Abstract
As a quintessential “One Health” issue, antimicrobial resistance (AMR) can be transmitted in the environment via multiple pathways, thereby constituting an integral dimension of the human-animal-environment loop. Only recently has air been recognized as a potentially important pathway for the dissemination of antibiotic resistance genes (ARGs) and antibiotic-resistant bacteria. This review presents the current know-how about the hypothesized dissemination from emission sources to human airways in association with influencing environmental factors and the consequent health implications. The presence of ARGs in source-specific and ambient air is no longer in question. The transport propensity of antibiotic-resistant bacteria needs to be quantified in order to assess the magnitude of the inhalation exposure of occupational and general populations. Reactive atmospheric components and prevailing meteorological conditions strongly influence the dynamics of and exposure to ambient inhalable ARGs. Considering the evolving trends of air pollution and climate change, such impacts on antibiotic-resistant pathogens need to be systematically studied. The ultimate question is the fate and consequences of inhalable AMR in interaction with microbiomes in healthy and diseased human airways, which would shed light on the role of AMR in viral-bacterial co-infections leading to acute and chronic respiratory diseases. We put forward a holistic methodological framework to address the major research gaps to establish the airborne transmission chain of antimicrobial resistance. An advanced understanding of these issues will be of benefit in devising effective control and management measures to minimize the airborne transmission of AMR, an integral environmental dimension to protecting the health of large populations.
Graphical abstract
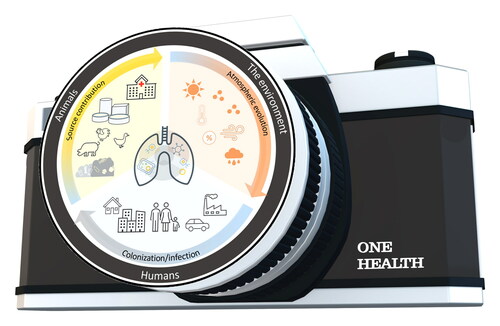
HANDLING EDITORS:
1. Role of environmental dissemination in the “One Health” framework of antimicrobial resistance
Antimicrobial resistance (AMR) is an intrinsic attribute of many environmental microorganisms. They harbor antibiotic resistance genes (ARGs) that encode resistance to the surrounding naturally existing antibiotics for self-protection or to their endogenous metabolites to inhibit the growth of other microbes that compete with them for niches (Martinez, Citation2012). Some soil microbes with intrinsic ARGs can even obtain nutrition by degrading the antibiotics in the surrounding environment (Allen et al., Citation2010). Such intrinsic ARGs are ubiquitous in the environment as functional genes of a kind in all ecosystems. In the case of other microorganisms, ARGs can be acquired by horizontal gene transfer (HGT) or mutation, which occurs spontaneously in the biosphere (Martinez & Baquero, Citation2000).
One of the most important discoveries of the 20th century, antibiotics have made great contributions to safeguarding human health and promoting animal husbandry. In recent years, however, the overuse and misuse of antibiotics across the globe has led to the emergence of antibiotic-resistant pathogens and “superbugs” (Aslam et al., Citation2018). The selective pressure imposed by antibiotics in the environment has been accelerating the spread of ARGs within and between bacterial species. The United Nations Environment Programme (Citation2017) has listed AMR as one of the six priority environmental issues with global implications, and the World Health Organization (WHO) has announced a strategic plan to control ARGs as one of the biggest public health challenges worldwide. According to O’Neill (Citation2016), by 2050, 10 million lives a year and a cumulative 100 trillion USD of economic output will be at risk due to the rise of drug-resistant infections if we are not able to find proactive solutions to this threat.
It is increasingly recognized that AMR is not just a simple matter of the overuse or misuse of antibiotics in hospitals. With a share of >60% in recent years, community acquisition has dominated over hospital acquisition in cases of resistant infection (). Reducing the consumption of antibiotics alone would not be sufficient to control AMR because the spread of resistant strains and resistance genes appears to be the dominant contributing factor (Collignon et al., Citation2018; Merlin, Citation2020). In addition to the well-documented clinical settings, the realms of the husbandry industry (Udikovic-Kolic et al., Citation2014), urbanization (Winglee et al., Citation2017), and the related natural environments (Li et al., Citation2015) are all included in the “One Health” perspective. “One Health” is a collaborative, multi-sectoral, and transdisciplinary approach to attain optimal health outcomes by recognizing the interconnection between people, animals, plants, and their shared environment (Mackenzie & Jeggo, Citation2019). Given the interdependence of human, animal, and environmental dimensions of AMR, it is logical to adopt a “One Health” framework integrating environmental and clinical settings engaging multi-sectoral efforts to tackle the challenge (Booton et al., Citation2021; Kim & Cha, Citation2021). This includes taking measures to preserve the continued effectiveness of existing or novel antimicrobials by eliminating their inappropriate use and by limiting the spread of infection (Berendonk et al., Citation2015; Hernando-Amado et al., Citation2019; Larsson et al., Citation2018). Apart from medication-induced antimicrobial resistance, AMR can be disseminated from the environment to humans through external exposure pathways, namely, through drinking water, food, skin contact, and inhalation (Huijbers et al., Citation2015). Combating AMR in the environment can contribute to a reversal of the projected trend of AMR and the associated public health and economic aftermaths (Ahmad et al., Citation2021; Surette & Wright, Citation2017). To this end, it is imperative to address fundamental questions about the flow of bacterial resistance and pathogenic hosts via these environmental intake routes, the internal fate of inhaled and ingested ARGs in the human body system, and the impact of these environmental resistomes. Finding answers to these questions is essential to determining the relative contribution to community-associated AMR of environmental and lifestyle factors vs. antibiotic use, and to charting a path to effective communication and collaboration between the environmental and clinical research communities concerning AMR.
Figure 1. Total reported numbers of patients infected from a confirmed origin (community or hospital) by specific pathogens under surveillance that show resistance to at least one antibiotic in 2018 (66 countries) and 2019 (70 countries). The data are sourced from the 2020 and 2021 reports of the Global Antimicrobial Resistance and Use Surveillance System (GLASS) that summarize the 2018 and 2019 data, respectively (https://www.who.int/initiatives/glass). The sites of infection and pathogens included in the GLASS are the bloodstream (Acinetobacter spp., Escherichia coli, Klebsiella pneumoniae, Salmonella spp., Staphylococcus aureus, and Streptococcus pneumoniae), urinary tract (E. coli and K. pneumoniae), gastrointestinal tract (Salmonella spp. and Shigella spp.), and genitals (Neisseria gonorrhoeae). Please note that infection cases of unknown origin have been excluded from this analysis and that this figure does not imply a temporal trend due to the evolving number of countries that report to the GLASS.
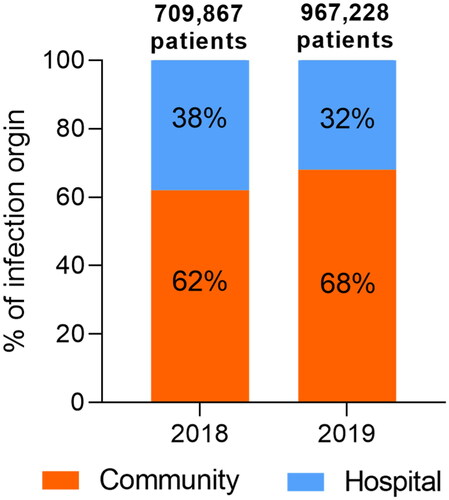
A growing body of research has shown that ARGs, including those with clinical relevance like blaNDM-1 and mcr-1, are becoming prevalent and enriched in non-clinical environments, such as some anthropogenic hotspots (e.g., wastewater treatment plants (WWTPs), landfills, and livestock farms), and then in the receiving environments (e.g., soil, surface water, and sediment), where there is low abundance in relatively pristine places (Chen et al., Citation2013, Citation2016; Wu et al., Citation2017). Meanwhile, mobile genetic elements (MGEs) (e.g., integrons, transposons, plasmids, and bacteriophages) that enable DNA to mobilize either within a given cell or between bacterial cells have been widely detected in the environment (Che et al., Citation2021; Gillings et al., Citation2015). The ability of MGEs to either capture ARGs or transfer them between bacteria—and the aptitude of bacteria under certain conditions to uptake extracellular DNA—can facilitate the dissemination of ARGs in environmental bacterial communities (Berglund, Citation2015; van Hoek et al., Citation2011; Wang et al., Citation2020).
Since their recognition as a class of emerging contaminants, ARGs have attracted increasing attention in terms of their environmental dissemination pathways (Berendonk et al., Citation2015; Pruden et al., Citation2006; Vikesland et al., Citation2017). Previous studies have focused primarily on the source end, including source water-treatment/distribution system-tap water, irrigation/manure-soil-agricultural produce, and livestock/poultry-animal products, surface sources-ambient air (McEachran et al., Citation2015; Murray et al., Citation2019; Zhu et al., Citation2013). However, these environmental sources and major pathways have rarely been explored in relation to human exposure and potential health implications ().
Figure 2. Transmission routes of antibiotic-resistant bacteria (ARB) into humans from environmental sources (Reprinted from Trends in Microbiology, Volume 25, Manaia C.M., Assessing the risk of antibiotic resistance transmission from the environment to humans: Non-direct proportionality between abundance and risk, pp. 173–181, Copyright (2017), with permission from Elsevier).
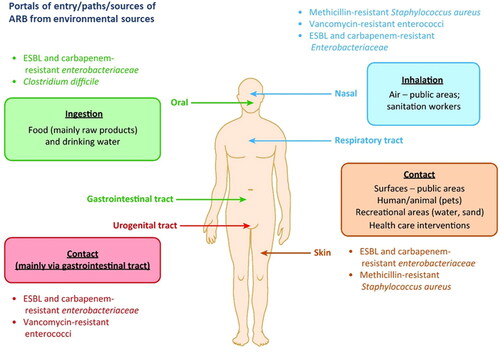
Although dust was mentioned as early as 1973 as a source of antibiotics from feedlots to the atmosphere (Shuyler, Citation1973), it was not until very recently that air was recognized as a potentially important pathway for the dissemination of ARGs and ARB (Li, Cao et al., Citation2018; Li, Wang et al., Citation2020; McEachran et al., Citation2015; Xie et al., Citation2018). The inhalation of urban airborne fine particulate matter (PM2.5) represents a comparable share in the human daily intake of some common environmental ARGs as the ingestion of drinking water and food (Li, Wang et al., Citation2020; Xie, Jin et al., Citation2019). This finding establishes the critical contribution of airborne PM2.5 in the environmental dissemination and consequent human health implications of ARGs.
In the clinical context, there is a growing volume of studies addressing pathogens and “superbugs,” particularly those associated with multi-drug resistant infections (Borgia et al., Citation2012). However, the environmental processes (e.g., foodborne, waterborne or airborne) by which these resistant pathogens find their way into humans remain largely unknown at this stage. In a broader sense, new insights are required to understand how environmentally transmitted bacterial communities influence their counterparts in the respiratory and gastrointestinal tracts and exchange genetic materials (e.g., ARGs) upon inhalation and ingestion. The resistomes in human airways and skin have been described as displaying a high degree of similarity, while being distinct from those observed in gastrointestinal, oral, and urological settings (Pal et al., Citation2016). This evidence underpins the disparities between inhalation and ingestion pathways to shape the human resistome. A concerted effort is thus needed to track bacterial resistance in humans to externally sourced counterparts other than selection pressure from the consumption of prescribed antibiotics. Improved knowledge of the transmission of clinically relevant ARGs and hosts shared between the environment and human gut/airways is critical to discerning and addressing the health implications, and in turn to prioritizing the key exposure pathways as control targets.
In this review, we aimed to achieve a synthesis of current understandings of the airborne dissemination and transmission of AMR, which used to be an under-appreciated occurrence but is gaining increasing recognition because of its importance in the environmental dimension of AMR and in the overall “One Health” human-animal-environment loop. We discussed the remaining puzzles regarding the human health impacts of airborne AMR from different environmental sources to human airways (), and proposed a methodological framework to address the key scientific questions () (elaborated upon in detail in Sections 2–5). Advances in these aspects will enable us to contribute to reversing the projected trend of human health and economic losses due to the worldwide AMR crisis as the public health challenge of the 21st century.
2. Airborne dissemination of ARGs and airborne transmission of resistant bacteria as part of fine particulate matter
Airborne fine particulate matter (PM2.5) contains a cocktail of microorganisms (Cao et al., Citation2014). The rate at which ARGs are inhaled has been estimated as ranging from 103 to 104 copies/day/adult (Xie, Jin et al., Citation2019). Global exposure to pollution from airborne particulate matter has been increasing by 20% over the last two decades, and now affects 91% of the world’s population (Health Effects Institute, Citation2020). This environmental pollution issue is exacerbating the AMR risk to human beings, as PM2.5 is the most inhalable portion of the total suspended particles. The increase should be accompanied by a substantially increased intake rate of airborne ARGs. Notably, PM2.5 pollution and AMR risks are both rising in the developing world, especially in Asia, which hints at a huge threat to human health (Laxminarayan et al., Citation2016; Li, Jin et al., Citation2019). It should be noted that, in comparison to other exposure pathways (e.g., drinking water and food), more than 80% of the materials in these airborne PM2.5 can be deposited in human bodies and penetrate deeply into the airways, i.e., the alveolar region of the human lung (Kwon et al., Citation2020; Meng et al., Citation2013). In addition, the PM2.5 related AMR could become more pronounced due to (i) the stable condition of bioaerosols containing PM2.5-ARGs prolonging the periods of contact between ARGs and the bacterial community, which would facilitate the HGT of ARGs and increase the occurrence of AMR in PM2.5 (Wei et al., Citation2016); and (ii) the tendency of airborne bacteria to exhibit higher bio-toxicity and the increased hazards under severely polluted PM2.5 conditions, leading to enhanced infection rates for human beings (Burnett et al., Citation2018). In addition, traffic exhaust contributes a large portion of PM2.5 into the urban ambient air and the bio-toxicant materials that it contains can impose selective AMR pressure on some airborne bacteria (Zhang, Gu et al., Citation2018).
Inhalable ARGs can be emitted from multiple urban sources as a result of aerosolization. Previous studies on the profile of PM2.5-antibiotic resistomes were only conducted in divided field investigations (He et al., Citation2020), such as in a single spot in a hospital, a suburban residential zone, the aeration process in WWTPs, and a municipal waste landfilling platform (Li, Wang et al., Citation2020). The role of ambient airborne PM2.5, which connects all antibiotic resistomes emitted from various urban settings, remains unclear. While the transmission mediated by inhalable particles occurs at short ranges and soon after aerosolization, PM2.5 can easily be dispersed and diluted in the air. The bioaerosols can travel long distances and remain in the atmosphere for long periods (Rodo et al., Citation2014; Smith et al., Citation2012). Genes encoding resistance to tetracycline antibiotics have been found to be 1,000 times more abundant in PM collected downwind of emission sources (a feedlot) as compared to upwind (McEachran et al., Citation2015). However, the distributions and relationships among different emission sources/areas have not been systematically analyzed. The long-distance transport of airborne PM2.5 has been observed to occur on a large regional scale (Cáliz et al., Citation2018). Therefore, it is imperative to decipher potential AMR interactions between different PM2.5 emission sources in urban ambient air.
Aerosol-associated bacteria showing resistance to different antibiotics such as macrolides, beta-lactams, tetracyclines, fluoroquinolones, and sulfonamides, have been continuously isolated from clinical settings and animal farms where a variety of antibiotics are used (Gibbs et al., Citation2006; Gilbert et al., Citation2010), and are also found in the urban atmospheric environment (Gandolfi et al., Citation2011; Liang et al., Citation2020; Mao et al., Citation2019; Zhang et al., Citation2019; Zhao et al., Citation2020). This isolation indicates the ubiquitous presence of airborne ARGs encoding the corresponding antibiotic resistance. Aerosol-associated bacteria and ARGs that withstand long-range transport via air movements and dust storms are likely to influence the local airborne bacterial and ARG profiles of downwind locations (Gat et al., Citation2017; McEachran et al., Citation2015). Using culture-independent methods, researchers have shown that the absolute and relative abundance of airborne ARGs can evolve frequently over time and across geographical gradients (Li, Cao et al., Citation2018; Liang et al., Citation2020; Wang, Wang et al., Citation2019; Zhang et al., Citation2019).
Beyond ARGs, risk-oriented investigations must be directed to antibiotic-resistant bacteria (ARB), particularly those that are able to colonize the human body (vectors), either transiently or as residents, and simultaneously harbor acquired ARGs. The AMR surveillance programs conducted worldwide have identified a few genera or species that fall within the description of widely disseminated AMR vectors (Molton et al., Citation2013), such as methicillin-resistant Staphylococcus aureas (MRSA), vancomycin-resistant Enterococci (VRE), extended-spectrum beta-lactamase (ESBL), and carbapenemase-producing Enterobacteriaceae, which can be transmitted via inhalation (as illustrated in ). If such vectors have high fitness in the human body, have acquired resistance to multiple classes of antibiotics, and are able to express specific virulence factors, they can be considered superbugs (i.e., multi-drug resistant pathogens). The threat posed by the vectors may vary depending on their capacity to disseminate ARGs to the host microbiome via free DNA (transformation), plasmids (conjugation), and/or phages (transduction), and to promote genetic recombination through transposons or integrons.
Although a growing number of investigations have provided valuable information on airborne ARGs near potential emission sources and in urban areas, most of them either mainly focused on coarser particles (e.g., TSP and PM10) (Gat et al., Citation2017; Li, Cao et al., Citation2018; Ling et al., Citation2013; Mazar et al., Citation2016) or considered a short-term snapshot of the situation in small-scale areas (Hu et al., Citation2018; Sun et al., Citation2020; Zhang et al., Citation2019). Meanwhile, knowledge of airborne antibiotic-resistant bacteria (ARB) is rather limited. Hence, information on the spatiotemporally resolved dynamics of aerosol-associated ARB and ARGs, especially those as part of PM2.5, is still required to assess long-term human exposure via inhalation on a larger scale from the regional to the global.
3. Contribution of anthropogenic sources to airborne antibiotic resistomes
Livestock farms, WWTPs, landfills, and hospitals are generally considered to be critical anthropogenic reservoirs of ARGs and bacteria, especially for opportunistic pathogens (Smets et al., Citation2016); hence, the occupational exposure risks at these locations merit close attention. The importance of investigating microbial emissions has already been emphasized by many researchers, who have revealed the frequent occurrence of respiratory symptoms in workers in WWTPs and farms with high levels of airborne microbes (Eduard et al., Citation2001; Melbostad et al., Citation1994). Previous publications have reported that concentrations of source-indicative bacterial and ARG species in the atmosphere fall in descending order as the distance away from bacteria- and ARGs-rich environments like poultry manure and municipal solid waste sites increases, which hints at the emission of microbes and ARGs from solid/water matrices to ambient air in bioaerosol form (Gao et al., Citation2016; Li, Wang et al., Citation2020).
The evidence is emerging to distinguish PM2.5-associated antibiotic resistomes from those in terrestrial and marine systems (Pal et al., Citation2016). These observations suggest that the atmospheric environment is a unique reservoir of ARGs, with varying stresses shaping their abundance and compositions. More data have enabled qualitative comparisons to be made of antibiotic resistome profiles between potential source matrices and nearby aerosol-bound ARGs. On such a basis, various typical sources including natural sources such as marine and soil dust (Gat et al., Citation2017; Liang et al., Citation2020) and other anthropogenic hotspots like animal feces in livestock farms, WWTPs, hospitals, and solid waste treatment systems (He et al., Citation2020; Li, Wang et al., Citation2020; Yang et al., Citation2018) have been identified as contributing ARGs to the ambient atmosphere. Most existing studies on the aerosolization potential of bacteria from potential sources have been conducted in selected WWTPs. Bioaerosol emission levels in WWTPs subjected to a strong impact by agitation methods could vary along with the treatment phases (Fathi et al., Citation2017; Gotkowska‐Płachta et al., Citation2013; Li et al., Citation2016), during which the aeration process can generate aerosols of less than 1 μm in size with a unimodal pattern peaking at the nanometer scale (Han, Yang, Yang, et al., Citation2019). By using either culture-dependent or fluorescence-based methods, WWTP-emitting bioaerosol particles have been found concentrating in inhalable range (2–3 μm) (Hsiao et al., Citation2020), while a considerable portion (20%–40%) of aerosolized bacteria is able to penetrate the human lung region (<2.1 μm) (Brandi et al., Citation2000; Han, Yang, Chen et al., Citation2019; Li et al., Citation2011). Additional studies also pointed out that the aerosolization ratio of culturable bacteria in sewage could fall within levels of 10−11 to 10−9 (expressed as colony-forming units [CFU] m−3 air/CFU m−3 wastewater) (Bauer et al., Citation2002; Wang et al., Citation2021). These findings highlight the biohazards of these emission sources to exposed human beings. To mathematically describe the kinetics of aerosolization, simple one-box models can be applied to estimate the potential for the emission of bacteria from different treatment trains of a WWTP or landfill site to the ambient air based on mass conservation. Other possible methods for measuring the emission rate of microorganisms could be based on dispersal models using meteorological parameters and concentrations of bioaerosols away from sources at different downwind distances (Li et al., Citation2013).
Compared with airborne bacteria, only a few studies have touched upon ARG emissions from key sources. Some studies have isolated opportunistic pathogens with AMR from bioaerosols collected in WWTPs (Zhang, Zuo et al., Citation2018), while others based on metagenomic sequencing have only compared the ARG profiles of activated/dewatered sludge and bioaerosol in WWTPs without attempting further quantitative estimations of the contributions (Han & Yoo, Citation2020). A bayesian community-wide culture-independent microbial source tracking tool (SourceTracker) has gained popularity in airborne bacterial studies (Knights et al., Citation2011). Based on this method, Yang et al. (Citation2019) attributed 4%–17% of airborne bacteria within a WWTP to wastewater and sludge; the data provided quantitative information of contributions from WWTP by-products to airborne bacteria. However, air samples from downwind rather than upwind sites of the WWTP were used as a potential source of the indoor airborne microbes in the WWTP. Such a design ignores the potential aerosolization of sewage-/sludge-relevant bacteria and their transport to the downwind air, which may lead to an underestimation of the contribution from WWTP matrices to airborne bacteria. Taking this problem into consideration, the feasibility of SourceTracker2 in predicting the contributions of different ARG sources was verified in environmental samples, including sediment and urban stream (Baral et al., Citation2018; Li, Yin et al., Citation2018). Its application should in future be extended to airborne antibiotic resistomes and resistant bacteria.
4. Evolution of antibiotic resistomes in ambient air under physicochemical influences
Once emitted from surface ecosystems to the atmosphere, bacteria and associated ARGs will further evolve along with atmospheric processes (Zhang et al., Citation2019). Airborne bacterial communities are unlikely to experience co-occurring anthropogenic stressors (e.g., antibiotics, biocides, metals) as selective pressure for AMR in the air. Instead, they are subjected to a high degree of dispersion, as well as to high oxidation capacity, particularly in urban air (Zhen et al., Citation2017).
Airborne bacterial communities are influenced by a range of meteorological factors and gaseous and particulate constituents. An analysis of snowfall samples from several countries has revealed that precipitation of ubiquitous ARGs in fresh snow can be exacerbated by air pollution (Zhu et al., Citation2021). A strong correlation between integrons and ARGs and a high air quality index (poor air quality) and no significant correlation with a low air quality index (good air quality) would suggest that air pollution has a significant impact on both the diversity and abundance of airborne ARGs and MGEs.
Oxidative gases (i.e., O3 and NOx) can degrade proteins/polypeptides into forming amino acids (Song et al., Citation2017; Wang, Song et al., Citation2019; Xu et al., Citation2020) and inducing bacterial lysis (Scott & Lesher, Citation1963; Thanomsub et al., Citation2002), leading to the release of free DNA to the extracellular environment. Free ARGs may thus be influenced by oxidative gases and ultraviolet radiation, which can act in a manner similar to disinfection techniques (He et al., Citation2019; Nihemaiti et al., Citation2020). Phage-associated ARGs are generated in metabolically active hosts during a viral infection and assemble to transfer ARGs among hosts (Frost et al., Citation2005). Relative humidity is one of the important factors modulating the survival and infectivity of viruses (Lin & Marr, Citation2020; Kormuth et al., Citation2018), which may have implications for phage-associated ARGs. This may determine the alternative strategies of airborne bacteria in adapting to stressors, such as oxidative gases, free radicals, strong irradiation, and nutrient limitations. Differentiating between natural and anthropogenic sources of airborne ARGs and between prevailing weather conditions and physicochemical pollution status is an essential, but challenging task in assessing spatiotemporal scenarios of human inhalational exposures. In a recent study, He and coworkers employed a variation partitioning analysis to estimate differential influences from meteorological factors and oxidative gases on geographically disparate profiles of intracellular and extracellular 16S rRNA genes and ARGs (He, Jin, Xie et al., Citation2021). The prevailing meteorological conditions and oxidative gaseous pollutants explained >60% of the total variance in the intracellular–extracellular partitioning of the 16S rRNA gene and ARGs in several temperate and subtropical urban areas. While the fraction explained solely by meteorological factors increased from temperate to subtropical cities, the interactive effects of meteorological factors and oxidative gaseous components decreased. In temperate cities like Beijing, the strong interactive effects of prevailing meteorology and photochemical pollution jointly drive the seasonal succession of bacterially associated, free, and phage-associated ARGs. In subtropical cities like Hong Kong, meteorological factors dictate the intracellular–extracellular partitioning of the 16S rRNA gene and ARGs in the air (He, Jin, Xie et al., Citation2021).
Validating the above statistical inference requires the use of transformative approaches to decipher the physicochemical processes that affect the biological decay rates of resistant bacteria in aerosol droplets, which are central to the understanding of their airborne transmission (He, Jin, & Li, Citation2021). A combination of the use of comparative kinetic electrodynamic balance and controlled electrodynamic levitation, and the extraction of bioaerosols onto a substrate can be employed to explore the true airborne state of airborne bacteria (Fernandez et al., Citation2020). The tandem system would allow for the simultaneous quantitation of the dynamic behavior of aerosol particles and the bioaerosol survival rates of identical particle types as a function of time, particle composition, and environmental conditions. In addition, controlled environmental chambers, such as a rotating drum-based chamber setting, can be used to investigate the changing viability and AMR of key host bacteria co-aerosolized with PM in characteristic relative humidities, temperatures, and ozone pollution episodes (Kormuth et al., Citation2018). O3 can be generated by exposing air to a UV lamp, and can be measured by UV absorption, with standard PM being aerosolized into the drum using a dry powder disperser (Tiwari et al., Citation2013). These efforts would enable a mechanistic probe into the complex interconnections between airborne microphysics and biological decay that affect the viability and fitness cost of resistant bacteria subject to atmospheric processes.
The influences of air pollution on resistant bacteria are not confined to the air but extend to the airway upon inhalation. Ambient and source-specific PM2.5 have been shown to stimulate the plasmid-mediated conjugative transfer of ARGs between donor and recipient E. coli strains (Xie, Gu et al., Citation2019; Zhou et al., Citation2021). The formation of intracellular reactive oxygen species and the subsequent induction of oxidative stress, SOS response, changes in membrane permeability, and alterations in the mRNA expression of genes involved in HGTs have been implicated as underlying mechanisms (Xie, Gu et al., Citation2019; Zhang, Gu et al., Citation2018; Zhou et al., Citation2021). City-specific ambient PM2.5, in particular, have exhibited differential slopes in their concentration-response in promoting conjugative transfer efficiencies (Xie, Gu et al., Citation2019), that is, the differential potency per unit mass of PM2.5. This observation implies that differential mixture components in PM2.5 contribute to the joint effects that facilitate conjugative transfer. Apart from PM2.5, acute inhalation of ozone could alter airway permeability and microbiota, thus increasing the susceptibility of exposed human subjects to respiratory infection (Niu et al., Citation2020).
In view of the evolving gas-particle cocktails worldwide, it is imperative to identify via integrated mixture-toxicity modeling the key airborne constituents that are driving the combined effects on airway microbiomes and resistomes (Jin et al., Citation2017, Citation2019). Achieving coherence of evidence over the in vitro-in vivo-epidemiological continuum also requires consistency in the use of methodologies to determine exposure, such as the air-liquid-interface in vitro exposure systems (Seurat et al., Citation2021), animal exposure models (Li, Hu et al., Citation2020), and controlled exposure studies on human subjects (with vs. without filters/masks; Niu et al., Citation2020) to reflect the realm of human exposure scenarios.
Linking the atmospheric dynamics of ARB and ARGs to prevailing meteorology and air pollution puts evolving global climate and pollution scenarios into perspective for determining the environmental dissemination of AMR. Geographically comparative studies coupled with controlled laboratory experiments are needed to decipher the mechanisms by which atmospheric processes can modify the antibiotic resistomes in airborne PM2.5 for a complete understanding of geographically driven human health impacts via inhalable airborne microbiomes.
5. Health implications of bacterial communities and antibiotic resistomes at the ambient air-human airway interface
These aforementioned typical emission sources in urban areas are known to release AMR materials into ambient air. Sometimes this may result in illness requiring hospitalization, but the explicit implications for exposure at the air-human interface have not been well investigated. A completed aerial transmission of inhalable AMR can be affected by i) concentrations of air PM2.5 in the transmission zones; ii) the viability of antibiotic resistant bacteria and the HGT potential of ARGs in emitted PM2.5; and iii) environmental PM2.5-AMR deposition efficiency (e.g., nostril, throat, and lung tissues) in the human airways. These factors are heavily influenced by source-specific emission fluxes, urban meteorological characteristics, and the health of the receptors’ respiratory systems.
Regarding the human-atmosphere interfaces, there are several scenarios to consider with regard to aerial AMR transmission. The first scenario is occupational exposure in source sectors, such as livestock farms, WWTPs, landfills, and hospitals, while the scale of the affected population is relatively limited. High levels of resistant pathogen groups (e.g., multidrug-resistant Enterococcus, coagulase-negative Staphylococci, and viridans group Streptococci) have been detected in the air of a concentrated swine-feeding operation (Chapin et al., Citation2005). The nasal carriage of livestock-associated, antibiotic-resistant S. aureas, a risk factor in developing skin and soft tissue infection (Wardyn et al., Citation2018), was reduced in industrial hog operation workers by the wearing of face masks (Nadimpalli et al., Citation2018). While suggesting the utility of personal protective equipment in limiting occupational exposure, these findings provide evidence of the transfer of multidrug-resistant bacterial pathogens from source-specific waste streams to workers in these occupational settings via inhalation. The second scenario is community exposure downstream from source sites. The blaTEM-1 gene harbored by Bacillus showing resistance phenotypes has been found to be phylogenetically identical in leachate/solid wastes and downwind air (Li, Wang et al., Citation2020), thus posing potential risks to the surrounding communities. Specific contributions of each source to the transmission of PM2.5-AMR to the neighboring community (residents) and the different emission-source comparisons in relation to the seasonal variations are not clearly known. Panel studies of human subjects in source-influenced communities are required for a full revelation of the extent to which the typical urban emission sources can pose transmission risks to a large number of community residents. The AWARE study (Antibiotic Resistance in Wastewater—Transmission Risks for Employees and Residents around Wastewater Treatment Plants; http://www.klinikum.uni-muenchen.de/AWARE-STUDY/en/project/index.html) conducted across four European countries is a pioneering example of the evaluation of the risk of the transmission (including airborne transmission) of prevalent resistance phenotypes to nearby residents as well as to WWTP employees (Wengenroth et al., Citation2021). The outcomes are expected to provide evidence-based support for possible mitigation strategies. The third scenario is exposure of the general population in dense urban indoor and outdoor environments without specific waste stream sources, which is virtually uncharacterized for AMR, but is increasingly recognized as playing a role in the spread of respiratory infectious diseases. Continuous efforts are needed to determine the risk to individuals in indoor settings and for communities in proximity to emission hotpots of antibiotic-resistant bacteria. It is equally important to establish the role of the (outdoor) ambient atmosphere that connects neighboring communities and exposes the large urban population to antibiotic-resistant bacteria. In such a scenario, both short-range and long-range aerosol transmission subject to varying atmospheric conditions merit systematic investigation. PM2.5 has been found to be an independent risk factor in the acquisition of methicillin-resistant S. aureas in young children with cystic fibrosis (Psoter et al., Citation2017). Disentangling the interplay underlying such associations would definitely substantiate the principal link in the continuum of air pollution, airborne resistant bacteria, innate immunity, and respiratory infection (Lin et al., Citation2018; Mokoena et al., Citation2019; Wu et al., Citation2020).
Thus far, the abundance and diversity of ARGs and, above all, the colonization of resistant bacterial taxa in the human respiratory microbiome remain poorly characterized. It is important to uncover the process that mediates between colonization and infection to unveil the role of environmental sources in the airborne transmission of ARGs and ARB to humans. Colonizing bacteria can be present on skin or mucosa but are incapable of penetrating into tissues; however, infectious bacteria have the capacity to proliferate and invade the host, leading to an immune reaction typical of infectious diseases (Mushtaq et al., Citation2011). In healthy individuals, silent colonization may be caused by the resident vector of ARGs or the commensal microbiota receiving ARGs from transiently colonizing vectors (Manaia, Citation2017). The silent colonization accrued over a long term may evolve to an endogenous infection if the host reaches an immunocompromised state. In immunocompromised individuals, acute colonization from external sources may occur with the immediate invasion of the host. Characterization of ARG expression in the upper respiratory tract could shed light on the role played by AMR in the pathogenesis of bacterial infection. Recent studies have indicated that the host’s response to an influenza infection could indirectly affect ARG expression in the respiratory tract by impacting the microbial community structure and overall microbial gene expression (Zhang et al., Citation2020). Interactions between the systemic responses of the host to influenza infection and ARG expression highlight the importance of viral-bacterial co-infection in acute respiratory infections. The general characteristics of the baseline bacterial communities and resistomes have yet to be established among healthy urban populations.
The microbial exchange between ambient air and human airways is bidirectional, with microbial immigration and elimination into and out of the airways (Dickson & Huffnagle, Citation2015), meaning that humans themselves are a source of ARGs and ARB apart from sites of anthropogenic activities. This human source is particularly important in densely populated urban areas and has strong implications for airborne transmission amongst other routes. Panel studies of human subjects along with other emission sources need to be established. Taking ethical and logistical constraints into consideration, easy-to-access samples (e.g., exhaled gas condensate, skin and nasopharyngeal swabs) from human subjects can be collected synchronously with ambient and source-specific air samples over seasonal cycles. Human commensal (e.g., E. coli, Enterococcus spp.) or pathogenic/virulent bacteria (e.g., S. aureus, K. pneumoniae) can be isolated from the swabs for antimicrobial susceptibility tests and whole genome sequencing of resistant bacterial strains. With regard to source predictions of resistance between human and ambient air, the multivariate method Discriminant Analysis of Principal Components (DAPC) model is designed for identifying and describing clusters of genetically related individuals and for assigning individuals to groups (Li, Bi et al., Citation2019). The constructed model can be used to predict the possible genetic sources of specific resistance carriages between the urban-relevant environment-animal sectors and humans. The ARGs that are identified, especially those located on the plasmids, can be holistically compared (i) between ambient air and human airway samples; and (ii) across different sampling zones to confirm the existence of the hypothesized dissemination chain from “emission sources to human airways.”
6. The way forward
The existing evidence substantiates the occurrence of airborne ARGs and ARB in source sectors and the ambient environment at the breathing zone. It is also clear that air pollution and prevailing meteorology have a strong influence on the dynamics of human exposure to ambient inhalable ARGs. Nevertheless, the transport propensity of particular resistant bacterial pathogens subject to indoor and/or outdoor atmospheric physics and chemistry conditions needs to be quantified in order to assess the magnitude of exposure among occupational groups at source sites, communities downwind from the source settings, and the general population in dense urban environments without apparent sources. The fate and consequences of inhalable AMR in interaction with microbiomes in the human airway remains largely unexplored.
The review pinpointed two major research needs of regulatory relevance for future studies: (1) the abundance and diversity of antibiotic resistomes and the flux of the antibiotic resistant bacteria from sources to ambient air via airborne PM2.5; and (2) the hypothesized dissemination from putative emission sources to the human airway and the influencing environmental factors and potential health implications. Geographically comparative studies across antibiotic source diversities, air pollution gradients, and climate zones are required to understand the contribution of various source sectors to airborne bacterial communities and antibiotic resistomes. Controlled chamber simulations are also needed to decipher the microscale mechanisms by which aerosolisation quantitatively transfers antibiotic-resistant bacteria from surface sources into ambient air and subsequent atmospheric processing alters the viability and/or resistance traits of these bacteria. Moreover, parallel panel studies of human subjects are desired in the surveyed environments to isolate commensal or pathogenic/virulent bacteria from accessible respiratory samples. Sequencing of the resistant bacteria strains at the plasmid and genome levels would resolve the geophylogenetic relationships between airway resistance and airborne resistance for fate prediction and impact assessment. Addressing these critical issues would enhance our fundamental understanding of inhalable AMR in ambient inhalable particles, as an integral part of the “One-health” conception, and of the role of urban living/production activities in the transmission pathways of airborne AMR. In addition, novel insights will be generated into the biohazards of inhaled ARB and their deposition on human airways by considering their seasonally resolved profiles and dynamics. Therefore, it is vital to integrate the environmental aspects into an enriched understanding of the complex dynamics of airborne AMR between ambient air and urban communities. This will be of benefit in devising appropriate control measures and effective management measures to safeguard the health of large urban populations.
Putting the airborne transmission of AMR into the human-animal-environment loop, critical knowledge gaps remain with regard to the extent to which environmental ARB and ARGs promote the acquisition and spread of AMR among clinically relevant bacteria, and on the issue of whether ARGs that are acquired by both clinically relevant bacteria and strictly environmental bacteria originate from the same reservoirs (Smalla et al., Citation2018). For these issues to be properly addressed, global efforts are required to characterize and quantify the recent origins of mobile ARGs in the environment (Ebmeyer et al., Citation2021). In particular, it is necessary to give adequate support to advanced assessment of biological risks (Manaia, Citation2017; Murray et al., Citation2020). Such evaluations are needed to determine how contaminated environments select for AMR and/or favor its cross-media movement (Kraupner et al., Citation2021; Xu et al., Citation2021). The absence of a significant overlap of antibiotic-resistant bacteria (ARB) and ARGs between the human microbiome and potential environmental sources should not be interpreted as an indication of the absence of risk. The risk is a function of bacterial fitness in the human body and the presence of resistance and virulence genes. Even at extremely low abundance in environmental sources, ARB may represent a high risk for human health. As such, an accurate measure of the risk of environmental transmission to humans should go beyond a mere screening of ARG pools to ARB that are able to colonize and proliferate in the human body. A more refined risk assessment would guide the development and/or implementation of tailored technological solutions for priority ARB and ARGs at source.
Community acquisition of AMR and related infections are becoming a widespread challenge to global public health, in addition to those acquired in hospitals (Knight et al., Citation2018). Addressing community-acquired AMR requires an adaptive framework where various community or household environmental settings (natural or built, indoor or outdoor) constitute the key pathways or media through which the general population is exposed. It is imperative to shift the current research paradigm of community- and hospital-acquired AMR from a narrow focus on segregated settings or ecosystems toward a full picture of environmental pathways in close proximity to the exposure of the general urban population. Current molecular epidemiological analyses of AMR at the genomic and strain levels are often correlated to household demographics, social contact information, and details of antibiotics exposure to infer transmission events (Shen et al., Citation2018). More integrated approaches are required to resolve the issue of where the transmission and acquisition of community-acquired AMR occurred—to what extent indoor or outdoor environmental settings contributed (). Active dialogues and concerted efforts of environmental and clinical microbiologists, environmental chemists, epidemiologists, and modelers are required to make such a determination. In addition to molecular epidemiological inferences on the transmission dynamics of AMR in community settings, mechanistic elucidation can be achieved in vivo of the colonization of exogenous resistant bacteria and the de novo emergence of resistance arising from community environmental exposures and/or (un)intentional antibiotic dosages (Duan et al., Citation2020; Lin, Chen et al., Citation2020). Data from the environmental and household surveillance and the in vivo mechanistic study can be incorporated into transmission dynamics mathematical models to quantify where the acquisition of community-associated AMR occurred and the contribution of various environmental settings (Knight et al., Citation2018). The effectiveness of practicable interventions in reducing transmission can be assessed within the model, such as various levels of improved public disinfection efforts, personal and home hygiene practices at critical checkpoints, and the thorough cooking of food. Therefore, a concerned community needs to develop a broad sense of the environmental dimension relevant to the exposure of the general population, mechanistic insights into how exogenous AMR is acquired and colonized, and quantitative approaches to dissecting the contributions of various environmental settings to the community acquisition of AMR. Breaking the critical barrier will boost the ability of healthcare authorities to exercise precise checkpoint control to break the chain of transmission in specific communities and large urban populations.
7. Conclusion
With extensive studies reporting the occurrence of ARGs in the air of source settings and ambient environments, “what next” questions regarding the human health impacts upon inhalation need to be addressed. For the environmental dimension, there is an imperative need to shift from genetic detection of ARGs to integrated genotypic and phenotypic assessments of ARB and HGT events and embrace medical microbiology to decipher the consequences arising from the colonization and infection of inhalable resistant pathogens. For the clinical dimension, it is equally important to develop a broad sense of the environmental regime, including the airborne transmission, which may contribute to community- and hospital-acquired AMR and infection. The “One Health” framework encourages such an interdisciplinary and multi-sectoral synergy to connect the dots between sources, evolution, and impacts of airborne AMR. Similar efforts are going on in the drinking water, soil-crop, and animal food production systems that are relevant to population exposures. Establishing the chain of evidence for all these environmental pathways will provide a full picture of the key checkpoints where the spread and acquisition of AMR is favored beyond the inappropriate use of antimicrobials. The regulatory bodies will then be better informed to devise effective mitigation measures for public health benefits.
Additional information
Funding
References
- Ahmad, I., Malak, H. A., & Abulreesh, H. H. (2021). Environmental antimicrobial resistance and its drivers: A potential threat to public health. Journal of Global Antimicrobial Resistance, 27, 101–111. https://doi.org/10.1016/j.jgar.2021.08.001
- Allen, H. K., Donato, J., Wang, H. H., Cloud-Hansen, K. A., Davies, J., & Handelsman, J. (2010). Call of the wild: antibiotic resistance genes in natural environments. Nature Reviews Microbiology, 8, 251–259. https://doi.org/10.1038/nrmicro2312
- Aslam, B., Wang, W., Arshad, M. I., Khurshid, M., Muzammil, S., Rasool, M. H., Nisar, M. A., Alvi, R. F., Aslam, M. A., Qamar, M. U., Salamat, M. K. F., & Baloch, Z. (2018). Antibiotic resistance: A rundown of a global crisis. Infection and Drug Resistance, 11, 1645–1658. https://doi.org/10.2147/IDR.S173867
- Baral, D., Dvorak, B. I., Admiraal, D., Jia, S. G., Zhang, C., & Li, X. (2018). Tracking the sources of antibiotic resistance genes in an urban stream during wet weather using shotgun metagenomic analyses. Environmental Science & Technology, 52, 9033–9044. https://doi.org/10.1021/acs.est.8b01219
- Bauer, H., Fuerhacker, M., Zibuschka, F., Schmid, H., & Puxbaum, H. (2002). Bacteria and fungi in aerosols generated by two different types of wastewater treatment plants. Water Research, 36, 3965–3970. https://doi.org/10.1016/s0043-1354(02)00121-5
- Berendonk, T. U., Manaia, C. M., Merlin, C., Fatta-Kassinos, D., Cytryn, E., Walsh, F., Bürgmann, H., Sørum, H., Norström, M., Pons, M. N., Kreuzinger, N., Huovinen, P., Stefani, S., Schwartz, T., Kisand, V., Baquero, F., & Martinez, J. L. (2015). Tackling antibiotic resistance: The environmental framework. Nature Reviews Microbiology, 13, 310–317. https://doi.org/10.1038/nrmicro3439
- Berglund, B. (2015). Environmental dissemination of antibiotic resistance genes and correlation to anthropogenic contamination with antibiotics. Infection Ecology & Epidemiology, 5, 28564. https://doi.org/10.3402/iee.v5.28564
- Booton, R. D., Meeyai, A., Alhusein, N., Buller, H., Feil, E., Lambert, H., Mongkolsuk, S., Pitchforth, E., Reyher, K. K., Sakcamduang, W., Satayavivad, J., Singer, A. C., Sringernyuang, L., Thamlikitkul, V., Vass, L., Avison, M. B., Turner, K. M. E., & OH-DART Study Group. (2021). One Health drivers of antibacterial resistance: Quantifying the relative impacts of human, animal and environmental use and transmission. One Health, 12, 100220. https://doi.org/10.1016/j.onehlt.2021.100220
- Borgia, S., Lastovetska, O., Richardson, D., Eshaghi, A., Xiong, J., Chung, C., Baqi, M., McGeer, A., Ricci, G., Sawicki, R., Pantelidis, R., Low, D. E., Patel, S. N., & Melano, R. G. (2012). Outbreak of carbapenem-resistant enterobacteriaceae containing blaNDM-1, Ontario, Canada. Clinical Infectious Diseases, 55, e109–e117. https://doi.org/10.1093/cid/cis737
- Brandi, G., Sisti, M., & Amagliani, G. (2000). Evaluation of the environmental impact of microbial aerosols generated by wastewater treatment plants utilizing different aeration systems. Journal of Applied Microbiology, 88, 845–852. https://doi.org/10.1046/j.1365-2672.2000.01024.x
- Burnett, R., Chen, H., Szyszkowicz, M., Fann, N., Hubbell, B., Pope, C. A., Apte, J. S., Brauer, M., Cohen, A., Weichenthal, S., Coggins, J., Di, Q., Brunekreef, B., Frostad, J., Lim, S. S., Kan, H. D., Walker, K. D., Thurston, G. D., Hayes, R. B., … Spadaro, J. V. (2018). Global estimates of mortality associated with long-term exposure to outdoor fine particulate matter. Proceedings of the National Academy of Sciences of the United States of America, 115, 9592–9597. https://doi.org/10.1073/pnas.1803222115
- Cáliz, J., Triadó-Margarit, X., Camarero, L., & Casamayor, E. O. (2018). A long-term survey unveils strong seasonal patterns in the airborne microbiome coupled to general and regional atmospheric circulations. Proceedings of the National Academy of Sciences of the United States of America, 115, 12229–12234. https://doi.org/10.1073/pnas.1812826115
- Cao, C., Jiang, W. J., Wang, B. Y., Fang, J. H., Lang, J. D., Tian, G., Jiang, J. K., & Zhu, T. F. (2014). Inhalable microorganisms in Beijing’s PM2.5 and PM10 pollutants during a severe smog event. Environmental Science & Technology, 48, 1499–1507. https://doi.org/10.1021/es4048472
- Chapin, A., Rule, A., Gibson, K., Buckley, T., & Schwab, K. (2005). Airborne multidrug-resistant bacteria isolated from a concentrated swine feeding operation. Environmental Health Perspectives, 113, 137–142. https://doi.org/10.1289/ehp.7473
- Che, Y., Yang, Y., Xu, X., Břinda, K., Polz, M. F., Hanage, W. P., & Zhang, T. (2021). Conjugative plasmids interact with insertion sequences to shape the horizontal transfer of antimicrobial resistance genes. Proceedings of the National Academy of Sciences, 118, e2008731118. https://doi.org/10.1073/pnas.2008731118
- Chen, B. W., Yang, Y., Liang, X. M., Yu, K., Zhang, T., & Li, X. D. (2013). Metagenomic profiles of antibiotic resistance genes (ARGs) between human impacted estuary and deep ocean sediments. Environmental Science & Technology, 47, 12753–12760. https://doi.org/10.1021/es403818e
- Chen, B. W., Yuan, K., Chen, X., Yang, Y., Zhang, T., Wang, Y. W., Luan, T. G., Zou, S. C., & Li, X. D. (2016). Metagenomic analysis revealing antibiotic resistance genes (ARGs) and their genetic compartments in the Tibetan environment. Environmental Science & Technology, 50, 6670–6679. https://doi.org/10.1021/acs.est.6b00619
- Collignon, P., Beggs, J. J., Walsh, T. R., Gandra, S., & Laxminarayan, R. (2018). Anthropological and socioeconomic factors contributing to global antimicrobial resistance: A univariate and multivariable analysis. The Lancet Planetary Health, 2, e398–e405. https://doi.org/10.1016/S2542-5196(18)30186-4
- Dickson, R. P., & Huffnagle, G. B. (2015). The lung microbiome: New principles for respiratory bacteriology in health and disease. PLoS Pathogens, 11, e1004923. https://doi.org/10.1371/journal.ppat.1004923
- Duan, Y. T., Gao, H. H., Zheng, L. Y., Liu, S. Q., Cao, Y., Zhu, S. Y., Wu, Z. Z., Ren, H. Q., Mao, D. Q., & Luo, Y. (2020). Antibiotic resistance and virulence of extraintestinal pathogenic Escherichia coli (ExPEC) vary according to molecular types. Frontiers in Microbiology, 11, 598305. https://doi.org/10.3389/fmicb.2020.598305
- Ebmeyer, S., Kristiansson, E., & Larsson, D. G. J. (2021). A framework for identifying the recent origins of mobile antibiotic resistance genes. Communications Biology, 4, 8. https://doi.org/10.1038/s42003-020-01545-5
- Eduard, W., Douwes, J., Mehl, R., Heederik, D., & Melbostad, E. (2001). Short term exposure to airborne microbial agents during farm work: Exposure-response relations with eye and respiratory symptoms. Occupational and Environmental Medicine, 58, 113–118. https://doi.org/10.1136/oem.58.2.113
- Fathi, S., Hajizadeh, Y., Nikaeen, M., & Gorbani, M. (2017). Assessment of microbial aerosol emissions in an urban wastewater treatment plant operated with activated sludge process. Aerobiologia, 33, 507–515. https://doi.org/10.1007/s10453-017-9486-2
- Fernandez, M. O., Thomas, R. J., Oswin, H., Haddrell, A. E., & Reid, J. P. (2020). Transformative approach to investigate the microphysical factors influencing airborne transmission of pathogens. Applied and Environmental Microbiology, 86, e01543-20. https://doi.org/10.1128/AEM.01543-20
- Frost, L. S., Leplae, R., Summers, A. O., & Toussaint, A. (2005). Mobile genetic elements: The agents of open source evolution. Nature Reviews Microbiology, 3, 722–732. https://doi.org/10.1038/nrmicro1235
- Gandolfi, I., Franzetti, A., Bertolini, V., Gaspari, E., & Bestetti, G. (2011). Antibiotic resistance in bacteria associated with coarse atmospheric particulate matter in an urban area. Journal of Applied Microbiology, 110, 1612–1620. https://doi.org/10.1111/j.1365-2672.2011.05018.x
- Gao, X. L., Shao, M. F., Luo, Y., Dong, Y. F., Ouyang, F., Dong, W. Y., & Li, J. (2016). Airborne bacterial contaminations in typical Chinese wet market with live poultry trade. Science of the Total Environment, 572, 681–687. https://doi.org/10.1016/j.scitotenv.2016.06.208
- Gat, D., Mazar, Y., Cytryn, E., & Rudich, Y. (2017). Origin-dependent variations in the atmospheric microbiome community in eastern mediterranean dust storms. Environmental Science & Technology, 51, 6709–6718. https://doi.org/10.1021/acs.est.7b00362
- Gibbs, S. G., Green, C. F., Tarwater, P. M., Mota, L. C., Mena, K. D., & Scarpino, P. V. (2006). Isolation of antibiotic-resistant bacteria from the air plume downwind of a swine confined or concentrated animal feeding operation. Environmental Health Perspectives, 114, 1032–1037. https://doi.org/10.1289/ehp.8910
- Gilbert, Y., Veillette, M., & Duchaine, C. (2010). Airborne bacteria and antibiotic resistance genes in hospital rooms. Aerobiologia, 26, 185–194. https://doi.org/10.1007/s10453-010-9155-1
- Gillings, M. R., Gaze, W. H., Pruden, A., Smalla, K., Tiedje, J. M., & Zhu, Y. G. (2015). Using the class 1 integron-integrase gene as a proxy for anthropogenic pollution. The ISME Journal, 9, 1269–1279. https://doi.org/10.1038/ismej.2014.226
- Gotkowska‐Płachta, A., Filipkowska, Z., Korzeniewska, E., Janczukowicz, W., Dixon, B., Gołaś, I., & Szwalgin, D. (2013). Airborne microorganisms emitted from wastewater treatment plant treating domestic wastewater and meat processing industry wastes. CLEAN - Soil, Air, Water, 41, 429–436. https://doi.org/10.1002/clen.201100466
- Han, I., & Yoo, K. (2020). Metagenomic profiles of antibiotic resistance genes in activated sludge, dewatered sludge and bioaerosols. Water, 12, 1516. https://doi.org/10.3390/w12061516
- Han, Y. P., Yang, K. X., Yang, T., Zhang, M. Z., & Li, L. (2019). Bioaerosols emission and exposure risk of a wastewater treatment plant with A2O treatment process. Ecotoxicology and Environmental Safety, 169, 161–168. https://doi.org/10.1016/j.ecoenv.2018.11.018
- Han, Y. P., Yang, T., Chen, T. Z., Li, L., & Liu, J. X. (2019). Characteristics of submicron aerosols produced during aeration in wastewater treatment. Science of the Total Environment, 696, 134019. https://doi.org/10.1016/j.scitotenv.2019.134019
- He, H., Zhou, P. R., Shimabuku, K. K., Fang, X. Z., Li, S., Lee, Y. H., & Dodd, M. C. (2019). Degradation and deactivation of bacterial antibiotic resistance genes during exposure to fee chlorine, monochloramine, chlorine dioxide, ozone, ultraviolet light, and hydroxyl radical. Environmental Science & Technology, 53, 2013–2026. https://doi.org/10.1021/acs.est.8b04393
- He, P., Wu, Y., Huang, W., Wu, X., Lv, J., Liu, P., Bu, L., Bai, Z., Chen, S., Feng, W., & Yang, Z. (2020). Characteristics of and variation in airborne ARGs among urban hospitals and adjacent urban and suburban communities: A metagenomic approach. Environment International, 139, 105625. https://doi.org/10.1016/j.envint.2020.105625
- He, T. T., Jin, L., & Li, X. D. (2021). On the triad of air PM pollution, pathogenic bioaerosols, and lower respiratory infection. Environmental Geochemistry and Health. https://doi.org/10.1007/s10653-021-01025-7
- He, T. T., Jin, L., Xie, J. W., Yue, S. Y., Fu, P. Q., & Li, X. D. (2021). Intracellular and extracellular antibiotic resistance genes in airborne PM2.5 for respiratory exposure in urban areas. Environmental Science & Technology Letters, 8, 128–134. https://doi.org/10.1021/acs.estlett.0c00974
- Health Effects Institute. (2020). State of global air. Special report. https://www.stateofglobalair.org
- Hernando-Amado, S., Coque, T. M., Baquero, F., & Martínez, J. L. (2019). Defining and combating antibiotic resistance from One Health and Global Health perspectives. Nature Microbiology, 4, 1432–1442. https://doi.org/10.1038/s41564-019-0503-9
- Hsiao, T.-C., Lin, A. Y.-C., Lien, W.-C., & Lin, Y.-C. (2020). Size distribution, biological characteristics and emerging contaminants of aerosols emitted from an urban wastewater treatment plant. Journal of Hazardous Materials, 388, 121809. https://doi.org/10.1016/j.jhazmat.2019.121809
- Hu, J. L., Zhao, F. Z., Zhang, X. X., Li, K., Li, C. R., Ye, L., & Li, M. (2018). Metagenomic profiling of ARGs in airborne particulate matters during a severe smog event. Science of the Total Environment, 615, 1332–1340. https://doi.org/10.1016/j.scitotenv.2017.09.222
- Huijbers, P., Blaak, H., de Jong, M. C. M., Graat, E. A. M., Vandenbroucke-Grauls, C. M. J. E., & De Roda Husman, A. M. (2015). Role of the environment in the transmission of antimicrobial resistance to humans: A review. Environmental Science & Technology, 49, 11993–12004. https://doi.org/10.1021/acs.est.5b02566
- Jin, L., Luo, X. S., Fu, P. Q., & Li, X. D. (2017). Airborne particulate matter pollution in urban China: A chemical mixture perspective from sources to impacts. National Science Review, 4, 593–610. https://doi.org/10.1093/nsr/nww079
- Jin, L., Xie, J. W., Wong, C. K. C., Chan, S. K. Y., Abbaszade, G., Schnelle-Kreis, J., Zimmermann, R., Li, J., Zhang, G., Fu, P. Q., & Li, X. D. (2019). Contributions of city-specific fine particulate matter (PM2.5) to differential in vitro oxidative stress and toxicity implications between Beijing and Guangzhou of China. Environmental Science & Technology, 53, 2881–2891. https://doi.org/10.1021/acs.est.9b00449
- Kim, D. W., & Cha, C. J. (2021). Antibiotic resistome from the One-Health perspective: Understanding and controlling antimicrobial resistance transmission. Experimental & Molecular Medicine, 53, 301–309. https://doi.org/10.1038/s12276-021-00569-z
- Knight, G. M., Costelloe, C., Deeny, S. R., Moore, L. S. P., Hopkins, S., Johnson, A. P., Robotham, J. V., & Holmes, A. H. (2018). Quantifying where human acquisition of antibiotic resistance occurs: A mathematical modelling study. BMC Medicine, 16, 137. https://doi.org/10.1186/s12916-018-1121-8
- Knights, D., Kuczynski, J., Charlson, E. S., Zaneveld, J., Mozer, M. C., Collman, R. G., Bushman, F. D., Knight, R., & Kelley, S. T. (2011). Bayesian community-wide culture-independent microbial source tracking. Nature Methods, 8, 761–763. https://doi.org/10.1038/nmeth.1650
- Kormuth, K. A., Lin, K. S., Prussin, A. J., Vejerano, E. P., Tiwari, A. J., Cox, S. S., Myerburg, M. M., Lakdawala, S. S., & Marr, L. C. (2018). Influenza virus infectivity is retained in aerosols and droplets independent of relative humidity. The Journal of Infectious Diseases, 218, 739–747. https://doi.org/10.1093/infdis/jiy221
- Kraupner, N., Hutinel, M., Schumacher, K., Gray, D. A., Genheden, M., Fick, J., Flach, C. F., & Larsson, D. G. J. (2021). Evidence for selection of multi-resistant E. coli by hospital effluent. Environment International, 150, 106436. https://doi.org/10.1016/j.envint.2021.106436
- Kwon, H. S., Ryu, M. H., & Carlsten, C. (2020). Ultrafine particles: Unique physicochemical properties relevant to health and disease. Experimental & Molecular Medicine, 52, 318–328. https://doi.org/10.1038/s12276-020-0405-1
- Larsson, D. J., Andremont, A., Bengtsson-Palme, J., Brandt, K. K., de Roda Husman, A. M., Fagerstedt, P., Fick, J., Flach, C. F., Gaze, W. H., Kuroda, M., Kvint, K., Laxminarayan, R., Manaia, C. M., Nielsen, K. M., Plant, L., Ploy, M. C., Segovia, C., Simonet, P., Smalla, K., … Wernersson, A. S. (2018). Critical knowledge gaps and research needs related to the environmental dimensions of antibiotic resistance. Environment International, 117, 132–138. https://doi.org/10.1016/j.envint.2018.04.041
- Laxminarayan, R., Matsoso, P., Pant, S., Brower, C., Røttingen, J. A., Klugman, K., & Davies, S. (2016). Access to effective antimicrobials: A worldwide challenge. The Lancet, 387, 168–175. https://doi.org/10.1016/S0140-6736(15)00474-2
- Li, B., Yang, Y., Ma, L. P., Ju, F., Guo, F., Tiedje, J. M., & Zhang, T. (2015). Metagenomic and network analysis reveal wide distribution and co-occurrence of environmental antibiotic resistance genes. The ISME Journal, 9, 2490–2502. https://doi.org/10.1038/ismej.2015.59
- Li, J., Cao, J. J., Zhu, Y. G., Chen, Q. L., Shen, F. X., Wu, Y., Xu, S. Y., Fan, H. Q., Da, G., Huang, R. J., Wang, J., de Jesus, A. L., Morawska, L., Chan, C. K., Peccia, J., & Yao, M. S. (2018). Global survey of antibiotic resistance genes in air. Environmental Science & Technology, 52, 10975–10984. https://doi.org/10.1021/acs.est.8b02204
- Li, J., Zhou, L. T., Zhang, X. Y., Xu, C. J., Dong, L. M., & Yao, M. S. (2016). Bioaerosol emissions and detection of airborne antibiotic resistance genes from a wastewater treatment plant. Atmospheric Environment, 124, 404–412. https://doi.org/10.1016/j.atmosenv.2015.06.030
- Li, J. L., Hu, Y. R., Liu, L. J., Wang, Q., Zeng, J. H., & Chen, C. S. (2020). PM2.5 exposure perturbs lung microbiome and its metabolic profile in mice. Science of the Total Environment, 721, 137432. https://doi.org/10.1016/j.scitotenv.2020.137432
- Li, J. Y., Bi, Z. W., Ma, S. Z., Chen, B. L., Cai, C., He, J. J., Schwarz, S., Sun, C. T., Zhou, Y. Q., Yin, J., Hulth, A., Wang, Y. Q., Shen, Z. Q., Wang, S. L., Wu, C. M., Nilsson, L. E., Walsh, T. R., Börjesson, S., Shen, J. Z., Sun, Q., & Wang, Y. (2019). Inter-host transmission of carbapenemase-producing Escherichia coli among humans and backyard animals. Environmental Health Perspectives, 127, 107009. https://doi.org/10.1289/EHP5251
- Li, L., Gao, M., & Liu, J. X. (2011). Distribution characterization of microbial aerosols emitted from a wastewater treatment plant using the Orbal oxidation ditch process. Process Biochemistry, 46, 910–915. https://doi.org/10.1016/j.procbio.2010.12.016
- Li, L. G., Yin, X., & Zhang, T. (2018). Tracking antibiotic resistance gene pollution from different sources using machine-learning classification. Microbiome, 6, 93. https://doi.org/10.1186/s40168-018-0480-x
- Li, L. Y., Wang, Q., Bi, W., Hou, J., Xue, Y. G., Mao, D. Q., Das, R., Luo, Y., & Li, X. D. (2020). Municipal solid waste treatment system increases ambient airborne bacteria and antibiotic resistance genes. Environmental Science & Technology, 54, 3900–3908. https://doi.org/10.1021/acs.est.9b07641
- Li, X. D., Jin, L., & Kan, H. (2019). Air pollution: A global problem needs local fixes. Nature, 570, 437–439. https://doi.org/10.1038/d41586-019-01960-7
- Li, Y. P., Zhang, H. F., Qiu, X. H., Zhang, Y. R., & Wang, H. R. (2013). Dispersion and risk assessment of bacterial aerosols emitted from rotating-brush aerator during summer in a wastewater treatment plant of Xi’an. Aerosol and Air Quality Research, 13, 1807–1814. https://doi.org/10.4209/aaqr.2012.09.0245
- Liang, Z. S., Yu, Y., Ye, Z. K., Li, G. Y., Wang, W. J., & An, T. C. (2020). Pollution profiles of antibiotic resistance genes associated with airborne opportunistic pathogens from typical area, Pearl River Estuary and their exposure risk to human. Environment International, 143, 105934. https://doi.org/10.1016/j.envint.2020.105934
- Lin, D. C., Chen, K. C., Guo, J. B., Ye, L. W., Li, R. C., Chan, E. W. C., & Chen, S. (2020). Contribution of biofilm formation genetic locus, pgaABCD, to antibiotic resistance development in gut microbiome. Gut Microbes, 12, 1–12. https://doi.org/10.1080/19490976.2020.1842992
- Lin, H. L., Tao, J., Kan, H. D., Qian, Z. M., Chen, A. L., Du, Y. D., Liu, T., Zhang, Y. H., Qi, Y. Q., Ye, J. J., Li, S. M., Li, W. L., Xiao, J. P., Zeng, W. L., Li, X., Stamatakis, K. A., Chen, X. Y., & Ma, W. J. (2018). Ambient particulate matter air pollution associated with acute respiratory distress syndrome in Guangzhou, China. Journal of Exposure Science & Environmental Epidemiology, 28, 392–399. https://doi.org/10.1038/s41370-018-0034-0
- Lin, K. S., & Marr, L. C. (2020). Humidity-dependent decay of viruses, but not bacteria, in aerosols and droplets follows disinfection kinetics. Environmental Science & Technology, 54, 1024–1032. https://doi.org/10.1021/acs.est.9b04959
- Ling, A. L., Pace, N. R., Hernandez, M. T., & LaPara, T. M. (2013). Tetracycline resistance and Class 1 integron genes associated with indoor and outdoor aerosols. Environmental Science & Technology, 47, 4046–4052. https://doi.org/10.1021/es400238g
- Mackenzie, J. S., & Jeggo, M. (2019). The One Health approach—Why is it so important? Tropical Medicine and Infectious Disease, 4, 88. https://doi.org/10.3390/tropicalmed4020088
- Manaia, C. M. (2017). Assessing the risk of antibiotic resistance transmission from the environment to humans: Non-direct proportionality between abundance and risk. Trends in Microbiology, 25, 173–181. https://doi.org/10.1016/j.tim.2016.11.014
- Mao, Y. X., Ding, P., Wang, Y. B., Ding, C., Wu, L. P., Zheng, P., Zhang, X., Li, X., Wang, L. Y., & Sun, Z. K. (2019). Comparison of culturable antibiotic-resistant bacteria in polluted and non-polluted air in Beijing. Environment International, 131, 104936. https://doi.org/10.1016/j.envint.2019.104936
- Martinez, J. L. (2012). Bottlenecks in the transferability of antibiotic resistance from natural ecosystems to human bacterial pathogens. Frontiers in Microbiology, 2, 265. https://doi.org/10.3389/fmicb.2011.00265
- Martinez, J. L., & Baquero, F. (2000). Mutation frequencies and antibiotic resistance. Antimicrobial Agents and Chemotherapy, 44, 1771–1777. https://doi.org/10.1128/AAC.44.7.1771-1777.2000
- Mazar, Y., Cytryn, E., Erel, Y., & Rudich, Y. (2016). Effect of dust storms on the atmospheric microbiome in the Eastern Mediterranean. Environmental Science & Technology, 50, 4194–4202. https://doi.org/10.1021/acs.est.5b06348
- McEachran, A. D., Blackwell, B. R., Hanson, J. D., Wooten, K. J., Mayer, G. D., Cox, S. B., & Smith, P. N. (2015). Antibiotics, bacteria, and antibiotic resistance genes: Aerial transport from cattle feed yards via particulate matter. Environmental Health Perspectives, 123, 337–343. https://doi.org/10.1289/ehp.1408555
- Melbostad, E., Eduard, W., Skogstad, A., Sandven, P., Lassen, J., Søstrand, P., & Heldal, K. (1994). Exposure to bacterial aerosols and work-related symptoms in sewage workers . American Journal of Industrial Medicine, 25, 59–63. https://doi.org/10.1002/ajim.4700250116
- Meng, X., Ma, Y. J., Chen, R. J., Zhou, Z. J., Chen, B. H., & Kan, H. D. (2013). Size-fractionated particle number concentrations and daily mortality in a Chinese city. Environmental Health Perspectives, 121, 1174–1178. https://doi.org/10.1289/ehp.1206398
- Merlin, C. (2020). Reducing the consumption of antibiotics: Would that be enough to slow down the dissemination of resistances in the downstream environment? Frontiers in Microbiology, 11, 33. https://doi.org/10.3389/fmicb.2020.00033
- Mokoena, K. K., Ethan, C. J., Yu, Y., Shale, K., & Liu, F. (2019). Ambient air pollution and respiratory mortality in Xi’an, China: A time-series analysis. Respiratory Research, 20, 139. https://doi.org/10.1186/s12931-019-1117-8
- Molton, J. S., Tambyah, P. A., Ang, B. S., Ling, M. L., & Fisher, D. A. (2013). The global spread of healthcare-associated multidrug-resistant bacteria: A perspective from Asia. Clinical Infectious Diseases, 56, 1310–1318. https://doi.org/10.1093/cid/c20
- Murray, A. K., Stanton, I. C., Wright, J., Zhang, L. H., Snape, J., & Gaze, W. H. (2020). The ‘SELection End points in Communities of bacTeria’ (SELECT) method: A novel experimental assay to facilitate risk assessment of selection for antimicrobial resistance in the environment. Environmental Health Perspectives, 128, 107007. https://doi.org/10.1289/EHP6635
- Murray, R., Tien, Y. C., Scott, A., & Topp, E. (2019). The impact of municipal sewage sludge stabilization processes on the abundance, field persistence, and transmission of antibiotic resistant bacteria and antibiotic resistance genes to vegetables at harvest. Science of the Total Environment, 651, 1680–1687. https://doi.org/10.1016/j.scitotenv.2018.10.030
- Mushtaq, N., Ezzati, M., Hall, L., Dickson, I., Kirwan, M., Png, K. M. Y., Mudway, I. S., & Grigg, J. (2011). Adhesion of Streptococcus pneumoniae to human airway epithelial cells exposed to urban particulate matter. The Journal of Allergy and Clinical Immunology, 127, 1236–1242.e2. https://doi.org/10.1016/j.jaci.2010.11.039
- Nadimpalli, M. L., Stewart, J. R., Pierce, E., Pisanic, N., Love, D. C., Hall, D., Larsen, J., Carroll, K. C., Tekle, T., Perl, T. M., & Heaney, C. D. (2018). Face mask use and persistence of livestock-associated Staphylococcus aureus nasal carriage among industrial hog operation workers and Household contacts, USA. Environmental Health Perspectives, 126, 127005. https://doi.org/10.1289/EHP3453
- Nihemaiti, M., Yoon, Y., He, H., Dodd, M. C., Croue, J. P., & Lee, Y. (2020). Degradation and deactivation of a plasmid-encoded extracellular antibiotic resistance gene during separate and combined exposures to UV254 and radicals. Water Research, 182, 115921. https://doi.org/10.1016/j.watres.2020.115921
- Niu, Y., Chen, R. J., Wang, C. P., Wang, W. D., Jiang, J., Wu, W. D., Cai, J., Zhao, Z. H., Xu, X. H., & Kan, H. D. (2020). Ozone exposure leads to changes in airway permeability, microbiota and metabolome: A randomised, double-blind, crossover trial. European Respiratory Journal, 2020, 56. https://doi.org/10.1183/13993003.00165-2020
- O’Neill, J. (2016). Tackling drug-resistant infections globally: Final report and recommendations. Review on Antimicrobial Resistance.
- Pal, C., Bengtsson-Palme, J., Kristiansson, E., & Larsson, D. G. J. (2016). The structure and diversity of human, animal and environmental resistomes. Microbiome, 4, 54. https://doi.org/10.1186/s40168-016-0199-5
- Pruden, A., Pei, R., Storteboom, H., & Carlson, K. H. (2006). Antibiotic resistance genes as emerging contaminants: Studies in Northern Colorado. Environmental Science & Technology, 40, 7445–7450. https://doi.org/10.1021/es060413l
- Psoter, K. J., De Roos, A. J., Wakefield, J., Mayer, J. D., & Rosenfeld, M. (2017). Air pollution exposure is associated with MRSA acquisition in young U.S. children with cystic fibrosis. BMC Pulmonary Medicine, 17, 106. https://doi.org/10.1186/s12890-017-0449-8
- Rodo, X., Curcoll, R., Robinson, M., Ballester, J., Burns, J. C., Cayan, D. R., Lipkin, W. I., Williams, B. L., Couto-Rodriguez, M., Nakamura, Y., Uehara, R., Tanimoto, H., & Morgui, J.-A. (2014). Tropospheric winds from northeastern China carry the etiologic agent of Kawasaki disease from its source to Japan. Proceedings of the National Academy of Sciences of the United States of America, 111, 7952–7957. https://doi.org/10.1073/pnas.1400380111
- Scott, D. M., & Lesher, E. (1963). Effect of ozone on survival and permeability of Escherichia coli. Journal of Bacteriology, 85, 567–576. https://doi.org/10.1128/jb.85.3.567-576.1963
- Seurat, E., Verdin, A., Cazier, F., Courcot, D., Fitoussi, R., Vié, K., Desauziers, V., Momas, I., Seta, N., & Achard, S. (2021). Influence of the environmental relative humidity on the inflammatory response of skin model after exposure to various environmental pollutants. Environmental Research, 196, 110350. https://doi.org/10.1016/j.envres.2020.110350
- Shen, Y. B., Zhou, H. W., Xu, J., Wang, Y. Q., Zhang, Q. J., Walsh, T. R., Shao, B., Wu, C. M., Hu, Y. Y., Yang, L., Shen, Z. Q., Wu, Z. W., Sun, Q. L., Ou, Y. N., Wang, Y. L., Wang, S. L., Wu, Y. N., Cai, C., Li, J., Shen, J. Z., … Wang, Y. (2018). Anthropogenic and environmental factors associated with high incidence of mcr-1 carriage in humans across China. Nature Microbiology, 3, 1054–1062. https://doi.org/10.1038/s41564-018-0205-8
- Shuyler, L. R. (1973). National animal feedlot waste research program. E.P.A.-R2-73-157 Environmental Protection Agency.
- Smalla, K., Cook, K., Djordjevic, S. P., Klümper, U., & Gillings, M. (2018). Environmental dimensions of antibiotic resistance: Assessment of basic science gaps. FEMS Microbiology Ecology, 94, fiy195. https://doi.org/10.1093/femsec/fiy195
- Smets, W., Moretti, S., Denys, S., & Lebeer, S. (2016). Airborne bacteria in the atmosphere: Presence, purpose, and potential. Atmospheric Environment, 139, 214–221. https://doi.org/10.1016/j.atmosenv.2016.05.038
- Smith, D. J., Jaffe, D. A., Birmele, M. N., Griffin, D. W., Schuerger, A. C., Hee, J., & Roberts, M. S. (2012). Free tropospheric transport of microorganisms from Asia to North America. Microbial Ecology, 64, 973–985. https://doi.org/10.1007/s00248-012-0088-9
- Song, T. L., Wang, S., Zhang, Y. Y., Song, J. W., Liu, F. B., Fu, P. Q., Shiraiwa, M., Xie, Z. Y., Yue, D. L., Zhong, L. J., Zheng, J. Y., & Lai, S. C. (2017). Proteins and amino acids in fine particulate matter in rural Guangzhou, southern China: Seasonal cycles, sources, and atmospheric processes. Environmental Science & Technology, 51, 6773–6781. https://doi.org/10.1021/acs.est.7b00987
- Surette, M. D., & Wright, G. D. (2017). Lessons from the environmental antibiotic resistome. Annual Review of Microbiology, 71, 309–329. https://doi.org/10.1146/annurev-micro-090816-093420
- Sun, X. Z., Li, D. M., Li, B., Sun, S. J., Yabo, S. D., Geng, J. L., Ma, L. X., & Qi, H. (2020). Exploring the disparity of inhalable bacterial communities and antibiotic resistance genes between hazy days and non-hazy days in a cold megacity in Northeast China. Journal of Hazardous Materials, 398, 122984. https://doi.org/10.1016/j.jhazmat.2020.122984
- Thanomsub, B., Anupunpisit, V., Chanphetch, S., Watcharachaipong, T., Poonkhum, R., & Srisukonth, C. (2002). Effects of ozone treatment on cell growth and ultrastructural changes in bacteria. The Journal of General and Applied Microbiology, 48, 193–199. https://doi.org/10.2323/jgam.48.193
- Tiwari, A. J., Fields, C. G., & Marr, L. C. (2013). A cost-effective method of aerosolizing dry powdered nanoparticles. Aerosol Science and Technology, 47, 1267–1275. https://doi.org/10.1080/02786826.2013.834292
- Udikovic-Kolic, N., Wichmann, F., Broderick, N. A., & Handelsman, J. (2014). Bloom of resident antibiotic-resistant bacteria in soil following manure fertilization. Proceedings of the National Academy of Sciences of the United States of America, 111, 15202–15207. https://doi.org/10.1073/pnas.1409836111
- UNEP (2017). Frontiers 2017 Emerging Issues of Environmental Concern. United Nations Environment Programme, Nairobi.
- van Hoek, A. H. A. M., Mevius, D., Guerra, B., Mullany, P., Roberts, A. P., & Aarts, H. J. M. (2011). Acquired antibiotic resistance genes: An overview. Frontiers in Microbiology, 2, 203. https://doi.org/10.3389/fmicb.2011.00203
- Vikesland, P. J., Pruden, A., Alvarez, P. J. J., Aga, D., Bürgmann, H., Li, X. D., Manaia, C. M., Nambi, I., Wigginton, K., Zhang, T., & Zhu, Y. G. (2017). Toward a comprehensive strategy to mitigate dissemination of environmental sources of antibiotic resistance. Environmental Science & Technology, 51, 13061–13069. https://doi.org/10.1021/acs.est.7b03623
- Wang, R. N., Li, X., & Yan, C. (2021). Seasonal fluctuation of aerosolization ratio of bioaerosols and quantitative microbial risk assessment in a wastewater treatment plant. Environmental Science and Pollution Research. https://doi.org/10.1007/s11356-021-15462-5
- Wang, S., Song, T., Shiraiwa, M., Song, J., Ren, H., Ren, L., Wei, L., Sun, Y., Zhang, Y., Fu, P., & Lai, S. (2019). Occurrence of aerosol proteinaceous matter in urban Beijing: An investigation on composition, sources, and atmospheric processes during the “APEC Blue” period. Environmental Science & Technology, 53, 7380–7390. https://doi.org/10.1021/acs.est.9b00726
- Wang, Y., Lu, J., Engelstädter, J., Zhang, S., Ding, P. B., Mao, L. K., Yuan, Z. G., Bond, P. L., & Guo, J. H. (2020). Non-antibiotic pharmaceuticals enhance the transmission of exogenous antibiotic resistance genes through bacterial transformation. The ISME Journal, 14, 2179–2196. https://doi.org/10.1038/s41396-020-0679-2
- Wang, Y. Z., Wang, C., & Song, L. (2019). Distribution of antibiotic resistance genes and bacteria from six atmospheric environments: Exposure risk to human. Science of the Total Environment, 694, 133750. https://doi.org/10.1016/j.scitotenv.2019.133750
- Wardyn, S. E., Stegger, M., Price, L. B., & Smith, T. C. (2018). Whole-genome analysis of recurrent Staphylococcus aureus t571/ST398 infection in farmer, Iowa, USA. Emerging Infectious Diseases, 24, 153–154. https://doi.org/10.3201/eid2401.161184
- Wei, K., Zou, Z. L., Zheng, Y. H., Li, J., Shen, F. X., Wu, C. Y., Wu, Y. S., Hu, M., & Yao, M. (2016). Ambient bioaerosol particle dynamics observed during haze and sunny days in Beijing. Science of the Total Environment, 550, 751–759. https://doi.org/10.1016/j.scitotenv.2016.01.137
- Wengenroth, L., Berglund, F., Blaak, H., Chifiriuc, M. C., Flach, C. F., Pircalabioru, G. G., Larsson, D. G. J., Marutescu, L., Passel, M. W. J., van Passel, M. W. J., Popa, M., Radon, K., de Roda Husman, A. M., Rodríguez-Molina, D., Weinmann, T., Wieser, A., & Schmitt, H. (2021). Antibiotic resistance in wastewater treatment plants and transmission risks for employees and residents: The concept of the AWARE study. Antibiotics, 10, 478. https://doi.org/10.3390/antibiotics10050478
- Winglee, K., Howard, A. G., Sha, W., Gharaibeh, R. Z., Liu, J. W., Jin, D. H., Fodor, A. A., & Gordon-Larsen, P. (2017). Recent urbanization in China is correlated with a Westernized microbiome encoding increased virulence and antibiotic resistance genes. Microbiome, 5, 121. https://doi.org/10.1186/s40168-017-0338-7
- Wu, B. G., Kapoor, B., Cummings, K. J., Stanton, M. L., Nett, R. J., Kreiss, K., Abraham, J. L., Colby, T. V., Franko, A. D., Green, F. H. Y., Sanyal, S., Clemente, J. C., Gao, Z., Coffre, M., Meyn, P., Heguy, A., Li, Y., Sulaiman, I., Borbet, T. C., … Segal, L. N. (2020). Evidence for environmental-human microbiota transfer at a manufacturing facility with novel work-related respiratory disease. American Journal of Respiratory and Critical Care Medicine, 202, 1678–1688. https://doi.org/10.1164/rccm.202001-0197OC
- Wu, D., Huang, X. H., Sun, J. Z., Graham, D. W., & Xie, B. (2017). Antibiotic resistance genes and associated microbial community conditions in aging landfill systems. Environmental Science & Technology, 51, 12859–12867. https://doi.org/10.1021/acs.est.7b03797
- Xie, J. W., Jin, L., He, T. T., Chen, B. W., Luo, X. S., Feng, B., Huang, W., Li, J., Fu, P. Q., & Li, X. D. (2019). Bacteria and antibiotic resistance genes (ARGs) in PM2.5 from China: Implications for human exposure. Environmental Science & Technology, 53, 963–972. https://doi.org/10.1021/acs.est.8b04630
- Xie, J. W., Jin, L., Luo, X. S., Zhao, Z., & Li, X. D. (2018). Seasonal disparities in airborne bacteria and associated antibiotic resistance genes in PM2.5 between urban and rural sites. Environmental Science & Technology Letters, 5, 74–79. https://doi.org/10.1021/acs.estlett.7b00561
- Xie, S. S., Gu, A. Z., Cen, T. Y., Li, D., & Chen, J. M. (2019). The effect and mechanism of urban fine particulate matter (PM2.5) on horizontal transfer of plasmid-mediated antimicrobial resistance genes. Science of the Total Environment, 683, 116–123. https://doi.org/10.1016/j.scitotenv.2019.05.115
- Xu, H., Chen, Z. Y., Huang, R. Y., Cui, Y. X., Li, Q., Zhao, Y. H., Wang, X. L., Mao, D. Q., Luo, Y., & Ren, H. Q. (2021). Antibiotic resistance gene-carrying plasmid spreads into the plant endophytic bacteria using soil bacteria as carriers. Environmental Science & Technology, 55, 10462–10470. https://doi.org/10.1021/acs.est.1c01615
- Xu, Y., Xiao, H., Wu, D., & Long, C. (2020). Abiotic and biological degradation of atmospheric proteinaceous matter can contribute significantly to dissolved amino acids in wet deposition. Environmental Science & Technology, 54, 6551–6561. https://doi.org/10.1021/acs.est.0c00421
- Yang, K., Li, L., Wang, Y., Xue, S., Han, Y., & Liu, J. (2019). Airborne bacteria in a wastewater treatment plant: Emission characterization, source analysis and health risk assessment. Water Research, 149, 596–606. https://doi.org/10.1016/j.watres.2018.11.027
- Yang, Y., Zhou, R. J., Chen, B. W., Zhang, T., Hu, L. G., & Zou, S. C. (2018). Characterization of airborne antibiotic resistance genes from typical bioaerosol emission sources in the urban environment using metagenomic approach. Chemosphere, 213, 463–471. https://doi.org/10.1016/j.chemosphere.2018.09.066
- Zhang, L. D., Forst, C. V., Gordon, A., Gussin, G., Geber, A. B., Fernandez, P. J., Ding, T., Lashua, L., Wang, M. H., Balmaseda, A., Bonneau, R., Zhang, B., & Ghedin, E. (2020). Characterization of antibiotic resistance and host-microbiome interactions in the human upper respiratory tract during influenza infection. Microbiome, 8, 39. https://doi.org/10.1186/s40168-020-00803-2
- Zhang, M. Y., Zuo, J. N., Yu, X., Shi, X. C., Chen, L., & Li, Z. X. (2018). Quantification of multi-antibiotic resistant opportunistic pathogenic bacteria in bioaerosols in and around a pharmaceutical wastewater treatment plant. Journal of Environmental Sciences, 72, 53–63. https://doi.org/10.1016/j.jes.2017.12.011
- Zhang, T., Li, X. Y., Wang, M. F., Chen, H. X., Yang, Y., Chen, Q. L., & Yao, M. S. (2019). Time-resolved spread of antibiotic resistance genes in highly polluted air. Environment International, 127, 333–339. https://doi.org/10.1016/j.envint.2019.03.006
- Zhang, Y., Gu, A. Z., Cen, T. Y., Li, X. Y., Li, D., & Chen, J. M. (2018). Petrol and diesel exhaust particles accelerate the horizontal transfer of plasmid-mediated antimicrobial resistance genes. Environment International, 114, 280–287. https://doi.org/10.1016/j.envint.2018.02.038
- Zhao, Y. H., Chen, Z. Y., Hou, J., Mao, D. Q., Lin, H., Xue, Y. G., & Luo, Y. (2020). Monitoring antibiotic resistomes and bacterial microbiomes in the aerosols from fine, hazy, and dusty weather in Tianjin, China using a developed high-volume tandem liquid impinging sampler. Science of the Total Environment, 731, 139242. https://doi.org/10.1016/j.scitotenv.2020.139242
- Zhen, Q., Deng, Y., Wang, Y. Q., Wang, X. K., Zhang, H. X., Sun, X., & Ouyang, Z. Y. (2017). Meteorological factors had more impact on airborne bacterial communities than air pollutants. Science of the Total Environment, 601, 703–712. https://doi.org/10.1016/j.scitotenv.2017.05.049
- Zhou, Z. C., Shuai, X. Y., Lin, Z. J., Liu, Y., Zhu, L., & Chen, H. (2021). Prevalence of multi-resistant plasmids in hospital inhalable particulate matter (PM) and its impact on horizontal gene transfer. Environmental Pollution, 270, 116296. https://doi.org/10.1016/j.envpol.2020.116296
- Zhu, G. B., Wang, X. M., Yang, T., Su, J. Q., Qin, Y., Wang, S. Y., Gillings, M., Wang, C., Ju, F., Lan, B. R., Liu, C. L., Li, H., Long, X. E., Wang, X. M., Jetten, M. S. M., Wang, Z. F., & Zhu, Y. G. (2021). Air pollution could drive global dissemination of antibiotic resistance genes. The ISME Journal, 15, 270–281. https://doi.org/10.1038/s41396-020-00780-2
- Zhu, Y. G., Johnson, T. A., Su, J. Q., Qiao, M., Guo, G. X., Stedtfeld, R. D., Hashsham, S. A., & Tiedje, J. M. (2013). Diverse and abundant antibiotic resistance genes in Chinese swine farms. Proceedings of the National Academy of Sciences of the United States of America, 110, 3435–3440. https://doi.org/10.1073/pnas.1222743110