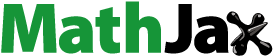
ABSTRACT
Performing wet bench experiments to search for lead molecules for drug delivery is a long and tedious process where computational tools have played a crucial role. Molecular docking studies have been carried out for selecting the nanocarrier, and the results of the computational studies have been validated using the model protein Ova albumin and anticancer drug 5-Fluorouracil (5-FU) with mesoporous silica (MSNPs) and chitosan nanoparticles (CSNPs) as the nanocarriers. Formulated nanocarriers were tested for in-vitro release, which showed a sustained release of the drugs. In-vitro studies on the lung cancer cell line A459 revealed excellent biocompatibility and non-toxic nature of the designed drug delivery system. This chitosan nanoparticle-based drug delivery system could have the potential for chemotherapeutic treatment of cancer.
Introduction
Research in nanobiotechnology has enabled nanocarrier-based cargo vehicles for the delivery of biological compounds in living organisms. To enhance the bioavailability of drugs that are poorly water-soluble and non-target specific, nanocarrier-based delivery systems have been proven successful. However, for efficient drug delivery, the choice of the nanosystem is always challenging [Citation1]. The efficiency of the carrier depends on the structural and chemical properties of the carrier as well as of the drug molecule. Out of various available nanocarrier systems, silica and chitosan are the materials of choice due to their biocompatible and/or biodegradable nature. Additionally, chitosan has been proven successful in shielding the drug molecules from its degradation by enzymes, providing better physicochemical stability [Citation2].
5-Fluorouracil (5-FU), an antineoplastic agent, is a first-line anticancer drug and a highly water-soluble metabolite [Citation3]. 5-FU has low molecular weight and is negatively charged (pKa ~8; 130.08 g/mol) and removed from the body rapidly via excretion (7–20% excreted via urine in 6 h and up to 90% in 1 h). However, one of the disadvantages of administering 5-FU drugs can be their lower average half-life (10–20 min) which is also dependent on the number of doses [Citation4]. To increase the effectiveness of 5-FU, combinatorial treatment or nano conjugation can be used.
Compatibility between the carrier and the drug molecule determines the stability of the nanocarrier system. Due to an infinite number of possible conformations between the two systems, the drug and the carrier, the determination of a perfect combination for efficient drug delivery becomes a difficult task. Taking the help of in-silico methods such as molecular docking provides the potential re-orientation of biomolecules’ conformation and non-covalent binding energies in molecules [Citation5].
Investigating the effect of various reaction parameters on the size of particles, researchers have discovered that with increasing ammonia and tetraethyl orthosilicate (TEOS) concentration, the size of silica (SiO2) particle increases but with increasing water concentration it decreases [Citation6]. Surface modifications of silica were also studied for better homogeneity in the mixing of organic and filler groups by silane coupling agents, which helps in conjugating silica nanostructure with polymers or proteins for medicine and biotechnology application [Citation7]. Chitosan showed antimicrobial properties by converting it into nano sizes of required surface charge and size [Citation8]. Preparation techniques and applications such as drug release and wastewater treatment of nano chitosan were reviewed [Citation9]. Biological studies on chitosan nanoparticles (CSNPs) exhibited them as non-toxic, having good stability, biodegradability and blood biocompatibility [Citation10]. Binding properties of ligand and target were studied which revealed that molecular docking is helpful in the prediction of the 3-D structure of any complex [Citation11]. Applications of molecular docking in understanding the unfavourable effects in drug delivery, reusing the drug, target fishing and profiling were illustrated, and future potential and applications have also been discussed by researchers [Citation12].
This study aims to compare the results obtained from the computational tools and wet bench experiments of loaded nanocarriers, MSNPs and CSNPs with ova albumin and 5-FU for efficient and sustained release of drug to lung cancer cells and to enhance its anti-cancer activities. Despite substantial research on anticancer drugs and polymers in nanosystems, there are scarcely any reports available for the relative studies of in-silico molecular docking to estimate the binding energies and interaction between the drug molecules and the nanocarriers. Hence, in this study, we have computationally explored the interaction of MSNPs and CSNPs with ova albumin and 5-FU which has prospective applications in drug delivery.
Experimental
Materials and methods
Reagent
All analytical-grade chemicals were used in this investigation. Tetraethyl orthosilicate (TEOS, 98%), Chitosan (CS with a medium molecular weight, and deacetylated chitin, Poly(D-glucosamine)), Sodium tripolyphosphate (STPP, 85%), Ova albumin (≥98%) and 5-Fluorouracil (5-FU, ≥99%) were purchased from Sigma Aldrich. Isopropyl alcohol (IPA, 99%), cetyltrimethylammonium bromide (CTAB, extra pure) and glacial acetic acid were purchased from SRL, India. Ammonia (NH3, 25%) was procured from Emparta, India. For all the experiments, distilled water (DW) was used.
Preparation of MSNPs
The conventional method was used to prepare monodispersed MSNPs as described below [Citation5]. In the synthesis procedure, appropriate amounts of aq. NH3, IPA and CTAB in DW were mixed and then sonicated for 15 minutes. The above mixture was then stirred magnetically at 50°C for 10 min. TEOS dissolved in IPA was then added dropwise to the mixture while stirring for 30 min (drop rate: 1 drop/2 min). The suspension was then stirred magnetically for 24 h at 600 rpm at room temperature (RT), 27°C. A white precipitate was formed which was later centrifuged and washed twice with DW and IPA, followed by oven-drying. The powder was calcined for 2 h at 600°C. The obtained MSNPs were characterised further.
Preparation of CSNPs
Ionic gelation of CS with TPP anions was performed to prepare CSNPs [Citation2]. One milligram per millilitre solution of CS was prepared with CS powder dissolved in 1% acetic acid. After it dissolved completely, NaOH (2 N) solution was added for maintaining the pH of the mixture around 4.5–5. Then, TPP dissolved in DW was added to CS solution dropwise maintaining 4:1 CS: TPP ratio, and the whole mixture was stirred magnetically for 3 h at 600 rpm followed by 24 h dialysis. The obtained solution was centrifuged at 5500 rpm for 15 min to remove the supernatant. The precipitate was allowed to dry and utilised for characterisation further.
Preparation of loaded MSNPs
In a classic procedure, Ova albumin/5-FU and MSNPs were mixed in a 2:1 ratio and added to 4 ml DW. The mixture was stirred magnetically for 24 h at 900 rpm, centrifuged for 15 min at 5500 rpm, and left undisturbed for the loaded NPs to settle. After which, the supernatant was removed and stored for advanced studies, and loaded NPs settled at the bottom of the vial were air-dried and characterised.
Preparation of loaded CSNPs
Three milligram per millilitre CS solution was prepared by dissolving CS powder into acetic acid (1%). TPP was dissolved in DW to form a 2 mg/ml TPP solution. Also, 5-FU/ova albumin was mixed with the TPP solution, and the whole mixture of TPP and 5-FU/ova alb was dropwise added to the CS solution. The final CSNP to 5-FU/ova albumin ratio was maintained at 2:1. The reaction mixture was stirred magnetically for 3 h at 900 rpm at room temperature. The obtained solution was then centrifuged, and the supernatant was separated after the powder settled completely and the air-dried powder was used for further characterisation.
Molecular docking studies
Protein/Drug–ligand docking studies were carried out based on the molecular structure of ova albumin and the molecular structure of 5-FU. All the extra elements and solvent molecules were removed from the structures. Molecular docking calculations for MSNP and CSNP were done with both ova albumin and 5-FU drug with the help of software. After performing different orientations of every protein–ligand pair, the binding energy for the best-suited combination was calculated by the software. Estimation of the binding energy and the docking studies were carried out using Hexa 8.0.0 Cuda and Autodock software. Visual representations of the complexes were obtained using PyMOL software.
In vitro release study
To show the pH-responsive release of the drugs from CSNPs and compare the molecular docking results, in vitro release study of ova albumin and 5-FU was carried out at two different pHs, 4.6 (acetate buffer) and 7.4 (phosphate buffer saline). The release studies were completed using the dialysis bag diffusion method. A fixed amount of nanocarrier and protein/drug were placed inside a dialysis bag (molecular cut-off 14,000 kDa) and kept in pH 4.6 and 7.4 buffers. After predetermined times, 1.5 ml of released sample solution was taken out for UV–vis spectroscopy measurement and the same amount of fresh buffer was added to the system for maintaining the sink conditions. By observing the absorbance values of the released samples at λmax~278 nm and 265 nm, the amounts of Ova albumin and 5-FU, respectively, were determined.
In vitro study for cell viability by MTT assay
MTT assay against RAW 264.7 cell line was performed to check the in vitro biocompatibility of MSNPs and CSNPs nanocarriers. In a typical procedure, RAW 264.7 (normal) cells were placed in 96-well plates along with growth media and incubated at 37°C for 24 h and CO2 (5%). To determine the cytocompatibility of nanocarriers on RAW cells, different concentrations such as 1, 3, 5, 10, 20, 40, 80 and 160 µg/ml of MSNPs and CSNPs nanocarriers were mixed with the RAW 264.7 cells and incubated at 37°C for another 24 h in CO2 (5%). MTT dye (20 µl) was added after incubation to each well and again incubated for 4 h. Then, fresh DMSO (100 µl) was added to replace the media. After which, 96-well plates were shaken slowly to break down the purple-coloured formazan crystals in DMSO and the OD (optical density) was calculated with a microplate reader at 580 nm wavelength. All the experiments were carried out in triplicates for accuracy. The viability of the cells was calculated from the given equation:
To determine the toxicity and cytocompatibility of the loaded MSNP and CSNP nanocarrier on normal (RAW 264.7) and A459 lung cancer cells, an MTT assay of the loaded samples was performed by the same method as the one described previously. The concentrations of the dose of 5-FU loaded MSNPs were 1, 2.5, 5, 10, 20, 40 and 80 µg/ml. The concentrations of the dose of 5-FU loaded CSNPs were 0.05, 0.1, 0.5, 1, 2, 4 and 8 µg/ml.
Characterisations
A powder x-ray diffractometer (XRD, Bruker D8 Advance) was utilised to investigate the crystallinity of the prepared nanoparticles. The specific surface area, pore size and pore volume of MSNPs and CSNPs were studied using Brunauer–Emmett–Teller (BET, Micromeritics 2030) surface area analyser. Particle sizes and morphology of synthesised samples were analysed by field emission scanning electron microscopy (Carl Zeiss Ultra 55 FESEM). Hydrodynamic sizes and zeta potential of nanoparticles were measured using a particle size analyser (Malvern ZEN5600 Zetasizer nano instrument). A Fourier transform infrared (FTIR) spectrometer (Perkin-Elmer FTIR spectrometer) was utilised to study the MSNPs, CSNPs and loaded nanoparticles by their distinct functional groups. A UV–vis spectrometer (Perkin Elmer, Lambda-750) was used for the release profile studies of the loaded nanocarriers.
Results and discussion
Zeta potential and hydrodynamic size of the prepared nanoparticles
The MSNP and CSNP were prepared by stober’s and ionic gelation methods, respectively. The zeta potential and average hydrodynamic size were determined by the particle size analyser.
The average hydrodynamic size of ova-loaded MSNP was found to be slightly less than that of the bare MSNPs, which might be related to the agglomeration of the bare MSNPs. The hydrodynamic size of MSNPs increased after the loading of 5-FU due to the coating of the drug onto the NP surface. Also, the hydrodynamic size of CSNPs increased after ova albumin and 5-FU loading due to the coating of protein/drug on the NPs surface.
Zeta potential was measured to understand the effect of loading of the drug into and/or on the NPs and is shown in . Zeta potential measures the electrostatic attraction or repulsion between the particles in the suspension and thus is a measure of dispersion or stability of nanoparticles in the suspension. Particles with zeta potential between +10 mV and −10 mV are counted as neutral and can eventually aggregate, while particles with zeta potential of more than +30 mV and less than −30 mV are counted as stable or highly dispersed. As mentioned in , MSNPs and ova-loaded MSNPs were moderately dispersed in suspension, while 5-FU loaded MSNPs, CSNPs, ova-loaded CSNP and 5-FU loaded CSNPs were neutral. According to Xun Lv et al., the increased zeta potential of ova and 5-FU loaded MSNP is an indication that at least some amount of drug is adsorbed on the surface of the nanoparticles [Citation13]. For CSNP, the lowering of zeta potential values confirmed the attachment of the drug molecules to chitosan, which correlates well with the experiments performed by S. Wazed Ali et al. [Citation2].
Table 1. Hydrodynamic size and values of zeta potential of loaded and unloaded MSNPs and CSNPs.
Crystallographic assay
Crystallographic nature of mesoporous silica, CS and CSNP were determined by powder-XRD using CuKα radiation (CuKα = 1.540598 Å) at RT. In , the prominent peak in the chitosan diffractogram at 2θ = 20.2° indicates high degree of crystallinity of chitosan. Against this, no sharp peak is found in chitosan nanoparticles diffractogram, indicating characteristics of an amorphous structure. Greater disorder in chain arrangement in the nanoparticles has been observed after cross-linking through XRD [Citation2]. The X-ray amorphous structure of the CSNPs has been attributed to the condensed network of polymer chains which are cross-linked by TPP ions [Citation14]. The wide peak around ~23° in can be assigned to and attributed to the amorphous nature of silica [Citation6].
Microstructural observation
Morphological properties of MSNPs, CSNPs and loaded nanoparticles have been studied using the FESEM technique.
It is observed from the micrograph, as shown in , that MSNPs and CSNPs are spherical with mean diameters ~400 and 300 nm, respectively. shows ova-loaded MSNPs and CSNPs with a size of ~480 and 680 nm, respectively. shows 5-FU loaded MSNPs and CSNPs with a size of ~530 and 580 nm, respectively. Not much difference in size is observed after ova loading into MSNPs, which might be due to loading of the maximum amount of protein into the pores of NPs. After loading 5-FU into both MSNP and CSNP, size was observed to be increased due to the coating of the drug onto the NP surface. The average size of the particles estimated from the microscopy data is less for both MSNP and CSNP than those studied by the DLS as discussed previously. This difference in size is due to the different conditions used while measuring in the two techniques and also the different measurement principles involved in the two cases. In DLS, hydrodynamic diameter is measured as the particles are diffused in aqueous media and are in swollen condition. On the contrary, in the FESEM measurement, particles are in the dry condition and hence in the obtained images particles are lower in size.
Fourier transform infrared (FTIR) analysis
FTIR studies of MSNPs and CSNPs were done to analyse the nanoparticles for the types of chemical bonds and functional groups present. FTIR spectra of MSNPs and CSNPs are shown in . The spectrum displays absorption bands due to Si-O asymmetric vibration (1091 cm−1), Si-OH (966 cm−1) and Si-O symmetric vibration (81 cm−1). These bands indicate a condensed silica network in the MSNP structure (). The major and broad band at 1091 cm−1 could be due to Si-O-Si asymmetric stretching vibrations. At 469 cm−1, the band represents the Si-O-Si bending vibration. The absorption band near 1640 cm−1 is due to deformational vibrations caused by adsorbed water molecules and surface silanols (broad band around 3563 cm−1). The bands at 2850–3061 cm−1 can be attributed to the C-H bond stretching mode, which may be due to the presence of left-out organic species in the pores of MSNPs [Citation13,Citation15].
Figure 3. FTIR spectrum of (a) silica NPs with and without template, (b) CSNPs, (c) ova albumin and ova-albumin-loaded MSNPs and (d) 5-FU and 5-FU-loaded MSNPs.
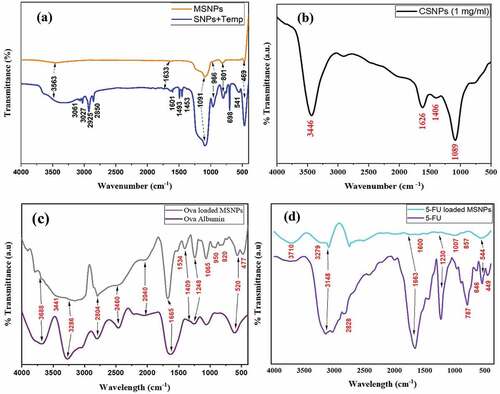
shows the FTIR spectrum of CSNPs which were prepared from chitosan and tripolyphosphate (TPP) by crosslinking. A less wide peak at 3446 cm−1 indicates reduced hydrogen bonding in CSNPs which is due to the formation of more open structure as a result of crosslinking between chitosan and TPP [Citation2]. As TPP links with the ammonium group of chitosan, stretching frequency gets reduced and H-bonding in CSNPs increases. For the IR spectrum of chitosan, C-O stretching of the primary alcoholic group was indicated by the typical bending vibration of N-H at 1626 cm−1 and absorption band at 1406 cm−1 [Citation16].
In FTIR spectrum of Ova-alb-loaded MSNPs (), the absorption peaks due to the terminal Si-OH, Si(OH)4 stretching vibration, Si-O-Si asymmetric stretching, Si-O stretching, Si-O-Si symmetric stretching and Si-O-Si bending vibrations at 3411, 1665, 1065, 950, 820 and 477 cm−1, respectively, confirmed the presence of MSNPs loaded with Ova albumin. The spectrum for ova alb showed specific peaks for amide I flexural vibration adsorption at 1665 cm−1 and for amide II at 1409 cm−1 [Citation17,Citation18]. The vibrations at 3286 cm−1 and 3688 cm−1 correspond to the stretching vibrations of O–H and N–H bonds, respectively, of amides in Ova alb. The COO− and C=O stretching bands of amides are at 1665 cm−1 and 2040 cm−1 respectively [Citation19]. The characteristic peaks of ova alb at 1665 cm−1 and 1409 cm−1 in the spectrum of ova loaded MSNPs confirmed the successful loading of protein into the carrier. Splitting of the combined peak of amine and amide at 1665 cm−1 and 1534 cm−1 also indicates the binding of silica with O and N of the ova structure.
In FTIR spectrum of MSNPs loaded with 5-FU (), the absorption peaks at 3279–3710, 1600, 1007, 857 and 544 cm−1 is due to the terminal Si-OH, Si(OH)4 stretching vibration, asymmetric stretching of Si-O-Si, symmetric stretching of Si-O-Si and Si-O-Si bending vibrations, respectively, which confirmed the existence of MSNPs along with 5-FU.
The observed bands for stretching of the aromatic C – N at 1230 cm−1 and for aromatic ring at the region from 500 to 600 cm−1 confirmed the loading of the 5-FU drug. Three distinguished peaks of the 5-FU are shown in the spectrum, where a broad peak at 3148 cm−1 displayed N-H stretching while C=O stretching is observed at 1663 cm−1, and the band at 1230 cm−1 corresponds to the stretching vibrations of the C-F bond [Citation20].
Study of porosity
N2 adsorption/desorption studies were conducted to characterise the porous nature and surface area of the formulated nanoparticles. shows the N2 adsorption/desorption isotherm for MSNP. According to the IUPAC classification, the isotherm obtained is of type IV and thus indicates the mesoporous nature of the formulated MSNPs. The BET surface area was observed to be ~2603.4 m2/g. The single point (at P/Po = 0.2816) and Langmuir surface area and were found to be ~2506.5 m2/g and ~4192.5 m2/g, respectively. Compared to the data available in the literature [Citation4,Citation21], the formulated MSNPs indicated a much higher surface area than already reported values. This might be due to the complete removal of the CTAB during calcination at 600°C for 2 h.
Figure 4. BET adsorption (a) – desorption (d) isotherm of (a) MSNP and (c) CSNP and differential logarithmic distribution of mesopore size of (b) MSNP and (d) CSNP.
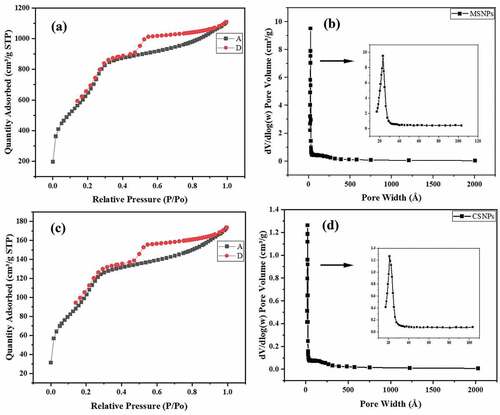
Due to the formation of mesoporous structures with high surface area, greater amount of drug is expected to be loaded and carried within the nanostructures thereby increasing the encapsulation capacity of the drug. The BJH pore size distribution graph of MSNPs () displayed a sharp peak at around 23.5 Å (~2.3 nm) having a pore volume of 1.26 cm3/g indicating that the formulated MSNPs have a uniform porous structure.
As per the IUPAC classification, the obtained isotherm at was of type IV and thus showed the existence of pores on the surface of the prepared CSNPs. The BET surface area of CSNPs was observed to be ~413.3 m2/g. The single point (at P/Po = 0.2816) and Langmuir surface area were found to be ~387 m2/g and ~671.6 m2/g, respectively. The BJH pore size distribution graph of CSNPs () displayed a sharp peak at around 21.1 Å (~2.1 nm) with a pore volume of 1.26 cm3/g indicating that the formulated CSNPs have a uniform porous structure.
Drug loading calculations
UV–Vis spectroscopy was performed to determine the amount of free drug in the supernatant after the loading experiment. The encapsulation capacity and encapsulation efficiency were calculated using the given formulas:
Ova-albumin-loaded nanoparticles
The calibration curve for ova albumin was obtained as below:
The maximum absorption (λmax) for ova albumin was observed at 278 nm as shown in . The concentration-dependent absorbance is shown in . To calculate the quantity of free drug in the supernatant and thus encapsulation capacity and efficiency, absorbance of the supernatant was recorded at λmax and results are as shown in .
Table 2. Drug loading capacities of ova alb/5-FU-loaded MSNPs and CSNPs.
5-FU-loaded nanoparticles
The calibration curve for 5-FU was obtained as below:
The maximum absorption (λmax) for 5-FU was observed at 265 nm as shown in . The concentration-dependent absorbance is shown in . To determine the quantity of free drug in the supernatant, absorbance of the supernatant was recorded at λmax, and the results are shown in .
In vitro cell viability study
MTT assay of MSNPs and CSNPs was carried out on RAW (normal) cells to examine the in vitro cytocompatibility of the given systems. shows the in vitro cell viability of the bare MSNPs in the dose concentration range of 1–160 µg/ml against RAW cells. MSNPs exhibited very less cell inhibition with outstanding biocompatibility on RAW cells. Only 0.7% of the cells were inhibited at a concentration of 40 µg/ml, and increased to 7.5% even at the highest concentration of 160 µg/ml.
Figure 7. Cell viability of (a) Normal (RAW 264.7) cells treated with various dose amounts of the MSNP and CSNP and different concentrations of 5-FU-loaded (b) MSNPs and (c) CSNPs treated with RAW 264.7 and lung cancer cell A459.
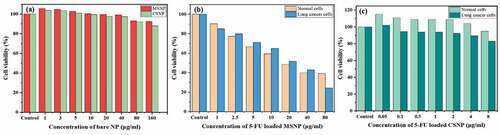
also displays the viability of normal cells when treated with bare CSNPs in the same dose concentration range of 1–160 µg/ml. The CSNPs also did not show much cell inhibition and around ~88.03% of the cells were viable at the highest concentration of 160 µg/ml. The obtained results suggest that prepared MSNP and CSNP carriers were not harmful or had negligible toxicity to RAW cells and hence can be employed as biocompatible drug nanocarriers.
Anticancer activity of the prepared MSNP-FU and CSNP-FU nanocarrier was examined by treating the samples with normal cells (RAW 264.7) and lung cancer cells. displays the in vitro cell viability of the loaded MSNP nanocarrier in the dose concentration range of 1–80 µg/ml against normal and cancerous cells. About 61% and 75.5% cell inhibition was observed in normal and lung cancer cells, respectively, at a dose concentration of 80 µg/ml. However, at a dose concentration less than 80 µg/ml, the cell inhibition rate of the normal cells was higher than that of the lung cancer cell. This might be due to the lack of target specificity of MSNPs. NPs might be releasing drug before reaching the cancerous cells as MSNPs are not pH selective. The in vitro cell viability of the loaded CSNP carrier in the dose concentration range of 0.05–8 µg/ml was examined against normal and cancerous cells as shown in . About 5% and 17.1% cell inhibition was observed in normal and lung cancer cells, respectively, at 8 µg/ml dose concentration. Cell inhibition was increased in the case of lung cancer cells, suggesting pH selectivity of the CSNPs. A Anitha et al. also showed that the release from the CSNPs was more and sustained in acidic, i.e. in tumour, environments than around normal cells due to the swelling of the polymer matrix in acidic environment as a result of protonation of the amine groups of the chitosan molecules [Citation22]. The observed cell inhibition is less in CSNPs than in MSNPs which may be due to less dose concentration. The efficiency of 5-FU is limited due to its short biological half-life; thus, it must be administered in the body at higher concentrations [Citation23].
Molecular docking study
The in-silico molecular docking studies help to eliminate improper compositions and provide a faster method for energy and structure determination for silica and chitosan nanoformulation based on ionic interaction between biodegradable and biocompatible polymers with drugs [Citation1].
The best possible compatibility of various nanocarriers and drugs was provided by molecular docking studies. As concluded by the docking studies and shown in that ova albumin can strongly bind with CS (B.E. = −452.98 kcal/mol) than with MSNPs (B.E. = −137.5 kcal/mol), and sustained delivery of drug can be related to the greater attraction towards the matrix. It also depends on other conditions such as pH and ionic strength. For 5-FU, studies showed a stronger attraction of 5-FU towards CS (B.E. = −100.65 kcal/mol) than MSNPs (B.E. = −58.62 kcal/mol). Thus, sustained drug release was observed in 5-FU loaded CSNPs. Hence, molecular docking studies provide useful information about the loading of the drug molecules to the carrier and the stability and release of drugs from NPs.
In-vitro release studies
The in-vitro release studies of ova albumin and 5-FU from CSNPs and MSNPs were performed in two different media for 64 h. NPs were used to obtain controlled drug release and to improve the bioavailability of the drug molecules.
Ova-loaded MSNPs exhibited an initial (up to 4 h) rapid release of ova albumin at pH 4.6 and pH 7.4 and the total drug release was about 0.77% as shown in . However, in 5-FU-loaded MSNPs, the observed release was about 18% and 40% at pH 4.6 and 7.4, respectively, as shown in . The initial rapid release was due to the desorption of the drug molecules from the NPs surface. As evident from the data of release studies of ova and 5-FU loaded MSNPs, the release was faster in the neutral medium than in the acidic medium. The release of ova from MSNPs was slower compared to the release of 5-FU from MSNPs, which correlates well with the prediction made from the molecular docking studies (lower binding energy of 5-FU-MSNPs).
Figure 9. Drug release studies at pH 4.6 and 7.4 of a) Ova-loaded MSNPs, b) 5-FU-loaded MSNPs, c) Ova-loaded CSNPs and d) 5-FU-loaded CSNPs.
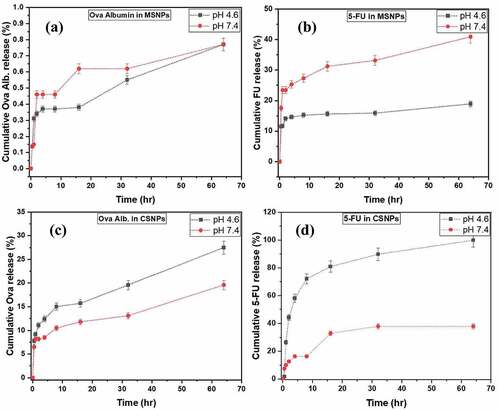
Ova-loaded CSNPs showed an initial (up to 4 h) burst release of ova albumin at pH 7.4 & pH 4.6 and the overall drug release was about 19% and 28%, respectively, as shown in . In 5-FU loaded CSNPs, the total release was observed about 38% and 100% at pH 7.4 and 4.6, respectively, and shown in . As evident from the data of release studies of ova albumin and 5-FU loaded CSNPs, the release was slower in the neutral medium than in the acidic medium. The chitosan polymer matrix swells in an acidic environment as the amine group of chitosan gets protonated facilitating faster release [Citation22]. Also, the larger release of 5-FU compared to ova from CSNPs could be correlated with the lower binding energy predicted from the molecular docking studies.
Conclusion
This paper reports the formulation of novel mesoporous silica and chitosan nanoparticles, predicts their drug carrier efficacy by computational studies and experimentally validates them. In-silico docking studies for MSNPs and CSNPs as nanocarriers and ova albumin and 5-FU as the drug molecules predicted CS to be the better nanocarrier for ova albumin. The current investigation also showed that CS-Ova was the most stable complex out of all the carrier-drug/protein combination studied, formed with a binding energy of −452.98 kcal/mol. This was further proven by wet-bench experiments. Results of in vitro drug release studies from MSNP and CSNP showed a release behaviour that is pH-dependent and is also important for smart drug delivery applications. It was noticed that release was faster in the neutral medium than in acidic for MSNP. On the other hand, the release was slower in the neutral medium than in acidic for CSNP. MTT assay showed the non-toxic nature of the nanocarriers against normal living cells. The obtained results suggest that the prepared nanocarriers with excellent biocompatibility, targeting ability and pH sensitivity could be a model formulation for smart drug delivery for the treatment of cancer. Also, the findings from molecular docking and wet-bench experiments conclude that the use of chitosan nanocarrier serves as a promising drug delivery vehicle to increase the therapeutic effect of 5-FU and can be used efficiently against cancer cells.
Acknowledgments
The authors sincerely acknowledge the financial support from the IoE Directorate through grant no UoH/IoE/RC1/RC1-20-017 dated 07-12-2020, University of Hyderabad. The technical support obtained from the Centre for Nanotechnology (CFN), School of Life Science (SLS) and ACRHEM from the University of Hyderabad is also gratefully acknowledged.
Disclosure statement
No potential conflict of interest was reported by the authors.
Additional information
Funding
References
- Yadav P, Bandyopadhyay A, Chakraborty A, et al. Enhancement of anticancer activity and drug delivery of chitosan-curcumin nanoparticle via molecular docking and simulation analysis. Carbohydr Polym. 2018;182:188–12.
- Wazed Ali S, Rajendran S, Joshi M. Synthesis and characterization of chitosan and silver loaded chitosan nanoparticles for bioactive polyester. Carbohydr Polym. 2011;83(2):438–446.
- Chandy T, Das GS, Rao GH. 5-Fluorouracil-loaded chitosan-coated polylactic acid microspheres as biodegradable drug carriers for cerebral tumours. J Microencapsulation. 2000;17(5):625–638. DOI:10.1080/026520400417676
- Moodley T, Singh M. Polymeric mesoporous silica nanoparticles for enhanced delivery of 5-fluorouracil in vitro. Pharmaceutics. 2019;11:288–309.
- Zhou C, Li S, Zhang Z, et al. Rapid synthesis of morphology-controlled mesoporous silica nanoparticles from silica fume. J Taiwan Inst Chem Eng. 2016;66:1–6.
- Qasim M, Ananthaiah J, Dhara S, et al. Synthesis, and characterization of ultrafine colloidal silica nanoparticles. Adv Sci Eng Med. 2014;6:965–973.
- Ab Rahman I, Padavettan V. Synthesis of silica nanoparticles by sol-gel: size-dependent properties, surface modification, and applications in silica-polymer nanocomposites—a review. J Nanomater. 2012;2012:1–15. article ID 132424. DOI:10.1155/2012/132424.
- Ali SW, Joshi M, Rajendran S. Synthesis, and characterization of chitosan nanoparticles with enhanced antimicrobial activity. Int J Nanosci. 2011;10(4 & 5):979–984.
- Jassal PS, Gupta S, Chand N, et al. Chitosan nanoparticles: synthesis and their applications. J Basic Appl Eng Res. 2016;3(8):713–716.
- Gomathi T, Prasad S, Sudha PN, et al. Size optimization and in vitro biocompatibility studies of chitosan nanoparticles. Int j biol macromol. 2017;104:1794–1806.
- Mahmood Dar A, Mir S. Molecular docking: approaches, types, applications, and basic challenges. J Anal Bioanal Tech. 2017;8(2):1–3.
- Pinzi L, Rastelli G. Molecular docking: shifting paradigms in drug discovery. Int J Mol Sci. 2019;20(18):4331.
- Lv X, Zhang L, Xing F, et al. Controlled synthesis of monodispersed mesoporous silica nanoparticles: particle size tuning and formation mechanism investigation. Microporous Mesoporous Mater. 2016;225:238–244.
- Shah PV, Rajput SJ. Facile synthesis of chitosan capped mesoporous silica nanoparticles: a pH-responsive smart delivery platform for raloxifene hydrochloride. AAPS Pharm Sci Tech. 2018;19:1344–1357.
- Wu X, Farooq MA, Li T, et al. Cationic chitosan-modified silica nanoparticles for oral delivery of protein vaccine. J Biomed Mater. 2021;109(11):2111–2119. DOI:10.1002/jbm.a.37198
- Li GY, Jiang Y-R, Huang K-L, et al. Preparation and properties of magnetic Fe3O4–chitosan nanoparticles. J Alloys Compd. 2008;466:451–456.
- Popat A, Liu J, Lu GQ, et al. A pH-responsive drug delivery system based on chitosan-coated mesoporous silica nanoparticles. J Mater Chem. 2012;22:11173–11178.
- Nosrati H, Sefidi N, Sharafi A, et al. Bovine serum albumin coated iron oxide magnetic nanoparticles as biocompatible carriers for the curcumin-anticancer drug. Bioorg Chem. 2018;76:501–509.
- Singh P, Raizada P, Pathania D, et al. Preparation of BSA-ZnWO4 nanocomposites with enhanced adsorptional photocatalytic activity for methylene blue degradation. Int J Photoenergy. 2013;2013: 1–7. article ID 726250. doi: 10.1155/2013/726250
- Patra P, Seesala VS, Das D, et al. Biopolymeric nanogel derived from functionalized glycogen towards targeted delivery of 5-fluorouracil. Polymer. 2018;140:122–130.
- Qiao ZA, Zhang L, Guo M, et al. Synthesis of mesoporous silica nanoparticles via controlled hydrolysis and condensation of silicon alkoxide. Chem Mater. 2009;21:3823–3829.
- Anitha A, Deepagan VG, Divya Rani VV, et al. Preparation, characterization, in vitro drug release and biological studies of curcumin loaded dextran sulphate–chitosan nanoparticles. Carbohydr Polym. 2011;84:1158–1164.
- Beňová E, Bergé-Lefranc D, Zeleňák V, et al. Adsorption properties, the pH-sensitive release of 5-fluorouracil, and cytotoxicity studies of mesoporous silica drug delivery matrix. Appl Surface Sci. 2020;504:144028.