Abstract
Biological remediation including bioremediation and phytoremediation has been used to remediate contaminated sites due to their cost-effectiveness, energy conservation, and environmental friendliness. There are 24 articles in Critical Reviews in Environmental Science and Technology (CREST) from 2018 to 2022 focusing on this topic. Bioremediation employs microorganisms to modify and/or degrade contaminants, while phytoremediation uses plants to remove, contain, and/or change contaminants. They cover different contaminants including traditional (nutrients, petroleum hydrocarbons, organo-pesticides, plastics, and heavy metals such as Cd, Cr, Pb and U) and emerging contaminants (pharmaceutical and personal care products, and perfluoroalkyl and polyfluoroalkyl substances). However, these remediation technologies have limitations in applications including lengthy periods, environmental sensitivity, nutrient deficiency and contaminant toxicity. Therefore, improvement strategies including immobilization of microbial/microalgal cells, construction of microbial/microalgal consortia, and use of amendments have been employed to overcome its drawbacks. Further studies should focus on cost-effective strategies to enhance the efficiency of biological remediation and their environmental adaptability, especially under extreme environments and with emerging contaminants. Microbes, microalgae and plants with good contaminant removal capability should be coupled with engineering practices based on their interactive mechanisms among bio-association and contaminants at molecular levels.
Graphical abstract
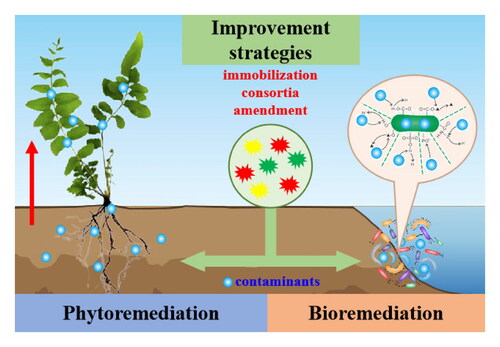
1. Introduction
Biological remediation including bioremediation and phytoremediation employs microorganisms and/or plants to remove, degrade and/or immobilize contaminants in the environment. With its advantages being low-cost, simple operation, and eco-friendly, biological remediation has wide application in restoring contaminated sites (Cui et al., Citation2022). In recent years, some successful cases have been reported, including sites in the cold climate and with co-contamination (Miri et al., Citation2019; Usmani et al., Citation2022). However, biological remediation takes time due to issues with its environmental adaptability, nutrient limitation, toxicity resistance and limited contaminant bioavailability. To overcome these limitations, improvement strategies, such as immobilization of microbial/microalgal cells (Miri et al., Citation2019; Partovinia & Rasekh, Citation2018), construction of microbial/microalgal consortia (Li et al., Citation2022; Usmani et al., Citation2022), and use of amendments (i.e., chemical agents, nanomaterials, poultry manure, industrial wastes and microbial agents) have been employed (Song et al., Citation2019; Wang et al., Citation2020).
With “bioremediation” or “phytoremediation” as the keywords, 18,001 peer-reviewed publications from 2018 to 2022 are available from “Web of Science” (), which can be grouped into 11 topical clusters based on 600 keywords. Among them, Cluster 1 (red) is the largest research theme, including biodegradation of organic contaminants and associated mechanisms; Cluster 2 (green) and Cluster 3 (blue) mainly focus on phytoremediation of inorganic contaminants heavy metals especially Cd; Cluster 4 (yellow) comprises wastewater treatment and microalgae application; Cluster 5 (purple) and Cluster 6 (baby-blue) are themed with the adsorption/immobilization of contaminants; and the remaining 5 clusters are related to remediation of metals like Cr and U, as well as oil and diesel using earthworm and microbes along with the associated mechanisms. For biological remediation, all clusters contain biotechnological improvements. In short, the research on biological remediation of contaminated sites has attracted considerable attention during the last 5 years.
Figure 1. Visualized network of 600 popular keywords of peer-reviewed publications from 2018 to 2022. The bibliographic data were retrieved from the “Web of Science Core Collection” with “bioremediation” or “phytoremediation” as keywords, with fractional counting within keyword co-occurrence analysis being performed using VOSviewer and Pajek.
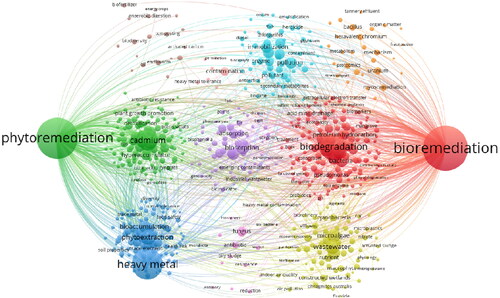
For this editorial, 24 articles on bioremediation and phytoremediation in CREST from 2018 to 2022 were collected, which are within the 11 clusters ( and ). Specifically, among them, 16 publications focus on bioremediation, and 4 on phytoremediation, with 4 on both. They cover different topics, including organic contaminant biodegradation, heavy metal removal, improvement strategies, and the interactive mechanisms between organisms and contaminants during remediation (). This editorial hopes to provide a comprehensive summary on the applications and improvements of biological remediation in different media (soils and wastewaters) and different contaminants (organic and inorganic).
2. Bioremediation of sites with organic and inorganic contaminants
Environmental contaminants include excessive nutrients, organic contaminants (e.g., petroleum hydrocarbons, organo-pesticides, plastics, pharmaceutical and personal care products), and heavy metals (e.g., Cd, Cr, Pb, and U). The microorganisms include bacteria, archaea, fungi, and microalgae.
2.1. Nutrients
Large amounts of C and N are the main contaminants in domestic wastewater. Some improved strategies, such as the integration of denitrifying anaerobic methane oxidation (DAMO) with anaerobic ammonium oxidation (Anammox), and biofilm technology, have been developed to efficiently remove these contaminants during wastewater treatment (Chen et al., Citation2022; Zhu et al., Citation2022). Chen et al. (Citation2022) proposed that the integrated strategy of DAMO-Anammox can simultaneously convert ammonium, nitrite, nitrate, and methane to harmless nitrogen and carbon dioxide, achieving an intriguingly energy-positive potential. Briefly, DAMO archaea transform nitrate to nitrite/ammonium by nitrate reductase and ammonium-producing nitrite reductase, which can be further transformed by Anammox bacteria with CO2/bicarbonate as the C source. For C metabolism, DAMO archaea/bacteria convert methane to CO2, which is in turn fixed by Anammox bacteria via the acetyl-CoA pathway to complete the C cycle (Chen et al., Citation2022).
Biofilms are effective in nitrogen removal due to their advantages of high loading capacity and efficiency as well as their abilities to retain microorganisms with a promising regeneration (Zhu et al., Citation2022). Exogenous quorum sensing bacteria can efficiently promote the formation of biofilm by adhesion, growth, maturation, and diffusion, thus improving denitrification and nitrogen removal (Zhu et al., Citation2022). Besides, they proposed that the soluble signal molecules, such as acyl-homoserine-lactones, oligopeptide autoinducers, and hydroxyl-palmitic acid methyl ester, play important roles in regulating bacterial growth, metabolism, gene transcription, extracellular polymeric substance formation, bacterial motility, and community structure (Zhu et al., Citation2022). Hence, these signal molecules may help in controlling nitrogen removal efficiency.
2.2. Petroleum hydrocarbons
Petroleum hydrocarbons consist of a complex mixture of different organic contaminants, including alkanes, cycloalkanes, aromatic hydrocarbons, and other organic contaminants. Three articles focused on their biodegradation and associated mechanisms. Miri et al. (Citation2019) summarized research progress on the bioremediation of sites contaminated by petroleum hydrocarbons under the cold climate. Furthermore, some successful cases showed the effectiveness of both aerobic and anaerobic biodegradation. While aerobic bioremediation serves as a more conventional strategy, anaerobic bioremediation has received increasing attention, with much progress being made. Harindintwali et al. (Citation2022) reviewed the syntrophy of bacteria and archaea during the anaerobic degradation of hydrocarbons, especially with the methanogenic process as its final step. They highlighted that syntrophic bacteria with different metabolic capabilities can cooperate with methanogenic archaea to biodegrade hydrocarbons. As important components of hydrocarbons, polycyclic aromatic hydrocarbons (PAHs) are priority contaminants according to USEPA because of their persistence, potential carcinogenicity, and high toxicity. During wastewater treatment and sludge disposal, dominant PAHs with low molecular weight are mainly biodegraded by microorganisms in wastewater, while the residues with high molecular weight are transferred to the sludge, which are further removed via anaerobic digestion (Zhang et al., Citation2019).
2.3. Organo-pesticides
Pesticides, including organophosphates and organochlorines, have been used worldwide in crop production over the past few decades. Although they are beneficial economically, they cause adverse impacts on the environment and human health (Briceño et al., Citation2018). Sidhu et al. (Citation2019) reviewed the toxicity of organophosphate pesticides on plants and animals in multifarious terrestrial and aquatic ecosystems, including inhibition of various enzyme activities. They also summarized the genes and enzymes involved in their degradations. Based on 337 measurements of reductive dechlorination and methanogenesis production (Cheng et al., Citation2022), accelerated methanogenesis occurs simultaneously with the accelerated reductive removal of chlorinated organic contaminants including organochlorine pesticides. The possible mechanisms related to synergistic coupling between bioreductive removal of chlorinated organic contaminants and methanogenesis were further discussed. To date, many microbes capable of degrading organic pesticides have been isolated. Among them, Streptomyces, one of the most common degraders, can efficiently degrade pesticides including diuron (S. albidoflavus), cypermethrin (Streptomyces sp. HU-S-01) and chlordane (Streptomyces sp. A5) in aquatic, soil and slurries systems (Briceño et al., Citation2018).
2.4. Plastic contaminants
Plastics are synthetic organic polymers with high-persistence and low-degradability. Nonetheless, several organisms have been used in plastic biodegradation, including bacteria Pseudomonas aeruginosa and Streptomyces badius, and fungi Aspergillus niger and Aspergillus flavus (Paço et al., Citation2019). Bioaugmentation is often used in bioremediation, which refers to the application of exogenous microbes with specific catabolic activities to contaminated sites. Coupled these functional microbes with chemical/physical pretreatments, these plastic wastes may be reduced and removed from the environment. Besides, plastic contaminants may be often associated with some organic additives, with bisphenol-S contamination having received increasing attention. Fang et al. (Citation2020) summarized some active species for bisphenol-S biodegradation and the associated degradation pathways. As the first reported strain, Phingobium fuliginis OMI is capable of complete aerobic degradation of bisphenol-S. The strain was isolated from the rhizosphere of aquatic plants, which can degrade bisphenol-S within 24 h via phenolic ring hydroxylation followed by a meta-cleavage pathway (Fang et al., Citation2020).
2.5. Pharmaceutical and personal care products (PPCPs)
In addition to traditional organic contaminants, bioremediation of emerging contaminants has attracted some attention, particularly PPCPs, which include ketoprofen, caffeine, and steroid hormones like estrone, estradiol, and estrogen. Although those anthropogenic PPCPs enter the environment at trace levels, they still pose adverse impacts on human health and ecosystems (Nguyen et al., Citation2021). Various fungal enzymes, like laccase from Trametes pubescens and lignin peroxidase from Phanerochaete chrysosporium, show the abilities to remediate PPCPs. For instance, laccase can catalytically transfer estrogens into by-products like dimers and trimers, resulting in reduced toxicity and concentration (Zdarta et al., Citation2022).
In wastewater treatment plants, the removal of pharmaceutically-active compounds by conventional activated sludge is inefficient. As such, several biological technologies including membrane reactors, enzymatic membrane reactors, and hybrid systems have been developed (Leal et al., Citation2020). In these systems, pharmaceutically-active compounds are degraded by active microbes or bioactive molecules. Similarly, at drinking-water treatment plants with biofiltration, PPCPs can be biodegraded metabolically by autotrophic bacteria (ammonia/methane oxidizing bacteria) or heterotrophic bacteria via different mechanisms and pathways (Wang et al., Citation2021). While heterotrophic bacteria take organic C as primary substrates for cell growth, autotrophic bacteria degrade contaminants via co-metabolism. For example, bacterial ammonia monooxygenase and methane monooxygenase can oxidize different PPCPs, which are supported by the fact that the removal of ketoprofen and caffeine is positively correlated with bacterial ammonia oxidizing activities.
Moreover, microalgae have been employed in the remediation of PPCPs-contaminated waters. This is because they show excellent bioremediation performance, including high efficiency, large surface area, strong binding affinity, and outstanding environmental adaptability. Usmani et al. (Citation2022) and Nguyen et al. (Citation2021) illustrated the mechanisms of contaminant removal by microalgae, including cell surface adsorption, intracellular bioaccumulation, and intra/extracellular biodegradation. In the microalgae-based treatment system, illumination is required for the photosynthesis of microalgae, with contaminants being removed by UV-induced photodegradation (Nguyen et al., Citation2021). This is because contaminants absorb light energy to initiate chemical reactions, or dissolved organic substances absorb light to produce reactive oxygen species for target contaminant degradation.
2.6. Heavy metals
Besides nutrients and organic contaminants, heavy metals can also be bioremediated by microbes (). Their immobilization, transformation, and detoxification in the environment can be achieved via various microbial activities (Fakhar et al., Citation2022). As important microbial genera in the bioremediation of metal-contaminated sites, Aeromonas, Bacillus, and Pseudomonas can reduce metal toxicity, as well as change the bioavailability and/or oxidation state of heavy metals including Cd, Cr, and Pb through different strategies and mechanisms (Fakhar et al., Citation2022). Biosorption occurs in bacterial removal of heavy metals such as Cd, Cr and Pb, i.e., Aeromonas trap metals through negatively-charged groups on the cell wall, Bacillus absorb metals via teichoic acid, carboxyl, and chitin, and Pseudomonas bind with metals by polysaccharides on their cell surface.
Unlike the degradation process in organic pollutants, bioremediation of metal-contaminated sites is more applicable to redox-active metals like Cr and U. This is because different redox forms show different toxicity, i.e., they show higher toxicity at oxidized states (CrVI and UVI), but lower toxicity at reduced states (CrIII and UIV) (Cui et al., Citation2022; Xia et al., Citation2019). For example, bioremediation of Cr-contaminated sites includes many processes, including fixation via extracellular complexation, cell surface sorption, intracellular accumulation, reduction of CrVI to CrIII to lower its toxicity, and mineralization via conversion of metals into solid-phase minerals (Xia et al., Citation2019). Similar to Cr, U is another metal with different valences in the environment. However, compared to Cr, U is more complex because it is not only chemically toxic but also radioactive (Cui et al., Citation2022). Nevertheless, U toxicity can also be reduced by microbes isolated from U-contaminated environments through sorption, mineralization, and biochemical reduction.
3. Phytoremediation of sites with organic and inorganic contaminants
Phytoremediation can be applied to both organic (e.g., perfluoroalkyl and polyfluoroalkyl substances, pesticides, and PAHs) and inorganic contaminants (e.g., heavy metals including Cd, Pb, U and Cr). To determine its feasibility, field trials have been conducted (Wang et al., Citation2020). However, in the field, many factors impact the performance of phytoremediation, including contaminant concentrations, nutrient contents, amendment application, plant species, and soil characteristics. Wang et al. (Citation2020) discussed the field application of phytoremediation and associated factors, which provide some practical guidelines for better phytoremediation of contaminated sites.
3.1. Phytoremediation of organic contaminants
Perfluoroalkyl and polyfluoroalkyl substances (PFASs) are ubiquitous chemicals, which are commonly used as industrial products and widely accumulated in the environment and biota including plants. Jiao et al. (Citation2021) summarized the advances in their absorption, accumulation, and distribution in plants as well as their metabolisms. Generally, there are two ways for PFASs to enter plants, i.e., root absorption from soil/water and foliar absorption from the atmosphere. After entering the plants, PFASs are detoxified or eliminated through enzymatic pathways. Jiao et al. (Citation2021) discussed key enzymes such as alcohol dehydrogenase and aldehyde dehydrogenase during plant metabolisms of representative PFAS compounds.
Among plants, perennial grass Vetiver is special as it shows characteristics of high productivity, deep-rooting, and high tolerance to various abiotic stresses. Therefore, Vetiver grass and associated microbes have been applied in phytoremediation (Chen et al., Citation2021). The chemical oxygen demand, total dissolved/suspended solids, and total fungal/bacterial/coliform counts of compost leachate are decreased after Vetiver treatment. Additionally, other organic contaminants including petroleum hydrocarbon, phenol, 2,4,6-trinitrotoluene, atrazine, benzo[a]pyrene, and trichloroethylene can be biodegraded and/or removed by Vetiver grass.
3.2. Phytoremediation of inorganic contaminants
Among phytoremediation, the use of hyperaccumulating plants, which can accumulate >1000 mg·kg−1 metal for Pb, Cu, Cr and As, and >100 mg·kg−1 metal for Cd in the shoots, is common. Such an example is As-hyperaccumulator Pteris vittata (Ma et al., Citation2001). Vetiver grass has been applied in sites contaminated by heavy metals such as Cd and Pb, but it is not a hyperaccumulator (Xia et al., Citation2019). Based on Cui et al. (Citation2022) and Xia et al. (Citation2019), several plants have been identified, including Nicotiana tabacum and Sesbania rostrata for U, and Dicoma niccolifera and Sutera fodina for Cr, which can accumulate U and Cr.
For instance, N. tabacum can accumulate U up to 937 mg·kg−1 in its shoots after exposing to 0.5 mM U under hydroponics (Cui et al., Citation2022). Recently, A. thaliana has attracted considerable attention in U removal and several studies have proved its tolerance for U. This is because A. thaliana is capable of scavenging reactive oxygen species to alleviate oxidative damages like lipid peroxidation with its well-developed antioxidant enzyme system, including superoxide dismutase, guaiacol peroxidase, and catalase. It has been reported that the U content in the growth medium increased its root growth and shoot biomass at 1-2 μM U, showing its potential for U remediation (Cui et al., Citation2022).
As Cr-hyperaccumulators, the maximum Cr concentrations in D. niccolifera and S. fodina reached 1,500 and 2,400 mg·kg−1 in the leaves (Xia et al., Citation2019). Up to date, only few plants can be categorized as Cr-hyperaccumulators because Cr is mainly sequestrated in the roots to decrease its toxicity, with poor translocation to the shoots including P. vittata and P. ensiformis (de Oliveira et al., Citation2014, Citation2018; Xia et al., Citation2019). In plant rhizosphere, CrVI tends to be reduced to CrIII and bind with cell walls, resulting in the decrease of Cr translocation (Xia et al., Citation2019). Consequently, plants with efficient reduction capability can help remediate Cr-contaminated sites by converting CrVI to CrIII, thereby lowering its toxicity and mobility.
4. Strategies to improve bioremediation and phytoremediation
Despite having many advantages, biological remediation has several limitations including lengthy periods, environmental factors, nutrient limitation, contaminant bioavailability, and phytotoxicity. To improve remediation efficiency, several strategies have been used, such as immobilization of microbial/microalgal cells, construction of microbial/microalgal consortia, and use of different amendments.
4.1. Immobilization of microbial/microalgal cells
Immobilization is to confine biocatalysts such as bacterial cells and microalgae inside or on the carrier or support materials (Partovinia & Rasekh, Citation2018; Usmani et al., Citation2022). Compared to free cells, immobilized cells are easier to be separated and re-utilized. In addition, they are more tolerant to changes in pH and temperature, and contaminant toxicity. Several studies show that the biodegradation of organic contaminants can be improved using immobilized microbial cells, especially in harsh environments. For example, after 10 days of operation, the attenuation of organic contaminants including pharmaceuticals (sulfacetamide, sulfamethazine, and sulfamethoxazole) and herbicides (bromacil, atrazine, diuron, and bentazone) increased from 36% to 51% using immobilized microalgae (Nguyen et al., Citation2021). Compared to 37% with free cell system, the immobilized Zoogloea sp. in polyvinyl alcoholin-alginate beads show 85% biodegradation of phenanthrene in slurry phase (Partovinia & Rasekh, Citation2018). However, cell immobilization technology has some drawbacks including high cost, and complicated and/or laborious cell immobilization processes. Besides, low cell survivability during immobilization is another concern.
4.2. Consortia of different microbes/microalgae
Compared to single species, microbial consortia, such as algae-bacteria consortia, perform better in bioremediation, which is less sensitive to fluctuations in environmental fluctuations and more resistant to stress caused by contaminants (Nguyen et al., Citation2021). Several studies show that dissolved organic C secreted by algae can act as C sources for bacterial growth and in return, bacteria stimulate algae growth by mineralizing nutrient elements including N, P, and S. The syntrophic interactions of algae-bacteria consortia help to remove contaminants in aqueous/aquatic systems, including nutrients (C, N, and P), heavy metals, pesticides, PPCPs, phenolic compounds, naphthenic acids and dyes (Li et al., Citation2022; Nguyen et al., Citation2021). For example, compared to the algae-free system, the decolorization and mineralization rate of Congo red in the C. vulgaris-bacteria consortia system increased by 93% and 8%, respectively (Li et al., Citation2022). Such improvements may relate to higher biosorption and bioconversion, more extracellular polymeric substances and membrane fluidity, and stronger coagulation behavior. Besides, mixed populations of microbes/microalgaes in the consortia can perform multi-functions that are difficult or even impossible for single species. For example, the removal rate of pharmaceuticals including acetaminophen, aspirin, and ketoprofen is up to 98-100% in stirred-tank photobioreactors with microalgae-bacteria consortium (Nguyen et al., Citation2021).
4.3. Use of amendments
Various amendments have been used to improve the biological remediation of contaminated sites. Such amendments include chemical agents (i.e., trace elements, H2S, nitrate, and nitrite; Chen et al., Citation2022; Miri et al., Citation2019), nanomaterials (i.e., zero-valent iron, hydroxyapatite, and fullerene particles; Chen et al., Citation2022; Song et al., Citation2019), industrial waste-based additives (i.e., coal fly ash, red mud, and steel slag; Wang et al., Citation2020), bio-based materials (i.e., biochar, sewage sludge, and compost; Wang et al., Citation2020) and microbial agents (i.e., phosphate-solubilizing bacteria, nitrogen-fixing bacteria, and arbuscular mycorrhizal fungi; Wang et al., Citation2020; Xia et al., Citation2019).
The amendment-based improvements in remediation mainly contributed to better nutrient availability in the substrate, regulation of oxidation-reduction potential, protective effects on the organisms, reduction of contaminant toxicity, and alteration of contaminant bioavailability. For example, as micronutrient Cu concentration is increased from 3 μM to 6 μM, the removal rate of nitrite is enhanced from 1.26 to 3 mM d−1 because Cu accelerates the activity and growth of DAMO bacteria, as well as the expression of key enzyme methane monooxygenase (Chen et al., Citation2022). As a reductive material, zero-valent iron can effectively create a stably-reductive environment. With its addition, the total nitrogen removal rate is enhanced by 24% due to the promotion of obligate anaerobe growth and acceleration of the startup time in Anammox systems (Chen et al., Citation2022). Besides, the applications of fullerene nanoparticles can enhance the uptake of trichloroethylene by eastern cottonwood up to 26-82% (Song et al., Citation2019). Under cold climate conditions, amendments rich in N and P can stimulate the bioremediation of petroleum hydrocarbons due to their enhancement on microbial growth (Miri et al., Citation2019).
In contaminated sites where vegetation is difficult to be established, amendments can improve plant growth by enhancing soil nutrients and reducing contaminant bioavailability and ecotoxicity. A field experiment shows that the use of red mud increases the biomass production of silver grass due to the reduction in phytoavailable heavy metals (Cd, Pb, and Zn) (Wang et al., Citation2020). Similarly, biochar from hardwood reduces soil acidity and metal toxicity, as well as improves soil fertility and water availability, thereby promoting plant growth (Wang et al., Citation2020).
Microbial agents are effective in promoting plant growth and contaminant removal. With the inoculation of arbuscular mycorrhizal fungi, the biomass of Dandelion and Bermuda grass grown in a Cr-contaminated soil increased by 232 and 85%, respectively (Xia et al., Citation2019). As such, the inoculated microbes improve nutrient absorption, decrease the toxic effects of contaminants, and secrete plant hormones to promote plant growth, thereby enhancing phytoremediation. In another example, the total phenol degradation is >90% in less than 4 days after a co-application of bacteria Burkholderia sp. to plants (Brassica chinensis and Ipomoea aquatica), compared to 38–50% without microbial amendment (Wang et al., Citation2020).
5. Summary
This editorial focuses on biological remediation including bioremediation and phytoremediation based on 24 articles in CREST during 2018–2022. Biological remediation has been used to treat sites contaminated with organic and inorganic contaminants, including traditional (nutrients, petroleum hydrocarbons, organo-pesticides, plastics, and heavy metals Cd, Cr, Pb, and U) and emerging contaminants (PPCPs and PFASs). To improve biological remediation efficiency, several improvement strategies, such as the immobilization of microbial/microalgal cells, construction of microbial/microalgal consortia, and the use of amendments have been applied. Besides, the metabolism pathways and interactive mechanisms among organisms, enzymes, and contaminants are highlighted, which may provide theoretical guidance for the application of biological remediation at contaminated sites.
Based on articles focusing on biological remediation during the last five years in CREST, further research is needed. They include: 1) screening plant and microbe species with excellent environmental adaptability and remediation capability, particularly, hyperaccumulators and multifunctional microbes for emerging contaminants, including PFASs and plastics; 2) developing new consortia of different microbes/microalgae with higher remediation efficiency and environmental adaptability; 3) developing more effective and feasible improvement strategies, especially for extreme environments such as low-temperature, drought condition, and multiple contaminations; and 4) exploring intrinsic effects of plant density, environmental parameters, and amendment dosage on phytoremediation efficiency during field trials. In addition, during biological remediation, the complex interactions between algae and bacteria, microbes and plants, as well as organisms and contaminants should be explored, especially at molecular levels.
Institute of Soil and Water Resources and Environmental Science, College of Environmental and Resource Sciences, and Zhejiang Provincial Key Laboratory of Agricultural Resources and Environment, Zhejiang University, Hangzhou, China
Peng Gao
Department of Environmental and Occupational Health, and Department of Civil and Environmental Engineering, University of Pittsburgh, Pittsburgh, Pennsylvania, USA
Eakalak Khan
Department of Civil and Environmental Engineering and Construction, University of Nevada, Las Vegas, Nevada, USA
Chang-Ping Yu
Graduate Institute of Environmental Engineering, National Taiwan University, Taipei, Taiwan
[email protected]
Disclosure statement
No potential conflict of interest was reported by the authors.
References
- Briceño, G., Fuentes, M. S., Saez, J. M., Diez, M. C., & Benimeli, C. S. (2018). Streptomyces genus as biotechnological tool for pesticide degradation in polluted systems. Critical Reviews in Environmental Science and Technology, 48(10–12), 773–805. https://doi.org/10.1080/10643389.2018.1476958
- Chen, X. W., Wong, J. T. F., Wang, J., & Wong, M. H. (2021). Vetiver grass-microbe interactions for soil remediation. Critical Reviews in Environmental Science and Technology, 51(9), 897–938. https://doi.org/10.1080/10643389.2020.1738193
- Chen, Y., Jiang, G., Sivakumar, M., & Wu, J. (2022). Enhancing integrated denitrifying anaerobic methane oxidation and Anammox processes for nitrogen and methane removal: A review. Critical Reviews in Environmental Science and Technology, 53(3), 390–415. https://doi.org/10.1080/10643389.2022.2056391
- Cheng, J., Yuan, J., Li, S., Yang, X., Lu, Z., Xu, J., & He, Y. (2022). Promoted reductive removal of chlorinated organic pollutants co-occurring with facilitated methanogenesis in anaerobic environment: A systematic review and meta-analysis. Critical Reviews in Environmental Science and Technology, 52(14), 2582–2609. https://doi.org/10.1080/10643389.2021.1886890
- Cui, Q., Zhang, Z., Beiyuan, J., Cui, Y., Chen, L., Chen, H., & Fang, L. (2022). A critical review of uranium in the soil-plant system: Distribution, bioavailability, toxicity, and bioremediation strategies. Critical Reviews in Environmental Science and Technology, 53(3), 340–365. https://doi.org/10.1080/10643389.2022.2054246
- de Oliveira, L. M., Ma, L. Q., Santos, J. A. G., Guilherme, L. R. G., & Lessl, J. T. (2014). Effects of arsenate, chromate, and sulfate on arsenic and chromium uptake and translocation by arsenic hyperaccumulator Pteris vittata. Environmental Pollution, 184, 187–192. https://doi.org/10.1016/j.envpol.2013.08.025
- de Oliveira, L. M., Suchismita, D., da Silva, E. B., Gao, P., Vardanyan, L., Liu, Y., & Ma, L. Q. (2018). Interactive effects of chromate and arsenate on their uptake and speciation in Pteris ensiformis. Plant and Soil, 422(1–2), 515–526. https://doi.org/10.1007/s11104-017-3480-x
- Fakhar, A., Gul, B., Gurmani, A. R., Khan, S. M., Ali, S., Sultan, T., Chaudhary, H. J., Rafique, M., & Rizwan, M. (2022). Heavy metal remediation and resistance mechanism of Aeromonas, Bacillus, and Pseudomonas: A review. Critical Reviews in Environmental Science and Technology, 52(11), 1868–1914. https://doi.org/10.1080/10643389.2020.1863112
- Fang, Z., Gao, Y., Wu, X., Xu, X., Sarmah, A. K., Bolan, N., Gao, B., Shaheen, S. M., Rinklebe, J., Ok, Y. S., Xu, S., & Wang, H. (2020). A critical review on remediation of bisphenol S (BPS) contaminated water: Efficacy and mechanisms. Critical Reviews in Environmental Science and Technology, 50(5), 476–522. https://doi.org/10.1080/10643389.2019.1629802
- Harindintwali, J. D., Xiang, L., Wang, F., Chang, S. X., Zhao, Z., Mei, Z., Jia, Z., Jiang, X., Zhu, Y-g., & Tiedje, J. M. (2022). Syntrophy of bacteria and archaea in the anaerobic catabolism of hydrocarbon contaminants. Critical Reviews in Environmental Science and Technology. https://doi.org/10.1080/10643389.2022.2134702
- Jiao, X., Shi, Q., & Gan, J. (2021). Uptake, accumulation and metabolism of PFASs in plants and health perspectives: A critical review. Critical Reviews in Environmental Science and Technology, 51(23), 2745–2776. https://doi.org/10.1080/10643389.2020.1809219
- Leal, C. S., Mesquita, D. P., Amaral, A. L., Amaral, A. M., & Ferreira, E. C. (2020). Environmental impact and biological removal processes of pharmaceutically active compounds: The particular case of sulfonamides, anticonvulsants and steroid estrogens. Critical Reviews in Environmental Science and Technology, 50(7), 698–742. https://doi.org/10.1080/10643389.2019.1642831
- Li, S., Zhang, C., Li, F., Ren, N., & Ho, S. (2022). Recent advances of algae-bacteria consortia in aquatic remediation. Critical Reviews in Environmental Science and Technology, 53(3), 315–339. https://doi.org/10.1080/10643389.2022.2052704
- Ma, L. Q., Komar, K. M., Tu, C., Zhang, W., Cai, Y., & Kennelley, E. D. (2001). A fern that hyperaccumulates arsenic. Nature, 409(6820), 579. https://doi.org/10.1038/35054664
- Miri, S., Naghdi, M., Rouissi, T., Kaur Brar, S., & Martel, R. (2019). Recent biotechnological advances in petroleum hydrocarbons degradation under cold climate conditions: A review. Critical Reviews in Environmental Science and Technology, 49(7), 553–586. https://doi.org/10.1080/10643389.2018.1552070
- Nguyen, H. T., Yoon, Y., Ngo, H. H., & Jang, A. (2021). The application of microalgae in removing organic micropollutants in wastewater. Critical Reviews in Environmental Science and Technology, 51(12), 1187–1220. https://doi.org/10.1080/10643389.2020.1753633
- Paço, A., Jacinto, J., Da Costa, J. P., Santos, P. S. M., Vitorino, R., Duarte, A. C., & Rocha-Santos, T. (2019). Biotechnological tools for the effective management of plastics in the environment. Critical Reviews in Environmental Science and Technology, 49(5), 410–441. https://doi.org/10.1080/10643389.2018.1548862
- Partovinia, A., & Rasekh, B. (2018). Review of the immobilized microbial cell systems for bioremediation of petroleum hydrocarbons polluted environments. Critical Reviews in Environmental Science and Technology, 48(1), 1–38. https://doi.org/10.1080/10643389.2018.1439652
- Sidhu, G. K., Singh, S., Kumar, V., Dhanjal, D. S., Datta, S., & Singh, J. (2019). Toxicity, monitoring and biodegradation of organophosphate pesticides: A review. Critical Reviews in Environmental Science and Technology, 49(13), 1135–1187. https://doi.org/10.1080/10643389.2019.1565554
- Song, B., Xu, P., Chen, M., Tang, W., Zeng, G., Gong, J., Zhang, P., & Ye, S. (2019). Using nanomaterials to facilitate the phytoremediation of contaminated soil. Critical Reviews in Environmental Science and Technology, 49(9), 791–824. https://doi.org/10.1080/10643389.2018.1558891
- Usmani, Z., Sharma, M., Lukk, T., Karpichev, Y., Thakur, V. K., Kumar, V., Allaoui, A., Awasthi, A. K., & Gupta, V. K. (2022). Developments in enzyme and microalgae based biotechniques to remediate micropollutants from aqueous systems-A review. Critical Reviews in Environmental Science and Technology, 52(10), 1684–1729. https://doi.org/10.1080/10643389.2020.1862551
- Wang, J., de Ridder, D., van der Wal, A., & Sutton, N. B. (2021). Harnessing biodegradation potential of rapid sand filtration for organic micropollutant removal from drinking water: A review. Critical Reviews in Environmental Science and Technology, 51(18), 2086–2118. https://doi.org/10.1080/10643389.2020.1771888
- Wang, L., Hou, D., Shen, Z., Zhu, J., Jia, X., Ok, Y. S., Tack, F. M. G., & Rinklebe, J. (2020). Field trials of phytomining and phytoremediation: A critical review of influencing factors and effects of additives. Critical Reviews in Environmental Science and Technology, 50(24), 2724–2774. https://doi.org/10.1080/10643389.2019.1705724
- Xia, S., Song, Z., Jeyakumar, P., Shaheen, S. M., Rinklebe, J., Ok, Y. S., Bolan, N., & Wang, H. (2019). A critical review on bioremediation technologies for Cr(VI)-contaminated soils and wastewater. Critical Reviews in Environmental Science and Technology, 49(12), 1027–1078. https://doi.org/10.1080/10643389.2018.1564526
- Zdarta, J., Nguyen, L. N., Jankowska, K., Jesionowski, T., & Nghiem, L. D. (2022). A contemporary review of enzymatic applications in the remediation of emerging estrogenic compounds. Critical Reviews in Environmental Science and Technology, 52(15), 2661–2690. https://doi.org/10.1080/10643389.2021.1889283
- Zhang, X., Yu, T., Li, X., Yao, J., Liu, W., Chang, S., & Chen, Y. (2019). The fate and enhanced removal of polycyclic aromatic hydrocarbons in wastewater and sludge treatment system: A review. Critical Reviews in Environmental Science and Technology, 49(16), 1425–1475. https://doi.org/10.1080/10643389.2019.1579619
- Zhu, Y., Wang, J., Liu, Q., Jin, Y., Ding, L., & Ren, H. (2022). Principles for quorum sensing-based exogeneous denitrifier enhancement of nitrogen removal in biofilm: A review. Critical Reviews in Environmental Science and Technology. https://doi.org/10.1080/10643389.2022.2136932