protozoan (Hu et al., Citation2015; Zhao et al., Citation2017). Extracellular ROS generation could be facilitated by the photo-active NMs (e.g., TiO2). NMs with large surface area are able to adsorb nutrients and cause nutrient depletion to both algae and human cells (Creighton et al., Citation2013; Zhao et al., Citation2017). Ionic toxicity is originated from the dissolution of NMs (e.g., Ag, CuO), which is one of the most important toxicity mechanisms. After cellular internalization, the intracellular responses are activated, which are extensively investigated in the past two decades. At cellular levels, the internalized NMs could lead to oxidative stress, mitochondrial dysfunction, and DNA damage. Moreover, intracellular NMs could be further dissolved and/or biodegraded, which also contributes to the above responses and toxicity.
Figure 6. Schematic illustration of the mechanisms for NMs-induced toxicity and related processes as regulated by environmental factors. The four stages include environmental processes, external toxicity, interface interaction, and intracellular responses.
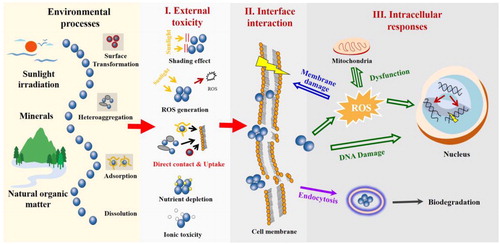
It should be noted that all these toxicity mechanisms (I–III, ) and the obtained toxicological data are highly influenced by environmental conditions. Environmental factors such as sunlight irradiation, natural organic matter (NOM), and minerals are able to change the toxicity of NMs through regulating the key processes of NMs. The key NM processes include surface transformation, heteroaggregation, adsorption and dissolution (), which are closely related to the external toxicity processes of NMs. How are these environmental conditions specifically regulating the processes and toxicity mechanisms of NMs? What are the changes of nanotoxicity under natural conditions? These questions are further discussed in the following sections.
4.2. Sunlight irradiation
TiO2, Ag, CuO and graphene NMs are all photo-active (Markiewicz et al., Citation2018; Mitrano et al., Citation2015). Among them, TiO2 is the most prominent example for photoactive NMs. Due to high photocatalytic activity, TiO2 NMs could result in photo-toxicity to bacteria (e.g., Escherichia coli and Aeromonas hydrophila), which was highly related to the production of ROS (e.g., OH·) (Tong et al., Citation2013). It is noted that anatase is more toxic than rutile TiO2 NMs under light irradiation due to higher photocatalytic activity. Ma et al. (Citation2012) found that TiO2 NMs under simulated sunlight irradiation exhibited much higher toxicity than that under ambient laboratory light toward D. magna and Japanese medaka. However, the photoactivity of TiO2 NMs was not obvious when interacting with some organisms such as algae and plants. For example, no enhanced toxicity to green algae (Raphidocelis subcapitata) was observed during exposure to TiO2 NMs under visible, UVA, and UVB irradiation (Lee & An, Citation2013). TiO2 NMs were reported to facilitate the photosynthesis, stomatal conductance and growth of plants in some cases (Hu et al., Citation2020; Liu et al., Citation2019; Tan et al., Citation2018).
Different from TiO2, Ag NMs are readily to undergo physical and chemical transformation. Cheng et al. (Citation2011) reported that sunlight irreversibly decreased the dispersion stability of Ag NMs, and the toxicity to a wetland plant (Lolium multiflorum) was highly suppressed. When releasing into natural environments, Ag NMs would not be the sole species. During oxidation and dissolution, Ag+ ions are releasing, and the more stable forms such as AgCl and Ag2S could be formed, which are the persistent forms in natural environments (Li et al., Citation2017). It is reported that the dissolution of Ag NMs was unchanged by sunlight irradiation, but altered in the presence of Cl− ions. The phototoxicity of Ag NMs toward E. coli was decreased by Cl− at low concentrations (e.g., 0.01 M, 0.1 M), which was highly related to the dissolved Ag+ ions (Li et al., Citation2018). Sulfidation of Ag NMs also reduced the toxicity to zebrafish (Devi et al., Citation2015). In addition, Ag+ ions could be formed to Ag NMs under sunlight irradiation with the assistance of different organic matters such as dissolved organic matter and extracellular polymeric substances (EPS) from bacteria and algae (Kathiraven et al., Citation2015; Yin et al., Citation2012; Zhang et al., Citation2016). Even GO could facilitate the formation of Ag NMs from Ag+ ions (Cao et al., Citation2019). Sunlight can accelerate the transfer of electrons from the EPS to adjacent Ag+, and/or excite organic matters to produce strong reducing species for the photoreduction of Ag+ ions, thus causing the formation of Ag NMs (Zhang et al., Citation2016). This photoreduction process could decrease the toxicity and risk of Ag+ ions in natural environments because Ag NMs are less toxic in most scenarios (Choi et al., Citation2018).
Carbon NMs such as graphene and CNTs could undergo photo-transformation due to their conjugated bond system (Markiewicz et al., Citation2018). This photo-transformation is mainly surface oxidation, but the surface change is minor because of the relative stable graphitic structures. However, GO is very photoactive, and the photoreduction of GO surface has been reported (Han et al., Citation2019; Matsumoto et al., Citation2011). For bacteria, the photo-reduction increased the toxicity of GO to E. coli (Hou et al., Citation2017). In another work, the antibacterial activity of GO was increased after short-time (1–3 h) UV irradiation, and increased after long-time irradiation (12–60 h) (Gao et al., Citation2019). For algae, the photo-transformation of GO also enhanced the toxicity, mainly due to the production of dissolved organic byproducts (low molecular weight species) (Zhao et al., Citation2020). However, there are no toxicological data on the long-term photo-transformation of GO. Although GO could be well-dispersed in waters, long-term photoreduction is expected to lower the suspension stability of GO due to enhanced hydrophobicity. Therefore, the toxicity to aquatic organisms is likely to be lower, and more attention should be paid on benthonic organisms. CuO NMs are not very photo-active. The role of sunlight irradiation in the transformation (e.g., dissolution, sulfidation) of CuO NMs is unknown. Possible effect of sunlight irradiation on CuO NM toxicity needs further investigation.
4.3. Natural organic matter
NOM is a key component in natural waters to alter the fate and toxicity of NMs, with the concentrations ranging at 1–100 mg/L in surface water, soil water, and groundwater (Sharma et al., Citation2019). All the environmental processes (surface transformation, aggregation, adsorption, dissolution) of NMs as shown in could be impacted by NOM through NMs-NOM interaction. There are already many studies focusing on the toxicity of NMs as altered by NOM. NOM could change nanotoxicity through regulating (I) suspension stability of NMs, (II) electrostatic repulsion between NMs and organisms/cells, (III) steric hindrance, (IV) generation of ROS, and (V) bioavailability of dissolved metal ions (Wang et al., Citation2016). For most scenarios, NOM could decrease the toxicity of NMs to organisms in both aquatic and soil environments. For TiO2 and graphene-based NMs, they do not dissolve much in water. Therefore, NOM could mitigate their toxicity through Mechanisms I-IV. For example, humic acid (HA) reduced the toxicity of TiO2 NMs to alga (Chlorella sp.) through preventing the adhesion to algae due to electrostatic and steric repulsions (Lin et al., Citation2012). HA could also mitigate the toxicity of GO to both alga and D. magna due to the alleviation of oxidative damage and steric hindrance (Zhang et al., Citation2019; Zhao et al., Citation2019). For Ag and CuO NMs, Mechanism V (bioavailability of dissolved metal ions) is also important for the nanotoxicity mitigation in addition to Mechanisms I-IV. NOM in aqueous systems could reduce the bioavailable metal ions for organisms through (1) decreasing NM dissolution (Gunsolus et al., Citation2015), and/or (2) the formation of complexes of NOM-metal ions (Seitz et al., Citation2015). In addition, soil NOM could also increase Ag retention by the soil solid particles and decrease dissolved Ag levels (Li et al., Citation2017).
There are a few investigations which show the nanotoxicity enhancement in the presence of NOM. Castro et al. (Citation2018) investigated the toxicity of GO to nine organisms including green algae (Raphidocelis subcapitata), aquatic plant (Lemna minor), lettuce (Lactuca sativa), planktonic microcrustacean (D. magna), brine shrimp (Artemia salina), chironomidae (Chironomus sancticaroli), freshwater polyp (Hydra attenuata), and nematodes (Caenorhabditis elegans). HA only increased the toxicity of GO to D. magna and C. elegans, and the reason for the observed toxicity enhancement was unclear. The increased toxicity of TiO2 NMs to zebrafish (Danio rerio) by HA was also reported (Yang et al., Citation2013) due to increased oxidative stress. HA was coated on the surface of TiO2 NMs and increased suspension stability, however, this was not responsible for the increased toxicity due to decreased TiO2 NM uptake. On the contrary, NOM increased the accumulation of graphene sheets in the bodies of zebrafish, especially for gut and gill (Lu et al., Citation2017), but the role of NOM in the biological responses was unknown. All these unclear points need to be further investigated to better understand the nanotoxicity enhancement mechanisms induced by NOM.
It is noted that toxicity of NMs could be influenced by NOM types. It is reported that bovine serum albumin (BSA) increased the toxicity of NMs (e.g., Ag) by increasing their accumulation and dispersion. HA reduced the toxicity of most of NMs by scavenging free radicals and reducing the accumulation of NMs. These findings highlighted the significant influence of the NOM types on the toxicity of NMs in aquatic environments (Liang et al., Citation2020). Sunlight irradiation is another factor that may influence nanotoxicity. NOM molecules such as HA and citric acid serve as free radical scavengers to mitigate the toxicity of NMs (e.g., Ag, CuO, CeO2) (Gao et al., Citation2012; Peng et al., Citation2015; Tella et al., Citation2014). However, under sunlight irradiation, HA could be as a photosensitizer or precursor for ROS production (Dasari & Hwang, Citation2010), which may be the reason for the stronger TiO2 NM toxicity in the presence of HA under sunlight irradiation than that without irradiation (Yang et al., Citation2013). Moreover, sunlight irradiation is able to degrade NOM (Yang et al., Citation2013), and the NOM-induced nanotoxicity may be ultimately reduced if the NOM-coated NMs are thoroughly exposed to long-term sunlight irradiation.
4.4. Minerals
Heteroaggregation is a dominant driving factor for NM settling from the water columns (Espinasse et al., Citation2018). Minerals are important components of soils and sediments, and are widely present in natural waters (Zhao et al., Citation2015). NMs in these environmental compartments can heteroaggregate with mineral particles. Heteroaggregation could occur between minerals and all the four types of NMs (TiO2, Ag, CuO, graphene). GO sheets were able to heteroaggregate with positively charged goethite and Al2O3 particles, while did not with negatively charged montmorillonite, kaolinite and SiO2 particles, confirming that the above heteroaggregation was dominated by electrostatic interaction (Zhao et al., Citation2015). Similar heteroaggregation was found between GO and hematite (Feng et al., Citation2017). Interestingly, negatively charged Ag NMs could heteroaggregate with negatively charged montmorillonite through the face-edge conformation (Zhou et al., Citation2012). This is because that the edge of montmorillonite remained positively charged when the solution pH was higher than its zero point of charge (ZPC). All these heteroaggregation processes could destabilize NMs in aquatic environments. Due to heteroaggregation, the toxicity of NMs in the presence of minerals was highly decreased for all the scenarios. It is reported that GO toxicity to protozoan was reduced by the formation of GO-kaolin heteroaggregates (Kryuchkova & Fakhrullin, Citation2018). Heteroaggregation with hematite decreased antimicrobial activity of Ag NMs by physically preventing the direct contact with bacteria (E. coli) (Huynh et al., Citation2014). GO toxicity to alga (Auxenochlorella pyrenoidosa) was reduced by Al2O3 particles with different shapes due to the formation of GO-Al2O3 heteroaggregates and the decrease in direct contact and membrane damage (Zhao et al., Citation2018). NOM further decreased the toxicity of GO in the presence of Al2O3 particles due to the surface coating of GO-Al2O3 heteroaggregates by NOM and the enhanced steric hindrance (Zhao et al., Citation2018). In addition to heteroaggregation, the reduction in bioavailable metal ions released from NMs (e.g., Ag, CuO) was another mechanism for the NM toxicity mitigation. For example, hematite reduced Ag NM toxicity to another alga Chlamydomonas reinhardtii by decreasing the bioavailability of Ag ions through adsorption (Huang, Wei, et al., Citation2019).
Different types of NMs are co-existing in environmental compartments. Antagonistic effect was observed during the co-exposure of different NMs, which could also be explained by the above two mechanisms (heteroaggregation and reduction in bioavailable metal ions). For example, combined mixtures of different NMs (e.g., CuO, TiO2, Fe2O3) were significantly less toxic in comparison to individual NMs due to increased heteroaggregation (Jośko et al., Citation2017). The antagonistic effect of negatively charged GO and ZnO NMs to freshwater fish larva (Danio rerio) was also observed in the incubation systems, which was mainly due to the adsorption of the released Zn2+ ions by GO (Ye et al., Citation2018). This mechanism was also responsible for the reduced toxicity of TiO2 and ZnO NMs to bacteria (Tong et al., Citation2015). The combined toxicity of NMs may also influenced by sunlight irradiation. Under dark condition, TiO2 NMs were reported to attenuate Ag NM toxicity due to the adsorption of dissolved Ag+ (Wilke et al., Citation2016). However, under sunlight irradiation, TiO2 and Ag NMs showed synergistic toxicity due to enhanced oxidative stress (Wilke et al., Citation2018).
4.5. Aging
NMs undergo aging after releasing into the compartments. The aging processes are the combined effect of sunlight irradiation, NOM, minerals, as well as biomolecules (), which include chemical transformation, adsorption, and heteroaggregation. Adsorption of NOM and heteroaggregation of minerals could reduce NM toxicity, which has been discussed above. For chemical transformation, sulfidation of NMs (e.g., CuO, Ag) was a main process in water, sediments, and soil compartments. For CuO NMs, sulfidation is an important transformation in biological and environmental systems, which was reported to reduce the toxicity of CuO NMs to human cells (Wang et al., Citation2013). For Ag NMs, sulfidation reduced the mortality and reproduction of C. elegans caused by Ag NMs. This is because sulfidation not only decreased dissolution of Ag NMs, but also reduced the bioavailability of intact Ag NMs (Starnes et al., Citation2015). AgCl is another transformed product in the environment. Cl− could decrease Ag NM toxicity to bacteria by forming AgCl and decreasing bioavailable Ag+ ions in aquatic phase (Chambers et al., Citation2014). The chemical transformation processes are further regulated by NOM. It is reported that Ag NMs were transformed to Ag2S (main species), AgCl, Ag3PO4, and Ag2O in the flooded soil after incubation for 28 days, and soil NOM further increased transformation and retention of Ag NMs in soils (Li et al., Citation2017). In another work, aging by NOM (fulvic acid, FA) was found to reduce the toxicity of pristine and sulfurized Ag NMs to soil bacteria in media with organic matter (FA) (Schultz et al., Citation2018). All these aging processes under laboratory conditions suggest that toxicity of NMs is likely to be reduced in natural environments.
The microcosm and mesocosm approaches could be more realistic to reveal the ecotoxicity of NMs in terrestrial and aquatic ecosystems (Jovanović et al., Citation2016; Lu et al., Citation2020; Vijayaraj et al., Citation2018). A mesocosm experiment (5 weeks) was conducted to investigate the toxicity of TiO2 NMs to periphytic algae. TiO2 NMs at 0.05 mg/L (ECRs) did not show significant changes on growth and algal assemblage composition, suggesting that TiO2 NMs had low environmental risk in natural waters (Wright et al., Citation2018). However, TiO2 NMs at 5 mg/L (concentration in wastewater) decreased periphyton biomass and altered algal assemblage structure, indicating that the toxicity of TiO2 NMs in wastewater should be paid more attention. In another mesocosm study, TiO2 NMs at 0.025 and 0.25 mg/L reduced the biomass of Rotifera, however, the growth of Cladocera, Copepoda, phytoplankton, macrophytes, chironomids and fish was unaffected (Jovanović et al., Citation2016). Therefore, TiO2 NMs at ECRs would not significantly affect the main functions of freshwater ecosystem. For CuO NMs (12.5 µg/L) in a mesocosm, no mortality was observed for the endobenthic species (Scrobicularia plana and Hediste diversicolor) after exposure for 21 days (Buffet et al., Citation2013), although some biochemical parameters such as behavioral biomarkers, antioxidant defenses and genotoxicity were changed during exposure.
5. Concluding remarks, challenges, and future research perspectives
Environmental risk assessment of NMs requires abundant and accurate data on their environmental concentrations and ecotoxicity. For environmental concentrations, the collected PEC and MEC data in this review show that the concentrations of the four types of NMs (TiO2, Ag, CuO, graphene) are very low in surface water and soil, while more NMs are deposited in sediments. Wastewater effluents and sewage sludges contain relatively high concentrations of NMs, and the environmental concentrations for the surface waters near the WWTPs and sewage sludge-treated soils are therefore at high levels. Currently, the ecotoxicity of NMs in surface water and soil compartments are of low concern as indicated by much higher PNECs than PECs. In wastewater, NMs showed higher toxicity than natural surface water, which could affect the treatment performance of WWTP. The toxicity of NMs at ERCs demonstrated that individual bacteria and microbial communities are very sensitive to NM exposure. For other organisms, no or low mortality was observed, instead, the biological responses such as antioxidative defense and gene expression could be triggered under NM exposure at ERCs.
It should be noted that the NM-induced toxicity under laboratory conditions could be changed by environmental factors including sunlight irradiation, NOM, and minerals. Generally, sunlight irradiation enhanced the toxicity of TiO2 and GO among the four types of NMs. NOM reduced the toxicity of NMs for most scenarios, in which the electrostatic and steric hindrance between NMs and organisms/cells induced by surface coating could be the main mechanisms. For minerals, toxicity of all the four types of NMs was mitigated for all the scenarios due to heteroaggregation, and the adsorption of released metal ions (for Ag and CuO) on minerals. The aging (e.g., sulfidation) of NMs in the environment generally reduced nanotoxicity. Taken together, the toxicity of NMs could be reduced under real environmental conditions, and the obtained toxic parameters under laboratory conditions may likely be overestimated.
Therefore, it can be generally concluded that the environmental risks of the four NMs are of low concern in surface water and soil compartments presently. More concerns should be raised on nanotoxicity in sediments, and any environmental compartments contaminated by wastewater, sewage sludges, and/or accidental spills. However, there are still many uncertainties/challenges on both environmental concentrations and ecotoxicity, which are identified as follows:
For the modeling of PECs, there are several fate models (e.g., materials flow analysis model, steady-state fate and transport model, dynamic stochastic model, nanoFate model) for predicting the environmental distribution of NMs (Garner et al., Citation2017; Giese et al., Citation2018; Musee, Citation2018). Each model has respective assumptions and limitations. Although the environmental processes of NMs such as dissolution, transformation and aggregation were considered in some fate models, there are many assumptions and uncertainties, which require the confirmation by experimental investigations. For examples, the production volume of NMs in the world or specific countries is largely uncertain, while it is crucial for the outcome of exposure modeling (Giese et al., Citation2018); the homoaggregation of NMs was commonly assumed to negligible (Garner et al., Citation2017); NMs in environments could be taken up by organisms, but this factor was not be included in the fate description during modeling. More efforts are needed for more accurate predictions.
For MECs, the measurement techniques such as sp-ICP-MS cannot distinguish a type of NMs with different forms (e.g., different surface coatings, crystal forms). It is also hard to distinguish engineered NMs from naturally occurring nanoparticles in environmental samples (Sun et al., Citation2014). In addition, the quantification of metal-based NMs by sp-ICP-MS is based on the assumptions: (1) all the particles are spherical; and (2) the molecular formula, and chemical compositions are already known (Tou et al., Citation2017). Moreover, the quantification technology of carbon-based NMs still has many limitations. The development of new analytical methods should be specific for very low concentrations of targeted NMs in different environmental compartments.
For ecotoxicity, most of the mechanistic investigations on nanotoxicity are based on the toxicological experiments at high concentrations. Therefore, the provided mechanistic insights are likely not useful for the risk assessments at ECRs (Krug, Citation2014). In addition, heteroaggregation and aging processes in aqueous environments could induce the settling of NMs into sediments. This process brings further risks to benthonic organisms, which deserves future and focused studies. Moreover, more efforts should be made on the compartments near the NM “point sources” (e.g., production plant outfalls and waste treatment plants). More nanotoxicity studies on field and outdoor mesocosms are also needed.
One of the main aims of environmental risk assessment of NMs is to better guide the application of nanotechnology. The environmental concentrations and PNECs of NMs summarized in this review may provide useful information on the selection of suitable and safe NM types and concentrations during nanotechnology applications (e.g., nano-agriculture; nano-remediation) in environments. In addition, the interfacial interactions between NMs and cells are helpful for designing the NMs with controlled shape, size and surface properties to minimize their toxicity and environment-related issues (Amde et al., Citation2017). For example, the safer NMs could be designed by coating with NOM and forming biomolecule coronas (Markiewicz et al., Citation2018).
Supplemental Material
Download MS Word (93.7 KB)Additional information
Funding
References
- Amde, M., Liu, J. F., Tan, Z. Q., & Bekana, D. (2017). Transformation and bioavailability of metal oxide nanoparticles in aquatic and terrestrial environments. A review. Environmental Pollution, 230, 250–267. doi: 10.1016/j.envpol.2017.06.064
- Arvidsson, R. (2018). Risk assessments show engineered nanomaterials to be of low environmental concern. Environmental Science & Technology, 52(5), 2436–2437. doi: 10.1021/acs.est.8b00754
- Arvidsson, R., Baun, A., Furberg, A., Hansen, S. F., & Molander, S. (2018). Proxy measures for simplified environmental assessment of manufactured nanomaterials. Environmental Science & Technology, 52(23), 13670–13680. doi: 10.1021/acs.est.8b05405
- Auffan, M., Rose, J., Bottero, J. Y., Lowry, G. V., Jolivet, J. P., & Wiesner, M. R. (2009). Towards a definition of inorganic nanoparticles from an environmental, health and safety perspective. Nature Nanotechnology, 4(10), 634–641. doi: 10.1038/nnano.2009.242
- Bebianno, M. J., Gonzalez-Rey, M., Gomes, T., Mattos, J. J., Flores-Nunes, F., & Bainy, A. C. D. (2015). Is gene transcription in mussel gills altered after exposure to Ag nanoparticles? Environmental Science and Pollution Research, 22(22), 17425–17433. doi: 10.1007/s11356-015-5186-z
- Bour, A., Mouchet, F., Silvestre, J., Gauthier, L., & Pinelli, E. (2015). Environmentally relevant approaches to assess nanoparticles ecotoxicity: A review. Journal of Hazardous Materials, 283, 764–777. doi: 10.1016/j.jhazmat.2014.10.021
- Bourgeault, A., Cousin, C., Geertsen, V., Cassier-Chauvat, C., Chauvat, F., Durupthy, O., Chanéac, C., & Spalla, O. (2015). The challenge of studying TiO2 nanoparticle bioaccumulation at environmental concentrations: Crucial use of a stable isotope tracer. Environmental Science & Technology, 49(4), 2451–2459. doi: 10.1021/es504638f
- Boyle, D., Al-Bairuty, G. A., Henry, T. B., & Handy, R. D. (2013). Critical comparison of intravenous injection of TiO2 nanoparticles with waterborne and dietary exposures concludes minimal environmentally-relevant toxicity in juvenile rainbow trout Oncorhynchus mykiss. Environmental Pollution, 182, 70–79. doi: 10.1016/j.envpol.2013.07.001
- Buchman, J. T., Hudson-Smith, N. V., Landy, K. M., & Haynes, C. L. (2019). Understanding nanoparticle toxicity mechanisms to inform redesign strategies to reduce environmental impact. Accounts of Chemical Research, 52(6), 1632–1642. doi: 10.1021/acs.accounts.9b00053
- Buffet, P. E., Richard, M., Caupos, F., Vergnoux, A., Perrein-Ettajani, H., Luna-Acosta, A., Akcha, F., Amiard, J. C., Amiard-Triquet, C., Guibbolini, M., Risso-De Faverney, C., Thomas-Guyon, H., Reip, P., Dybowska, A., Berhanu, D., Valsami-Jones, E., & Mouneyrac, C. (2013). A mesocosm study of fate and effects of CuO nanoparticles on endobenthic species (Scrobicularia plana). Environmental Science & Technology, 47(3), 130110104824003–130110104821628. doi: 10.1021/es303513r
- Buffet, P.-E., Tankoua, O. F., Pan, J.-F., Berhanu, D., Herrenknecht, C., Poirier, L., Amiard-Triquet, C., Amiard, J.-C., Bérard, J.-B., Risso, C., Guibbolini, M., Roméo, M., Reip, P., Valsami-Jones, E., & Mouneyrac, C. (2011). Behavioural and biochemical responses of two marine invertebrates Scrobicularia plana and Hediste diversicolor to copper oxide nanoparticles. Chemosphere, 84(1), 166–174. doi: 10.1016/j.chemosphere.2011.02.003
- Cao, X., Ma, C., Zhao, J., Guo, H., Dai, Y., Wang, Z., & Xing, B. (2019). Graphene oxide mediated reduction of silver ions to silver nanoparticles under environmentally relevant conditions: Kinetics and mechanisms. Science of the Total Environment, 679, 270–278. doi: 10.1016/j.scitotenv.2019.05.034
- Castro, V. L., Clemente, Z., Jonsson, C., Silva, M., Vallim, J. H., de Medeiros, A. M. Z., & Martinez, D. S. T. (2018). Nanoecotoxicity assessment of graphene oxide and its relationship with humic acid. Environmental Toxicology and Chemistry, 37(7), 1998–2012. doi: 10.1002/etc.4145
- Chambers, B. A., Afrooz, A. N., Bae, S., Aich, N., Katz, L., Saleh, N. B., & Kirisits, M. J. (2014). Effects of chloride and ionic strength on physical morphology, dissolution, and bacterial toxicity of silver nanoparticles. Environmental Science & Technology, 48(1), 761–769. doi: 10.1021/es403969x
- Chen, G., Peijnenburg, W. J., Xiao, Y., & Vijver, M. G. (2018). Developing species sensitivity distributions for metallic nanomaterials considering the characteristics of nanomaterials, experimental conditions, and different types of endpoints. Food and Chemical Toxicology, 112, 563–570. doi: 10.1016/j.fct.2017.04.003
- Cheng, Y., Yin, L., Lin, S., Wiesner, M., Bernhardt, E., & Liu, J. (2011). Toxicity reduction of polymer-stabilized silver nanoparticles by sunlight. The Journal of Physical Chemistry C, 115(11), 4425–4432. doi: 10.1021/jp109789j
- Choi, Y., Kim, H. A., Kim, K. W., & Lee, B. T. (2018). Comparative toxicity of silver nanoparticles and silver ions to Escherichia coli. Journal of Environmental Sciences, 66, 50–60. doi: 10.1016/j.jes.2017.04.028
- Coll, C., Notter, D., Gottschalk, F., Sun, T., Som, C., & Nowack, B. (2016). Probabilistic environmental risk assessment of five nanomaterials (nano-TiO2, nano-Ag, nano-ZnO, CNT, and fullerenes). Nanotoxicology, 10(4), 436–444. doi: 10.3109/17435390.2015.1073812
- Creighton, M. A., Rangel-Mendez, J. R., Huang, J., Kane, A. B., & Hurt, R. H. (2013). Graphene‐induced adsorptive and optical artifacts during in vitro toxicology assays. Small, 9(11), 1921–1927. doi: 10.1002/smll.201202625
- Dasari, T. P., & Hwang, H. M. (2010). The effect of humic acids on the cytotoxicity of silver nanoparticles to a natural aquatic bacterial assemblage. Science of the Total Environment, 408(23), 5817–5823. doi: 10.1016/j.scitotenv.2010.08.030
- Devi, G. P., Ahmed, K. B. A., Varsha, M. S., Shrijha, B. S., Lal, K. S., Anbazhagan, V., & Thiagarajan, R. (2015). Sulfidation of silver nanoparticle reduces its toxicity in zebrafish. Aquatic Toxicology, 158, 149–156. doi: 10.1016/j.aquatox.2014.11.007
- Dong, S., Qu, M., Rui, Q., & Wang, D. (2018). Combinational effect of titanium dioxide nanoparticles and nanopolystyrene particles at environmentally relevant concentrations on nematode Caenorhabditis elegans. Ecotoxicology and Environmental Safety, 161, 444–450. doi: 10.1016/j.ecoenv.2018.06.021
- Espinasse, B. P., Geitner, N. K., Schierz, A., Therezien, M., Richardson, C. J., Lowry, G. V., Ferguson, L., & Wiesner, M. R. (2018). Comparative persistence of engineered nanoparticles in a complex aquatic ecosystem. Environmental Science & Technology, 52(7), 4072–4078. doi: 10.1021/acs.est.7b06142
- Feng, Y., Liu, X., Huynh, K. A., McCaffery, J. M., Mao, L., Gao, S., & Chen, K. L. (2017). Heteroaggregation of graphene oxide with nanometer-and micrometer-sized hematite colloids: Influence on nanohybrid aggregation and microparticle sedimentation. Environmental Science & Technology, 51(12), 6821–6828. doi: 10.1021/acs.est.7b00132
- Fouqueray, M., Dufils, B., Vollat, B., Chaurand, P., Botta, C., Abacci, K., Labille, J., Rose, J., & Garric, J. (2012). Effects of aged TiO2 nanomaterial from sunscreen on Daphnia magna exposed by dietary route. Environmental Pollution, 163, 55–61. doi: 10.1016/j.envpol.2011.11.035
- Gao, J., Powers, K., Wang, Y., Zhou, H., Roberts, S. M., Moudgil, B. M., Koopman, B., & Barber, D. S. (2012). Influence of Suwannee River humic acid on particle properties and toxicity of silver nanoparticles. Chemosphere, 89(1), 96–101. doi: 10.1016/j.chemosphere.2012.04.024
- Gao, Y., Ren, X., Zhang, X., & Chen, C. (2019). Environmental fate and risk of ultraviolet-and visible-light-transformed graphene oxide: A comparative study. Environmental Pollution, 251, 821–829. doi: 10.1016/j.envpol.2019.05.010
- Garner, K. L., Suh, S., & Keller, A. A. (2017). Assessing the risk of engineered nanomaterials in the environment: Development and application of the nanoFate model. Environmental Science & Technology, 51(10), 5541–5551. doi: 10.1021/acs.est.6b05279
- Giese, B., Klaessig, F., Park, B., Kaegi, R., Steinfeldt, M., Wigger, H., Gleich, A. v., & Gottschalk, F. (2018). Risks, release and concentrations of engineered nanomaterial in the environment. Scientific Reports, 8(1), 1–18. doi: 10.1038/s41598-018-19275-4
- Gomes, T., Pinheiro, J. P., Cancio, I., Pereira, C. G., Cardoso, C., & Bebianno, M. J. (2011). Effects of copper nanoparticles exposure in the mussel Mytilus galloprovincialis. Environmental Science & Technology, 45(21), 9356–9362. doi: 10.1021/es200955s
- Goodwin, D. G., Jr, Adeleye, A. S., Sung, L., Ho, K. T., Burgess, R. M., & Petersen, E. J. (2018). Detection and quantification of graphene-family nanomaterials in the environment. Environmental Science & Technology, 52(8), 4491–4513. doi: 10.1021/acs.est.7b04938
- Gottschalk, F., Lassen, C., Kjoelholt, J., Christensen, F., & Nowack, B. (2015). Modeling flows and concentrations of nine engineered nanomaterials in the Danish environment. International Journal of Environmental Research and Public Health, 12(5), 5581–5602. doi: 10.3390/ijerph120505581
- Gottschalk, F., Sonderer, T., Scholz, R. W., & Nowack, B. (2009). Modeled environmental concentrations of engineered nanomaterials (TiO2, ZnO, Ag, CNT, fullerenes) for different regions. Environmental Science & Technology, 43(24), 9216–9222. doi: 10.1021/es9015553
- Gottschalk, F., Sonderer, T., Scholz, R. W., & Nowack, B. (2010). Possibilities and limitations of modeling environmental exposure to engineered nanomaterials by probabilistic material flow analysis. Environmental Toxicology and Chemistry, 29(5), n/a–1048. doi: 10.1002/etc.135
- Grün, A. L., & Emmerling, C. (2018). Long-term effects of environmentally relevant concentrations of silver nanoparticles on major soil bacterial phyla of a loamy soil. Environmental Sciences Europe, 30(1), 31. doi: 10.1186/s12302-018-0160-2
- Guan, X., Shi, W., Zha, S., Rong, J., Su, W., & Liu, G. (2018). Neurotoxic impact of acute TiO2 nanoparticle exposure on a benthic marine bivalve mollusk, Tegillarca granosa. Aquatic Toxicology, 200, 241–246. doi: 10.1016/j.aquatox.2018.05.011
- Gunsolus, I. L., Mousavi, M. P. S., Hussein, K., Bühlmann, P., & Haynes, C. L. (2015). Effects of humic and fulvic acids on silver nanoparticle stability, dissolution, and toxicity. Environmental Science & Technology, 49(13), 8078–8086. doi: 10.1021/acs.est.5b01496
- Han, Y., Knightes, C. D., Bouchard, D., Zepp, R., Avant, B., Hsieh, H. S., Chang, X., Acrey, B., Henderson, W. M., & Spear, J. (2019). Simulating graphene oxide nanomaterial phototransformation and transport in surface water. Environmental Science: Nano, 6(1), 180–194. doi: 10.1039/C8EN01088A
- Holden, P. A., Klaessig, F., Turco, R. F., Priester, J. H., Rico, C. M., Avila-Arias, H., Mortimer, M., Pacpaco, K., & Gardea-Torresdey, J. L. (2014). Evaluation of exposure concentrations used in assessing manufactured nanomaterial environmental hazards: Are they relevant? Environmental Science & Technology, 48(18), 10541–10551. doi: 10.1021/es502440s
- Hoque, M. E., Khosravi, K., Newman, K., & Metcalfe, C. D. (2012). Detection and characterization of silver nanoparticles in aqueous matrices using asymmetric-flow field flow fractionation with inductively coupled plasma mass spectrometry. Journal of Chromatography A, 1233, 109–115. doi: 10.1016/j.chroma.2012.02.011
- Hou, W. C., Lee, P. L., Chou, Y. C., & Wang, Y. S. (2017). Antibacterial property of graphene oxide: The role of phototransformation. Environmental Science: Nano, 4(3), 647–657. doi: 10.1039/C6EN00427J
- Hu, C., Wang, Q., Zhao, H., Wang, L., Guo, S., & Li, X. (2015). Ecotoxicological effects of graphene oxide on the protozoan Euglena gracilis. Chemosphere, 128, 184–190. doi: 10.1016/j.chemosphere.2015.01.040
- Hu, J., Wu, X., Wu, F., Chen, W., White, J. C., Yang, Y., Wang, B., Xing, B., Tao, S., & Wang, X. (2020). Potential application of titanium dioxide nanoparticles to improve the nutritional quality of coriander (Coriandrum sativum L.). Journal of Hazardous Materials, 389, 121837. doi: 10.1016/j.jhazmat.2019.121837
- Huang, B., Wei, Z. B., Yang, L. Y., Pan, K., & Miao, A. J. (2019). Combined toxicity of silver nanoparticles with hematite or plastic nanoparticles toward two freshwater algae. Environmental Science & Technology, 53(7), 3871–3879. doi: 10.1021/acs.est.8b07001
- Huang, J., Cao, C., Liu, J., Yan, C., & Xiao, J. (2019). The response of nitrogen removal and related bacteria within constructed wetlands after long-term treating wastewater containing environmental concentrations of silver nanoparticles. Science of the Total Environment, 667, 522–531. doi: 10.1016/j.scitotenv.2019.02.396
- Huynh, K. A., McCaffery, J. M., & Chen, K. L. (2014). Heteroaggregation reduces antimicrobial activity of silver nanoparticles: Evidence for nanoparticle–cell proximity effects. Environmental Science & Technology Letters, 1(9), 361–366. doi: 10.1021/ez5002177
- Jośko, I., Oleszczuk, P., & Skwarek, E. (2017). Toxicity of combined mixtures of nanoparticles to plants. Journal of Hazardous Materials, 331, 200–209. doi: 10.1016/j.jhazmat.2017.02.028
- Jovanović, B., Bezirci, G., Çağan, A. S., Coppens, J., Levi, E. E., Oluz, Z., Tuncel, E., Duran, H., & Beklioğlu, M. (2016). Food web effects of titanium dioxide nanoparticles in an outdoor freshwater mesocosm experiment. Nanotoxicology, 10(7), 902–912. doi: 10.3109/17435390.2016.1140242
- Kägi, R., Ulrich, A., Sinnet, B., Vonbank, R., Wichser, A., Zuleeg, S., Simmler, H., Brunner, S., Vonmont, H., Burkhardt, M., & Boller, M. (2008). Synthetic TiO2 nanoparticle emission from exterior facades into the aquatic environment. Environmental Pollution, 156(2), 233–239. doi: 10.1016/j.envpol.2008.08.004
- Kathiraven, T., Sundaramanickam, A., Shanmugam, N., & Balasubramanian, T. (2015). Green synthesis of silver nanoparticles using marine algae Caulerpa racemosa and their antibacterial activity against some human pathogens. Applied Nanoscience, 5(4), 499–504. doi: 10.1007/s13204-014-0341-2
- Khan, S. S., Ghouse, S. S., & Chandran, P. (2015). Toxic effect of environmentally relevant concentration of silver nanoparticles on environmentally beneficial bacterium Pseudomonas putida. Bioprocess and Biosystems Engineering, 38(7), 1243–1249. doi: 10.1007/s00449-015-1365-z
- Kjølholt, J., Gottschalk, F., Brinch, A., Lützhøft, H. C. H., Hartmann, N. B., Nowack, B., & Baun, A. (2015). Environmental assessment of nanomaterial use in Denmark: Final report, 15 September 2015.
- Krug, H. F. (2014). Nanosafety research—Are we on the right track? Angewandte Chemie International Edition, 53(46), n/a–12319. doi: 10.1002/anie.201403367
- Kryuchkova, M., & Fakhrullin, R. (2018). Kaolin alleviates graphene oxide toxicity. Environmental Science & Technology Letters, 5(5), 295–300. doi: 10.1021/acs.estlett.8b00135
- Lee, W. M., & An, Y. J. (2013). Effects of zinc oxide and titanium dioxide nanoparticles on green algae under visible, UVA, and UVB irradiations: No evidence of enhanced algal toxicity under UV pre-irradiation. Chemosphere, 91(4), 536–544. doi: 10.1016/j.chemosphere.2012.12.033
- Li, L., Stoiber, M., Wimmer, A., Xu, Z., Lindenblatt, C., Helmreich, B., & Schuster, M. (2016). To what extent can full-scale wastewater treatment plant effluent influence the occurrence of silver-based nanoparticles in surface waters? Environmental Science & Technology, 50(12), 6327–6333. doi: 10.1021/acs.est.6b00694
- Li, L., Wang, Q., Yang, Y., Luo, L., Ding, R., Yang, Z. G., & Li, H. P. (2019). Extraction method development for quantitative detection of silver nanoparticles in environmental soils and sediments by single particle inductively coupled plasma mass spectrometry. Analytical Chemistry, 91(15), 9442–9450. doi: 10.1021/acs.analchem.8b05575
- Li, M., Wang, P., Dang, F., & Zhou, D. M. (2017). The transformation and fate of silver nanoparticles in paddy soil: Effects of soil organic matter and redox conditions. Environmental Science: Nano, 4(4), 919–928. doi: 10.1039/C6EN00682E
- Li, Y., Wang, W., Wu, Q., Li, Y., Tang, M., Ye, B., & Wang, D. (2012). Molecular control of TiO2-NPs toxicity formation at predicted environmental relevant concentrations by Mn-SODs proteins. PLoS One, 7(9), e44688. doi: 10.1371/journal.pone.0044688
- Li, Y., Zhao, J., Shang, E., Xia, X., Niu, J., & Crittenden, J. (2018). Effects of chloride ions on dissolution, ROS generation, and toxicity of silver nanoparticles under UV irradiation. Environmental Science & Technology, 52(8), 4842–4849. doi: 10.1021/acs.est.7b04547
- Liang, D., Wang, X., Liu, S., Zhu, Y., Wang, Y., Fan, W., & Dong, Z. (2020). Factors determining the toxicity of engineered nanomaterials to Tetrahymena thermophila in freshwater: The critical role of organic matter. Environmental Science: Nano, 7(1), 304–316. doi: 10.1039/C9EN01017C
- Lin, D., Ji, J., Long, Z., Yang, K., & Wu, F. (2012). The influence of dissolved and surface-bound humic acid on the toxicity of TiO2 nanoparticles to Chlorella sp. Water Research, 46(14), 4477–4487. doi: 10.1016/j.watres.2012.05.035
- Liu, Y., Yue, L., Wang, Z., & Xing, B. (2019). Processes and mechanisms of photosynthesis augmented by engineered nanomaterials. Environmental Chemistry, 16(6), 430–445. doi: 10.1071/EN19046
- Londono, N., Donovan, A. R., Shi, H., Geisler, M., & Liang, Y. (2017). Impact of TiO2 and ZnO nanoparticles on an aquatic microbial community: Effect at environmentally relevant concentrations. Nanotoxicology, 11(9–10), 1140–1156. doi: 10.1080/17435390.2017.1401141
- Londono, N., Donovan, A. R., Shi, H., Geisler, M., & Liang, Y. (2019). Effects of environmentally relevant concentrations of mixtures of TiO2, ZnO and Ag ENPs on a river bacterial community. Chemosphere, 230, 567–577. doi: 10.1016/j.chemosphere.2019.05.110
- Loula, M., Kaňa, A., Koplík, R., Hanuš, J., Vosmanská, M., & Mestek, O. (2019). Analysis of silver nanoparticles using single-particle inductively coupled plasma–mass spectrometry (ICP-MS): Parameters affecting the quality of results. Analytical Letters, 52(2), 288–307. doi: 10.1080/00032719.2018.1459657
- Lu, K., Dong, S., Petersen, E. J., Niu, J., Chang, X., Wang, P., Lin, S., Gao, S., & Mao, L. (2017). Biological uptake, distribution, and depuration of radio-labeled graphene in adult zebrafish: Effects of graphene size and natural organic matter. ACS Nano, 11(3), 2872–2885. doi: 10.1021/acsnano.6b07982
- Lu, T., Qu, Q., Lavoie, M., Pan, X., Peijnenburg, W. J. G. M., Zhou, Z., Pan, X., Cai, Z., & Qian, H. (2020). Insights into the transcriptional responses of a microbial community to silver nanoparticles in a freshwater microcosm. Environmental Pollution, 258, 113727. doi: 10.1016/j.envpol.2019.113727
- Ma, H., Brennan, A., & Diamond, S. A. (2012). Phototoxicity of TiO2 nanoparticles under solar radiation to two aquatic species: Daphnia magna and Japanese medaka. Environmental Toxicology and Chemistry, 31(7), 1621–1629. doi: 10.1002/etc.1858
- Mahdi, K. N., Peters, R. J., Klumpp, E., Bohme, S., Van der Ploeg, M., Ritsema, C., & Geissen, V. (2017). Silver nanoparticles in soil: Aqueous extraction combined with single-particle ICP-MS for detection and characterization. Environmental Nanotechnology, Monitoring & Management, 7, 24–33. doi: 10.1016/j.enmm.2016.12.002
- Mahmoudi, M., Azadmanesh, K., Shokrgozar, M. A., Journeay, W. S., & Laurent, S. (2011). Effect of nanoparticles on the cell life cycle. Chemical Reviews, 111(5), 3407–3432. doi: 10.1021/cr1003166
- Markiewicz, M., Kumirska, J., Lynch, I., Matzke, M., Köser, J., Bemowsky, S., Docter, D., Stauber, R., Westmeier, D., & Stolte, S. (2018). Changing environments and biomolecule coronas: Consequences and challenges for the design of environmentally acceptable engineered nanoparticles. Green Chemistry, 20(18), 4133–4168. doi: 10.1039/C8GC01171K
- Matranga, V., & Corsi, I. (2012). Toxic effects of engineered nanoparticles in the marine environment: Model organisms and molecular approaches. Marine Environmental Research, 76, 32–40. doi: 10.1016/j.marenvres.2012.01.006
- Matsumoto, Y., Koinuma, M., Ida, S., Hayami, S., Taniguchi, T., Hatakeyama, K., Tateishi, H., Watanabe, Y., & Amano, S. (2011). Photoreaction of graphene oxide nanosheets in water. The Journal of Physical Chemistry C, 115(39), 19280–19286. doi: 10.1021/jp206348s
- Metcalfe, C. D., Sultana, T., Martin, J., Newman, K., Helm, P., Kleywegt, S., Shen, L., & Yargeau, V. (2018). Silver near municipal wastewater discharges into western Lake Ontario. Environmental Monitoring and Assessment, 190(9), 555. doi: 10.1007/s10661-018-6922-x
- Miao, L., Wang, C., Hou, J., Wang, P., Ao, Y., Li, Y., Yao, Y., Lv, B., Yang, Y., You, G., Xu, Y., & Gu, Q. (2017). Response of wastewater biofilm to CuO nanoparticle exposure in terms of extracellular polymeric substances and microbial community structure. Science of the Total Environment, 579, 588–597. doi: 10.1016/j.scitotenv.2016.11.056
- Miao, L., Wang, P., Hou, J., Yao, Y., Liu, Z., & Liu, S. (2019). Low concentrations of copper oxide nanoparticles alter microbial community structure and function of sediment biofilms. Science of the Total Environment, 653, 705–713. doi: 10.1016/j.scitotenv.2018.10.354
- Mitrano, D. M., Motellier, S., Clavaguera, S., & Nowack, B. (2015). Review of nanomaterial aging and transformations through the life cycle of nano-enhanced products. Environment International, 77, 132–147. doi: 10.1016/j.envint.2015.01.013
- Moore, M. N. (2006). Do nanoparticles present ecotoxicological risks for the health of the aquatic environment? Environment International, 32(8), 967–976. doi: 10.1016/j.envint.2006.06.014
- Mueller, N. C., & Nowack, B. (2008). Exposure modeling of engineered nanoparticles in the environment. Environmental Science & Technology, 42(12), 4447–4453. doi: 10.1021/es7029637
- Musee, N. (2018). Comment on “risk assessments show engineered nanomaterials to be of low environmental concern”. Environmental Science & Technology, 52(12), 6723–6724. doi: 10.1021/acs.est.8b02070
- Nel, A., Xia, T., Mädler, L., & Li, N. (2006). Toxic potential of materials at the nanolevel. Science, 311(5761), 622–627. doi: 10.1126/science.1114397
- Peng, C., Zhang, H., Fang, H., Xu, C., Huang, H., Wang, Y., Sun, L., Yuan, X., Chen, Y., & Shi, J. (2015). Natural organic matter–induced alleviation of the phytotoxicity to rice (Oryza sativa L.) caused by copper oxide nanoparticles. Environmental Toxicology and Chemistry, 34(9), 1996–2003. doi: 10.1002/etc.3016
- Peters, R. J., van Bemmel, G., Milani, N. B., den Hertog, G. C., Undas, A. K., van der Lee, M., & Bouwmeester, H. (2018). Detection of nanoparticles in Dutch surface waters. Science of the Total Environment, 621, 210–218. doi: 10.1016/j.scitotenv.2017.11.238
- Savić-Zdravković, D., Jovanović, B., Đurđević, A., Stojković-Piperac, M., Savić, A., Vidmar, J., & Milošević, D. (2018). An environmentally relevant concentration of titanium dioxide (TiO2) nanoparticles induces morphological changes in the mouthparts of Chironomus tentans. Chemosphere, 211, 489–499. doi: 10.1016/j.chemosphere.2018.07.139
- Schlich, K., Klawonn, T., Terytze, K., & Hund-Rinke, K. (2013). Hazard assessment of a silver nanoparticle in soil applied via sewage sludge. Environmental Sciences Europe, 25(1), 17. doi: 10.1186/2190-4715-25-17
- Schultz, C. L., Gray, J., Verweij, R. A., Busquets-Fité, M., Puntes, V., Svendsen, C., Lahive, E., & Matzke, M. (2018). Aging reduces the toxicity of pristine but not sulphidised silver nanoparticles to soil bacteria. Environmental Science: Nano, 5(11), 2618–2630. doi: 10.1039/C8EN00054A
- Seitz, F., Rosenfeldt, R. R., Storm, K., Metreveli, G., Schaumann, G. E., Schulz, R., & Bundschuh, M. (2015). Effects of silver nanoparticle properties, media pH and dissolved organic matter on toxicity to Daphnia magna. Ecotoxicology and Environmental Safety, 111, 263–270. doi: 10.1016/j.ecoenv.2014.09.031
- Sharma, V. K., Sayes, C. M., Guo, B., Pillai, S., Parsons, J. G., Wang, C., Yan, B., & Ma, X. (2019). Interactions between silver nanoparticles and other metal nanoparticles under environmentally relevant conditions: A review. Science of the Total Environment, 653, 1042–1051. doi: 10.1016/j.scitotenv.2018.10.411
- Simonin, M., Guyonnet, J. P., Martins, J. M., Ginot, M., & Richaume, A. (2015). Influence of soil properties on the toxicity of TiO2 nanoparticles on carbon mineralization and bacterial abundance. Journal of Hazardous Materials, 283, 529–535. doi: 10.1016/j.jhazmat.2014.10.004
- Simonin, M., Martins, J. M., Le Roux, X., Uzu, G., Calas, A., & Richaume, A. (2017). Toxicity of TiO2 nanoparticles on soil nitrification at environmentally relevant concentrations: Lack of classical dose–response relationships. Nanotoxicology, 11(2), 247–255. doi: 10.1080/17435390.2017.1290845
- Starnes, D. L., Unrine, J. M., Starnes, C. P., Collin, B. E., Oostveen, E. K., Ma, R., Lowry, G. V., Bertsch, P. M., & Tsyusko, O. V. (2015). Impact of sulfidation on the bioavailability and toxicity of silver nanoparticles to Caenorhabditis elegans. Environmental Pollution, 196, 239–246. doi: 10.1016/j.envpol.2014.10.009
- Statnano. (2019). https://statnano.com/
- Sun, T. Y., Bornhöft, N. A., Hungerbühler, K., & Nowack, B. (2016). Dynamic probabilistic modeling of environmental emissions of engineered nanomaterials. Environmental Science & Technology, 50(9), 4701–4711. doi: 10.1021/acs.est.5b05828
- Sun, T. Y., Gottschalk, F., Hungerbühler, K., & Nowack, B. (2014). Comprehensive probabilistic modelling of environmental emissions of engineered nanomaterials. Environmental Pollution, 185, 69–76. doi: 10.1016/j.envpol.2013.10.004
- Tan, W., Du, W., Darrouzet-Nardi, A. J., Hernandez-Viezcas, J. A., Ye, Y., Peralta-Videa, J. R., & Gardea-Torresdey, J. L. (2018). Effects of the exposure of TiO2 nanoparticles on basil (Ocimum basilicum) for two generations. Science of the Total Environment, 636, 240–248. doi: 10.1016/j.scitotenv.2018.04.263
- Tella, M., Auffan, M., Brousset, L., Issartel, J., Kieffer, I., Pailles, C., Morel, E., Santaella, C., Angeletti, B., Artells, E., Rose, J., Thiery, A., & Bottero, J. Y. (2014). Transfer, transformation, and impacts of ceria nanomaterials in aquatic mesocosms simulating a pond ecosystem. Environmental Science & Technology, 48(16), 9004–9013. doi: 10.1021/es501641b
- The Nanodatabase. (2019). http://nanodb.dk/
- Tong, T., Shereef, A., Wu, J., Binh, C. T. T., Kelly, J. J., Gaillard, J. F., & Gray, K. A. (2013). Effects of material morphology on the phototoxicity of nano-TiO2 to bacteria. Environmental Science & Technology, 47(21), 12486–12495. doi: 10.1021/es403079h
- Tong, T., Wilke, C. M., Wu, J., Binh, C. T. T., Kelly, J. J., Gaillard, J. F., & Gray, K. A. (2015). Combined toxicity of nano-ZnO and nano-TiO2: From single-to multinanomaterial systems. Environmental Science & Technology, 49(13), 8113–8123. doi: 10.1021/acs.est.5b02148
- Tou, F., Yang, Y., Feng, J., Niu, Z., Pan, H., Qin, Y., Guo, X., Meng, X., Liu, M., & Hochella, M. F. (2017). Environmental risk implications of metals in sludges from waste water treatment plants: The discovery of vast stores of metal-containing nanoparticles. Environmental Science & Technology, 51(9), 4831–4840. doi: 10.1021/acs.est.6b05931
- Venkatesan, A. K., Rodríguez, B. T., Marcotte, A. R., Bi, X., Schoepf, J., Ranville, J. F., Herckes, P., & Westerhoff, P. (2018). Using single-particle ICP-MS for monitoring metal-containing particles in tap water. Environmental Science: Water Research & Technology, 4(12), 1923–1932. doi: 10.1039/C8EW00478A
- Vijayaraj, V., Liné, C., Cadarsi, S., Salvagnac, C., Baqué, D., Elger, A., Barret, M., Mouchet, F., & Larue, C. (2018). Transfer and ecotoxicity of titanium dioxide nanoparticles in terrestrial and aquatic ecosystems: A microcosm study. Environmental Science & Technology, 52(21), 12757–12764. doi: 10.1021/acs.est.8b02970
- Wang, P., Menzies, N. W., Chen, H., Yang, X., McGrath, S. P., Zhao, F. J., & Kopittke, P. M. (2018). Risk of silver transfer from soil to the food chain is low after long-term (20 years) field applications of sewage sludge. Environmental Science & Technology, 52(8), 4901–4909. doi: 10.1021/acs.est.8b00204
- Wang, T., Long, X., Cheng, Y., Liu, Z., & Yan, S. (2014). The potential toxicity of copper nanoparticles and copper sulphate on juvenile Epinephelus coioides. Aquatic Toxicology, 152, 96–104. doi: 10.1016/j.aquatox.2014.03.023
- Wang, Z., Von Dem Bussche, A., Kabadi, P. K., Kane, A. B., & Hurt, R. H. (2013). Biological and environmental transformations of copper-based nanomaterials. ACS Nano, 7(10), 8715–8727. doi: 10.1021/nn403080y
- Wang, Z., Zhang, L., Zhao, J., & Xing, B. (2016). Environmental processes and toxicity of metallic nanoparticles in aquatic systems as affected by natural organic matter. Environmental Science: Nano, 3(2), 240–255. doi: 10.1039/C5EN00230C
- Welz, P. J., Khan, N., & Prins, A. (2018). The effect of biogenic and chemically manufactured silver nanoparticles on the benthic bacterial communities in river sediments. Science of the Total Environment, 644, 1380–1390. doi: 10.1016/j.scitotenv.2018.06.283
- Wilke, C. M., Tong, T., Gaillard, J. F., & Gray, K. A. (2016). Attenuation of microbial stress due to nano-Ag and nano-TiO2 interactions under dark conditions. Environmental Science & Technology, 50(20), 11302–11310. doi: 10.1021/acs.est.6b02271
- Wilke, C. M., Wunderlich, B., Gaillard, J. F., & Gray, K. A. (2018). Synergistic bacterial stress results from exposure to nano-Ag and nano-TiO2 mixtures under light in environmental media. Environmental Science & Technology, 52(5), 3185–3194. doi: 10.1021/acs.est.7b05629
- Wimmer, A., Markus, A. A., & Schuster, M. (2019). Silver nanoparticle levels in river water: Real environmental measurements and modeling approaches—A comparative study. Environmental Science & Technology Letters, 6(6), 353–358. doi: 10.1021/acs.estlett.9b00211
- Wright, M. V., Matson, C. W., Baker, L. F., Castellon, B. T., Watkins, P. S., & King, R. S. (2018). Titanium dioxide nanoparticle exposure reduces algal biomass and alters algal assemblage composition in wastewater effluent-dominated stream mesocosms. Science of the Total Environment, 626, 357–365. doi: 10.1016/j.scitotenv.2018.01.050
- Wu, Q., Nouara, A., Li, Y., Zhang, M., Wang, W., Tang, M., Ye, B., Ding, J., & Wang, D. (2013). Comparison of toxicities from three metal oxide nanoparticles at environmental relevant concentrations in nematode Caenorhabditis elegans. Chemosphere, 90(3), 1123–1131. doi: 10.1016/j.chemosphere.2012.09.019
- Wu, Q., Zhao, Y., Li, Y., & Wang, D. (2014). Susceptible genes regulate the adverse effects of TiO2-NPs at predicted environmental relevant concentrations on nematode Caenorhabditis elegans. Nanomedicine: Nanotechnology, Biology and Medicine, 10(6), 1263–1271. doi: 10.1016/j.nano.2014.03.010
- Wu, S., Zhang, S., Gong, Y., Shi, L., & Zhou, B. (2020). Identification and quantification of titanium nanoparticles in surface water: A case study in Lake Taihu, China. Journal of Hazardous Materials, 382, 121045. doi: 10.1016/j.jhazmat.2019.121045
- Xia, B., Zhu, L., Han, Q., Sun, X., Chen, B., & Qu, K. (2017). Effects of TiO2 nanoparticles at predicted environmental relevant concentration on the marine scallop Chlamys farreri: An integrated biomarker approach. Environmental Toxicology and Pharmacology, 50, 128–135. doi: 10.1016/j.etap.2017.01.016
- Xiao, B., Zhang, Y., Wang, X., Chen, M., Sun, B., Zhang, T., & Zhu, L. (2019). Occurrence and trophic transfer of nanoparticulate Ag and Ti in the natural aquatic food web of Taihu Lake. Environmental Science: Nano, 6(11), 3431–3441. doi: 10.1039/C9EN00797K
- Xu, L., Wang, Z., Zhao, J., Lin, M., & Xing, B. (2020). Accumulation of metal-based nanoparticles in marine bivalve mollusks from offshore aquaculture as detected by single particle ICP-MS. Environmental Pollution, 260, 114043. doi: 10.1016/j.envpol.2020.114043
- Yang, S. P., Bar-Ilan, O., Peterson, R. E., Heideman, W., Hamers, R. J., & Pedersen, J. A. (2013). Influence of humic acid on titanium dioxide nanoparticle toxicity to developing zebrafish. Environmental Science & Technology, 47(9), 4718–4725. doi: 10.1021/es3047334
- Ye, N., Wang, Z., Wang, S., & Peijnenburg, W. J. (2018). Toxicity of mixtures of zinc oxide and graphene oxide nanoparticles to aquatic organisms of different trophic level: Particles outperform dissolved ions. Nanotoxicology, 12(5), 423–438. doi: 10.1080/17435390.2018.1458342
- Yin, Y., Liu, J., & Jiang, G. (2012). Sunlight-induced reduction of ionic Ag and Au to metallic nanoparticles by dissolved organic matter. ACS Nano, 6(9), 7910–7919. doi: 10.1021/nn302293r
- Zhang, X., Yang, C. W., Yu, H. Q., & Sheng, G. P. (2016). Light-induced reduction of silver ions to silver nanoparticles in aquatic environments by microbial extracellular polymeric substances (EPS). Water Research, 106, 242–248. doi: 10.1016/j.watres.2016.10.004
- Zhang, X., Zhou, Q., Zou, W., & Hu, X. (2017). Molecular mechanisms of developmental toxicity induced by graphene oxide at predicted environmental concentrations. Environmental Science & Technology, 51(14), 7861–7871. doi: 10.1021/acs.est.7b01922
- Zhang, X., Zhou, Y., Xu, T., Zheng, K., Zhang, R., Peng, Z., & Zhang, H. (2018). Toxic effects of CuO, ZnO and TiO2 nanoparticles in environmental concentration on the nitrogen removal, microbial activity and community of Anammox process. Chemical Engineering Journal, 332, 42–48. doi: 10.1016/j.cej.2017.09.072
- Zhang, Y., Meng, T., Shi, L., Guo, X., Si, X., Yang, R., & Quan, X. (2019). The effects of humic acid on the toxicity of graphene oxide to Scenedesmus obliquus and Daphnia magna. Science of the Total Environment, 649, 163–171. doi: 10.1016/j.scitotenv.2018.08.280
- Zhao, J., Cao, X., Wang, Z., Dai, Y., & Xing, B. (2017). Mechanistic understanding toward the toxicity of graphene-family materials to freshwater algae. Water Research, 111, 18–27. doi: 10.1016/j.watres.2016.12.037
- Zhao, J., Dai, Y., Wang, Z., Ren, W., Wei, Y., Cao, X., & Xing, B. (2018). Toxicity of GO to freshwater algae in the presence of Al2O3 particles with different morphologies: Importance of heteroaggregation. Environmental Science & Technology, 52(22), 13448–13456. doi: 10.1021/acs.est.8b00815
- Zhao, J., Li, Y., Cao, X., Guo, C., Xu, L., Wang, Z., Feng, J., Yi, H., & Xing, B. (2019). Humic acid mitigated toxicity of graphene-family materials to algae through reducing oxidative stress and heteroaggregation. Environmental Science: Nano, 6(6), 1909–1920. doi: 10.1039/C9EN00067D
- Zhao, J., Liu, F., Wang, Z., Cao, X., & Xing, B. (2015). Heteroaggregation of graphene oxide with minerals in aqueous phase. Environmental Science & Technology, 49(5), 2849–2857. doi: 10.1021/es505605w
- Zhao, J., Ning, F., Cao, X., Yao, H., Wang, Z., & Xing, B. (2020). Photo-transformation of graphene oxide in the presence of co-existing metal ions regulated its toxicity to freshwater algae. Water Research, 176, 115735. doi: 10.1016/j.watres.2020.115735
- Zhao, J., Wang, Z., Mashayekhi, H., Mayer, P., Chefetz, B., & Xing, B. (2012). Pulmonary surfactant suppressed phenanthrene adsorption on carbon nanotubes through solubilization and competition as examined by passive dosing technique. Environmental Science & Technology, 46(10), 5369–5377. doi: 10.1021/es2044773
- Zhou, D., Abdel-Fattah, A. I., & Keller, A. A. (2012). Clay particles destabilize engineered nanoparticles in aqueous environments. Environmental Science & Technology, 46(14), 7520–7526. doi: 10.1021/es3004427