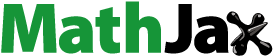
ABSTRACT
Li2TiSiO5 is a ‘zero-strain’ anode material with a large theoretical capacity, appropriate operation potential, and good cyclability. However, its low electronic conductivity negatively affects its practical capacity and rate performance. Herein, we improve the electrochemical performance of Li2TiSiO5 by carbon-coating and nanosizing dual modifications, and further explore its lithium-storage and ‘zero-strain’ mechanisms by in-situ XRD. Within 0.2–3.0 V, the carbon-coated Li2TiSiO5 nanowires deliver a large reversible capacity of 257 mAh g−1, high rate performance with a 5000 mA g−1 vs. 50 mA g−1 capacity ratio of 71.6%, and excellent cyclability with 90.0% capacity retention at 5000 mA g−1 over 4000 cycles. The special crystal structure of Li2TiSiO5 with electrochemical active TiO6 octahedra surrounded by inactive SiO4 and LiO6 polyhedra can effectively strengthen the volume-buffering capability, resulting in the ‘zero-strain’ behaviour with a tiny lattice-volume change of only 0.41%. Therefore, this dual-modified Li2TiSiO5 material has great practicability for high-performance lithium storage.
Introduction
Nowadays, lithium ion batteries (LIBs) are among the best power sources with good electrochemical performances, such as high specific energy and good cyclability [Citation1–4]. They are widely applied in a variety of devices used in the fields of portable electronics, electric vehicles, aviation, and military equipment [Citation5]. For LIBs, high-performance cathode and anode materials are highly desirable [Citation6–9]. As the commonly used anode material, graphite has a stable electrochemical performance with a reversible capacity approaching to its theoretical capacity of 372 mAh g−1. However, its operation potential is too low (∼0.1 V), which may cause the formation of lithium dendrites, resulting in short circuit [Citation10–13]. Li4Ti5O12 is another successfully commercialized anode material for LIBs. As a ‘zero-strain’ material, Li4Ti5O12 has excellent cyclability and a safe operation potential of ∼1.5 V. Nevertheless, its theoretical capacity is too small (175 mAh g−1), and its overly high operation potential has a negative impact on its energy density [Citation14,Citation15].
Li2TiSiO5, a recently-developed ‘zero-strain’ anode material, has a theoretical capacity of 315 mAh g−1 based on the Ti3+/Ti4+ and Ti2+/Ti3+ redox reactions and an appropriate operation potential, which appropriately tackle the issues in graphite and Li4Ti5O12 [Citation16]. However, it suffers from a low electronic conductivity, resulting in the negative impacts on its rate capability and reversible capacity [Citation17]. So far, however, only a few studies on the modifications of Li2TiSiO5 have been reported [Citation16–18]. In addition, the crystal-structural evolution and ‘zero-strain’ mechanism of Li2TiSiO5 are still unclear and should be clarified.
In this work, we improve the electrochemical performance of Li2TiSiO5 by carbon-coating and nanosizing dual modifications, and investigate its mechanisms by an in-situ XRD combined with Rietveld refinements. Within 0.2–3.0 V, the resulting carbon-coated Li2TiSiO5 (C-Li2TiSiO5) nanowires show a reversible capacity as large as 257 mAh g−1 with an appropriate operation potential of 0.83 V and high rate performance with a 5000 mA g−1 vs. 50 mA g−1 capacity ratio of 71.6%. The excellent cyclability with 90.0% capacity retention at 5000 mA g−1 over 4000 cycles confirms the ‘zero-strain’ behaviour of Li2TiSiO5 with a tiny lattice-volume change of 0.41%. The ‘zero-strain’ behaviour is mainly rooted in the ultra-stable crystal structure of Li2TiSiO5 with electrochemical active TiO6 octahedra surrounded by inactive SiO4 and LiO6 polyhedra, which can effectively enhance the volume-buffering capability during lithiation/delithiation. Therefore, the C-Li2TiSiO5 nanowires are suitable for high-performance lithium storage.
Experimental
Material synthesis
LiOH (99.9%, Macklin), C16H36O4Ti (99.9%, Macklin), and C8H12O8Si (95%, Macklin) in a molar ratio of 2 : 1 : 1 were dissolved in 10 mL ethanol. Then, 0.5 g polyvinylpyrrolidone (PVP, Mw = 1300000, Macklin) was dissolved, stirring for 2 h. The obtained electrospinning solution was transferred to a syringe with a 19 G needle. The provided voltage was 15 kV. The film collector was about 10 cm under the spinneret. The collected film was calcinated at 870°C for 4 h in argon with a ramping rate of 2°C min−1, obtaining the C-Li2TiSiO5 nanowires.
Material characterization
The X-ray diffraction (XRD) test was conducted on an Ultra IV diffractometer to characterize the crystal structure of the C-Li2TiSiO5 nanowires, and the obtained XRD spectrum was Rietveld-refined with the aid of the free GSAS programme [Citation19]. The field emission scanning electron microscopy (FESEM) test was performed on a JEOL JSM-7800F to collect morphological features. The energy-dispersive X-ray spectroscopy (EDX) test was conducted on an OXFORD X-Max to examine the element distributions. The transmission electron microscopy (TEM) was applied using a JEOL JEM-2100F. An ASAP 2460 surface area analyser was used to determine the Brunauer−Emmett−Teller (BET) specific surface area. The compositions and elemental valences were examined by X-ray photoelectron spectroscopy (XPS) characterizations on a PHI5000 Versaprobe III spectrometer.
Electrochemical measurement
To prepare the working electrodes, the C-Li2TiSiO5 nanowires, conductive carbon, and polyvinyl difluoride were well mixed into N-methyl-2-pyrrolidone in a mass ratio of 7 : 2 : 1. The obtained slurry was evenly spread on copper foils with a mass loading of ∼1.0 mg cm−2 and dried in vacuum at 110°C for 10 h. In a glove box filled with argon, CR2032 coin-type half cells were assembled by using the C-Li2TiSiO5 working electrodes and lithium-metal anode. The electrolyte was a mixture of dimethyl carbonate, ethylene carbonate, and diethylene carbonate in equal volumes with 1 M LiPF6. The separators were Whatman GF/D-1832 glass fibres.
The electrochemical performance and galvanostatic intermittent titration technique (GITT) experiments were carried out using a CT-3008 battery tester. Cyclic voltammetry (CV) was conducted using a CHI660E electrochemical workstation. All the electrochemical performances of the C-Li2TiSiO5 nanowires were investigated within 0.2–3.0 V.
In-situ XRD characterization
To study the crystal-structural change of the C-Li2TiSiO5 nanowires, an in-situ XRD half cell (Scistar LIB-XRD) equipped with a beryllium window was assembled [Citation20–22]. The separator was Whatman GF/D-1832 glass fibre. The recorded in-situ XRD spectra were Rietveld-refined by the free GSAS programme.
Results and discussion
The XRD spectrum of the C-Li2TiSiO5 nanowires is shown in , and the Rietveld refined data are summarized in Table S1 and Table S2. Li2TiSiO5 exhibits a tetragonal crystal structure and P4/nmm space group [Citation23]. The refined lattice constants of Li2TiSiO5 are a = 6.44059(53) Å, c = 4.40981(52) Å, and V = 182.925(46) Å3. The crystal structure of Li2TiSiO5 () contains electrochemical active TiO6-octahedron chains, which are surrounded by inactive SiO4-tetrahedron and LiO6-octahedron chains along the c axis. Each TiO6 octahedron corner-shares with four LiO6 octahedra and four SiO4 tetrahedra, and face-shares with four LiO6 octahedra. As a result, interconnected channels with large sizes are formed. The alternate arrangement of the active and inactive chains with an interconnected polyhedron network plays an important role in accommodating the volume change, and significantly contributes to the crystal-structure stability [Citation24].
Figure 1. Physico-chemical characterizations of the C-Li2TiSiO5 nanowires. a) XRD spectrum with Rietveld-refinement. b) Crystal structure. c) FESEM image. d) EDX mapping images. e) HRTEM image (inset: SAED pattern).
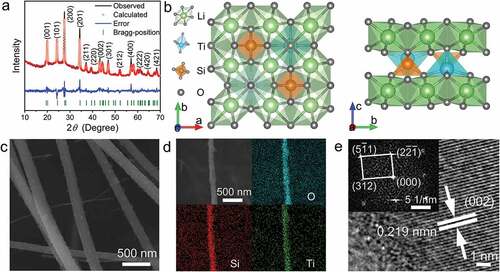
The FESEM image () clearly shows a nanowire morphology with a diameter range of 100 − 200 nm, and the large BET specific surface area is 31.90 m2 g−1 (Fig. S1). The EDX mapping images () exhibit the homogeneous distributions of Ti, Si, and O in the C-Li2TiSiO5 nanowires. The lattice spacing in the HRTEM image () of the C-Li2TiSiO5 nanowires is determined to be 0.219 nm, corresponding to the (002) crystallgraphic plane of Li2TiSiO5. The regular diffraction spots ( inset) reveal the single-crystalline characteristic of the Li2TiSiO5 primary particles and can be assigned to the P4/nmm space group. The content of coated-carbon in the C-Li2TiSiO5 nanowires is determined to be 9.43 wt% from the TGA result (Fig. S2).
The CV curves after the first cycle are well overlapped at 0.1 mV s−1 (), indicating the high reversibility after the initial activation. The cathodic peak located at 0.66 V in the first cycle is resulted from the solid-electrolyte-interface (SEI) formation. The cathodic/anodic peak-pairs located at 0.23/0.69 V can be based on the Ti2+ ↔ Ti4+ redox reactions [Citation16].
Figure 2. Electrochemical performance of the C-Li2TiSiO5 nanowires. a) CV curves for first four cycles at 0.1 mV s−1, b) first four-cycle GCD curves at 50 mA g−1, c) GCD curves at various current densities, d) rate performance, and e) cyclability of the Li2TiSiO5/Li half cell.
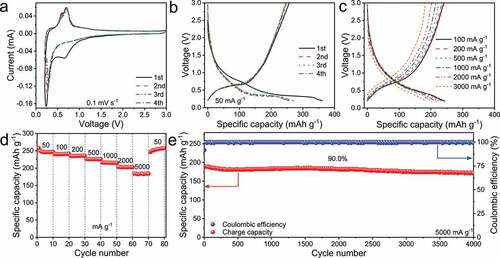
The galvanostatic charge – discharge (GCD) curves () of the C-Li2TiSiO5/Li half cell at various current densities show very similar shapes, confirming the high reversibility during lithiation and delithiation after the first activation. At 50 mA g−1, the reversible capacity of the C-Li2TiSiO5 nanowires is as large as 257 mAh g−1, and the operation potential is averaging at an appropriate value of 0.83 V (). This material retains large reversible capacities of 257, 240, 235, 226, 216, 203, and 184 mAh g−1 () at 50, 100, 200, 500, 1000, 2000, and 5000 mA g−1. When the current rate returns to 50 mA g−1, the reversible capacity recovers to 246 mAh g−1, showing its good electrochemical stability. The 5000 mA g−1 vs. 50 mA g−1 capacity ratio of 71.6% indicates its high rate performance. When the half cell is cycled at 5000 mA g−1 over 4000 cycles (), the capacity retention is up to 90.0%, revealing its excellent cyclability. Therefore, the electrochemical performance of the Li2TiSiO5 material in this work is among the best results in the Li2TiSiO5 research field (Table S3).
The ex-situ XPS characterizations reveal that the redox mechanism of the C-Li2TiSiO5 nanowires is based on the Ti4+ ↔ Ti2+ redox reaction. The Ti-2p peaks of the pristine C-Li2TiSiO5 nanowires () consist of Ti-2p1/2 (464.3 eV) and Ti-2p3/2 (458.6 eV) respectively, which correspond to Ti4+ [Citation25]. When lithiated to 0.2 V (), the Ti valence is changed to a combination of Ti3+ (19%) and Ti2+ (81%) [Citation26]. When delithiated to 3.0 V (), the Ti valence is recovered to a combination of Ti4+ (76%) and Ti3+ (23%). Therefore, the active Ti3+/Ti4+ and Ti2+/Ti3+ redox couples in Li2TiSiO5 are identified. The fact that a small portion of Ti3+ ions are not oxidated to Ti4+ during delithiation is reasonable since a small portion of inserted Li+ ions during initial delithiation are trapped in the Li2TiSiO5 lattice [Citation27–29].
Figure 3. XPS examinations and GITT experiment of the C-Li2TiSiO5 nanowires. Ex-situ Ti-2p XPS spectra of a) pristine, b) lithiated, and c) delithiated states. d) CV curves at various scan rates. e) Calculation of b values. f) DLi variations during lithiation and delithiation.
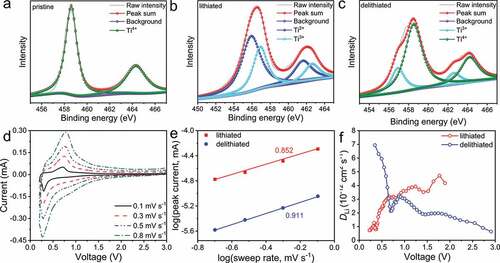
As the scan rate increases (), the small shifts of the CV peaks demonstrate the good electrochemical kinetics with low polarization. To further study the electrochemical kinetics, the recorded CV data is analysed based on the equation of I = avb (). In this equation, I and v respectively represent the peak current and scan rate, and a and b are changeable parameters [Citation30–32]. The b value should be in a range of 0.5 − 1, and the larger b value indicates the larger contribution of the capacitive behaviour. In the case of the C-Li2TiSiO5 nanowires, the b values are as large as 0.852 during lithiation and 0.911 during delithiation, suggesting that the capacitive behaviour remarkably contributes to the lithium storage, which can be mainly due to the large specific surface area of the C-Li2TiSiO5 nanowires.
To characterize the Li+ diffusivity of the C-Li2TiSiO5 nanowires, a GITT experiment is conducted at 0.1C within 0.2–3.0 V. Fig. S3a presents the GITT curves of the C-Li2TiSiO5/Li half cell after the first-cycle activation, and a single typical GITT step is clearly shown in Fig. S3b. According to the Fick’s second law, the apparent Li+ diffusion coefficients (DLi) at different states can be determined according to Eq. (1) [Citation33,Citation34]:
In this equation, Vm represents the molar volume of Li2TiSiO5, mb represents the real mass of the C-Li2TiSiO5 nanowires, Mb represents the molar mass of Li2TiSiO5, τ represents the pulse duration time, S represents the electrode surface area, L represents the electrode thickness, and ΔES and ΔEτ are respectively the changes in the total potential and equilibrium potential during the current pulse. As recorded in Fig. S3c, the voltage during the single titration shows a linear relationship with τ0.5. Consequently, Eq. (1) is simplified to Eq. (2):
ΔES and ΔEτ are obtained by the GITT curves (Fig. S3b). The DLi values of the C-Li2TiSiO5 nanowires are calculated and recorded in , reaching 2.58 × 10−12 and 2.70 × 10−12 cm2 s−1 during lithiation and delithiation, respectively, which are about three orders larger than that of Li4Ti5O12 thin films [Citation35]. Apparently, the large Li+ diffusion coefficients of the C-Li2TiSiO5 nanowires together with their remarkable capacitive behaviour undoubtedly contributes to their good rate performance.
In order to explore the lithium-storage mechanism of the C-Li2TiSiO5 nanowires, an in-situ XRD characterization is performed at 0.5C within 0.2–3.0 V [Citation36–38]. The collected in-situ XRD spectra of the first lithiation and delithiation process are recorded in . During lithiation, all the XRD peak intensities are obviously decreased since the inserted Li+ ions decrease the crystal structure order [Citation37], and the peak intensities almost recover during delithiation, which suggests the excellent structural reversibility and stability of the C-Li2TiSiO5 nanowires. The negligible peak shifts during lithiation and delithiation indicate the tiny lattice-constant changes (), revealing the Li+ intercalation mechanism and the ‘zero-strain’ behaviour of the C-Li2TiSiO5 nanowires.
Figure 4. In-situ XRD characterization of the C-Li2TiSiO5 nanowires. a) Contour in-situ XRD spectra and b) original in-situ XRD spectra. Enlarged contour in-situ XRD spectra within c) 20.0–20.4° and d) 34.4–34.7°. e) Variations in lattice constants of Li2TiSiO5.
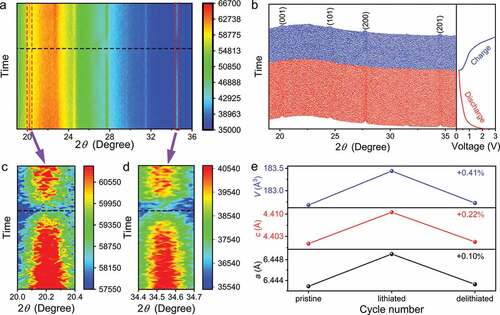
The tiny and reversible lattice-constant changes during lithiation – delithiation are confirmed by refining the collected in-situ XRD spectra (). After lithiation at 0.2 V, the a and c values slightly increases by 0.22 and 0.10%, respectively. Consequently, the V value shows a similar variation trend with an increase of 0.41%. This V-value change is among the smallest percentages in the research field of lithium-storage materials (Table S4), thereby leading to the excellent cyclability stability of the C-Li2TiSiO5 nanowires.
To explore the reason for the ‘zero-strain’ behaviour of Li2TiSiO5, its crystal-structure-change details at delithiated and lithiated states are carefully analysed by refining the collected in-situ XRD data, and the obtained bond-length, atomic-parameter, and polyhedron-volume changes are listed in Table S5–S9. After the lithiation with the external Li+ ions from the electrolyte intercalated into the 2a and 4e sites, no movements of the Li (4d) and Si4+ ions are observed, while the active Ti ions show a slightly shift along the c axis. Consequently, the Ti – O (8i), Li (4d)–O (2c), and Si – O (8i) bond lengths increase, and the Li (4d)–O (8i) and Ti – O (2c) bond lengths decrease. The O2− (8i) ions obviously shift to the inserted Li+ (2a) ions, and the O2− (2c) ions shift to the inserted Li+ (4e) ions. The lithiation expands the active TiO6-octahedron volume by 1.72% since the Ti4+ ions are converted to Ti3+/Ti2+ ions with larger ion sizes [Citation39], but slightly expands the inactive LiO6-octahedron volume by 1.16% and the SiO4-tetrahedron volume by 0.91%. Clearly, the inactive LiO6-octahedra and SiO4-tetrahedra have excellent volume-buffering capability, effectively buffering the volume expansions of the surrounded TiO6 octahedra. In addition, the interconnected polyhedron network can also effectively accommodate the expansions of LiO6, TiO6, and SiO4 polyhedra. Therefore, the ‘zero-strain’ behaviour of Li2TiSiO5 is achieved.
Conclusions
The practical capacity and rate performance of Li2TiSiO5 are improved by carbon-coating and nanosizing dual modifications. The carbon-coated Li2TiSiO5 nanowires show wire diameters of 100 − 200 nm and carbon percentage of 9.43 wt%. The Ti4+ ↔ Ti2+ redox reaction provides a large reversible capacity of 257 mAh g−1 within 0.2–3.0 V at 50 mA g−1 and an appropriate average operation potential of 0.83 V. The fast Li+ transport and remarkable capacitive behaviour effectively contribute to the good rate performance with a 5000 mA g−1 vs. 50 mA g−1 capacity ratio of 71.6%. The excellent cyclability with 90.0% capacity retention at 5000 mA g−1 over 4000 cycles is undoubtedly due to the ‘zero-strain’ behaviour of Li2TiSiO5. The obviously expanded active TiO6 octahedra are surrounded by slightly expanded inactive LiO6 octahedra and SiO4 tetrahedra, resulting in a tiny lattice-volume expansion of only 0.41%. This work provides new insights for exploring high-performance lithium-storage materials and more ‘zero-strain’ materials.
Supplemental Material
Download MS Word (223.8 KB)Disclosure statement
No potential conflict of interest was reported by the authors.
Supplementary material
Supplemental data for this article can be accessed online at https://doi.org/10.1080/10667857.2023.2204292.
References
- Wang L, Liu T, Wu T, et al. Strain-retardant coherent perovskite phase stabilized Ni-rich cathode. Nature. 2022;611:61–7.
- Wang C, Liu T, Yang X, et al. Fast charging of energy-dense lithium-ion batteries. Nature. 2022;611:485–490.
- Liu T, Liu J, Li L, et al. Origin of structural degradation in Li-rich layered oxide cathode. Nature. 2022;606:305–312.
- Bi Y, Tao J, Wu Y, et al. Reversible planar gliding and microcracking in a single-crystalline Ni-rich cathode. Science. 2020;370:1317–1323.
- Schmuch R, Wagner R, Hörpel G, et al. Performance and cost of materials for lithium-based rechargeable automotive batteries. Nat Energy. 2018;3:267–278.
- Chai Y, Guo Y, Wang Y, et al. TiO2 nanoparticles coated Co3O4/C microspherical as a high rate lithium storage material. Mater Technol. 2022;37:2588–2597.
- Yang J, Ren K, Hong M, et al. New insight into lattice variations of Ni-rich NMC811 cathode induced by Li2ZrO3 coating. Mater Technol. 2022;37:1926–1935.
- Zhang Q, Ji S, Yan C, et al. Insights into the porosity and electrochemical performance of nano Li2FeSiO4 and Li2FeSiO4/C composite cathode materials. Mater Technol. 2022;37:1195–1204.
- Huang C, Li R, Luo L, et al. The exploration of a CuNb3O8 Li+-storage anode compound. Mater Technol. 2022;37:814–821.
- Sivakkumar SR, Nerkar JY, Pandolfo AG. Rate capability of graphite materials as negative electrodes in lithium-ion capacitors. Electrochim Acta. 2010;55:3330–3335.
- Waldmann T, Wilka M, Kasper M, et al. Temperature dependent ageing mechanisms in lithium-ion batteries – a post-mortem study. J Power Sources. 2014;262:129–135.
- Lv C, Lin C, Zhao X. Rational design and synthesis of nickel niobium oxide with high-rate capability and cycling stability in a wide temperature range. Adv Energy Mater. 2021;11:2102550.
- Kim MS, Lee BH, Park JH, et al. Operando identification of the chemical and structural origin of Li-ion battery aging at near-ambient temperature. J Am Chem Soc. 2020;142:13406–13414.
- Zhao B, Ran R, Liu M, et al. A comprehensive review of Li4Ti5O12-based electrodes for lithium-ion batteries: the latest advancements and future perspectives. Mater Sci Eng R. 2015;98:1–71.
- He Y, Li B, Liu M, et al. Gassing in Li4Ti5O12-based batteries and its remedy. Sci Rep. 2012;2:913.
- Liu J, Pang W, Zhou T, et al. Li2TiSiO5: a low potential and large capacity Ti-based anode material for Li-ion batteries. Energy Environ Sci. 2017;10:1456–1464.
- He D, Wang B, Wu T, et al. TiO2 nanocrystal-framed Li2TiSiO5 platelets for low-voltage lithium battery anode. Adv Funct Mater. 2020;30:2001909.
- Li Y, Mei Y, Lan X, et al. Insight into effects of niobium on electrospun Li2TiSiO5 fibers as anode materials in lithium-ion batteries. Mater Res Bull. 2021;136:111145.
- Toby B. EXPGUI, a graphical user interface for GSAS. J Appl Crystallogr. 2001;34:210–213.
- Ma S, Jiang T, Deng J, et al. VPO5: an all-climate lithium-storage material. Energy Storage Mater. 2022;46:366–373.
- Jiang T, Ma S, Deng J, et al. Partially reduced titanium niobium oxide: a high-performance lithium-storage material in a broad temperature range. Adv Sci. 2022;9:2105119.
- Huang W, Yang C, Miao N, et al. A novel temperature-dependent electrochemical system for electrode materials for time resolved X-ray diffraction. Scr Mater. 2022;211:114529.
- Slobodin BV, Surat LL, Zubkov VG, et al. Structural, luminescence, and electronic properties of the alkaline metal-strontium cyclotetravanadates M2Sr(VO3)4, (M=na, K, Rb, Cs). Phys Rev B. 2005;72:155205.
- Yang L, Liang G, Cao H, et al. A new sodium calcium cyclotetravanadate framework: “zero-strain” during large-capacity lithium intercalation. Adv Funct Mater. 2021;31:2105026.
- Larsson P, Andersson A, Wallenberg R, et al. Combustion of CO and toluene; characterisation of copper oxide supported on titania and activity comparisons with supported cobalt, iron, and manganese oxide. J Catal. 1996;163:279–293.
- Gonbeau D, Guimon C, Pfister-Guillouzo G, et al. XPS study of thin films of titanium oxysulfides. Surf Sci. 1991;254:81–89.
- Zhang L, Zhang X, Tian G, et al. Lithium lanthanum titanate perovskite as an anode for lithium ion batteries. Nat Commun. 2020;11:3490.
- Lu X, Jian Z, Fang Z, et al. Atomic-scale investigation on lithium storage mechanism in TiNb2O7. Energy Environ Sci. 2011;4:2638–2644.
- Jayaraman S, Aravindan V, Kumar PS, et al. Exceptional performance of TiNb2O7 anode in all one-dimensional architecture by electrospinning. ACS Appl Mater Interfaces. 2014;6:8660–8666.
- Augustyn V, Come J, Lowe MA, et al. High-rate electrochemical energy storage through Li+ intercalation pseudocapacitance. Nat Mater. 2013;12:518–522.
- Augustyn V, Simon P, Dunn B. Pseudocapacitive oxide materials for high-rate electrochemical energy storage. Energy Environ Sci. 2014;7:1597–1614.
- Liu J, Wang J, Xu C, et al. Advanced energy storage devices: basic principles, analytical methods, and rational materials design. Adv Sci. 2018;5:1700322.
- Weppner W, Huggins RA. Determination of the kinetic parameters of mixed-conducting electrodes and application to the system Li3Sb. J Electrochem Soc. 1977;124:1569–1578.
- Fu Q, Zhu X, Li R, et al. A low-strain V3Nb17O50 anode compound for superior Li+ storage. Energy Storage Mater. 2020;30:401–411.
- Wunde F, Berkemeier F, Schmitz G. Lithium diffusion in sputter-deposited Li4Ti5O12 thin films. J Power Sources. 2012;215:109–115.
- Zhang Y, Liu J, Wang H. Alter martensitic phase transformation kinetics by forming Ni-rich nanolayer in metastable austenitic steels. Sci China Technol Sci. 2019;62:546–550.
- Cava RJ, Murphy DW, Zahurak SM. Lithium insertion in Wadsley-Roth phases based on niobium oxide. J Electrochem Soc. 1983;30:2345–2351.
- Peng N, Cheng X, Yu H, et al. LiY(MoO4)2 nanotubes: novel zero-strain anode for electrochemical energy storage. Energy Storage Mater. 2019;21:297–307.
- Zhang Q, Ma S, Wang W, et al. “Zero-strain” K2SrV4O12 as a high-temperature friendly Li+-storage material. Energy Storage Mater. 2022;52:637–645.