ABSTRACT
Recurrent eczematous lesions and acute itching describe chronic actinic dermatitis (CAD). Continuous use of corticosteroids might result in a dermal adverse effect. Chitosan (CS)-coated PLGA nanoparticles encapsulated prednisolone (PDS) and co-encapsulated to poloxamer hydrogel to enhance anti-inflammatory action and reduce side effects. The PDS@NPs were synthesized using the solvent-emulsification evaporation method, and their physical and chemical properties were analysed. Ex vivo drug absorption experiment was conducted utilizing the Franz diffusion cells in vitro. Toxic effects on human fibroblasts and keratinocytes were not observed in the nanoparticle formulations. Nanoparticles and hydrogels altered PDS’s release kinetics, but not by the non-encapsulated PDS (NE@PDS). Nanoparticles could not penetrate the stratum corneum of removed the skin, which shows the nano-encapsulation of PDS improved skin absorptions. Pseudoplastic and non-Newtonian behaviour was seen in all hydrogels. The nanoformulations appear to be a promising option for glucocorticoid delivery to individuals with chronic actinic dermatitis (CAD).
Introduction
Chronic actinic dermatitis (CAD) is a skin condition that affects millions daily. Environmental and psychological variables and genetic predispositions to immunological dysfunction and skin barrier abnormalities contribute to CAD development [Citation1–3]. An extensive T cell infiltration characterizes the cutaneous lesions caused by CAD, mainly constituted of memory/effector T cells [Citation4]. During the chronic phases of CAD, the spinous and cornified layers thicken, resulting in the formation of lichenified lesions, which are visible. Many immunoglobulins E (IgE)-bearing Langerhans cells can be found deep into the epidermis [Citation5]. The pathophysiology of CAD is still a mystery since it is a recurrent illness with several contributing factors [Citation6]. Th2 immune dysregulation and changes in the epidermal barrier can cause CAD. Each of these changes can lead to the occurrence of the others, producing a vicious cycle that is the root cause of the lesions that develop [Citation7–9].
Since the causes of CAD are so diverse, there isn’t a single therapy that works for everyone [Citation10]. A combination of pharmacological and nonpharmacological methods is used to alleviate itching, decrease inflammation, and restore skin barrier function [Citation11–13]. Despite the importance of nonpharmacological treatments (e.g. moisturizers), topical corticosteroids continue to be the first-line therapy for moderate to severe CAD management [Citation14]. It is a solid non-halogenated corticosteroid with high anti-inflammatory properties and poor mineralocorticoid properties because of its low systemic toxicity [Citation15]. Prednisolone (PDS) There are various cell types that PDS can inhibit; they include mast cells (which are immune cells), macrophages (which are white blood cells), neutrophils (which are white blood cells), and eosinophils (which are white blood cells) [Citation16]. Dermal atrophy is a common adverse effect of extended usage of topical corticosteroids despite their effective anti-inflammatory properties [Citation17]. The constant use of corticosteroids burdens CAD patients.
Improved methods of delivering drugs to the patient can lead to better outcomes. Topical administration of many drugs has been widely explored for the past decade using nano-based formulations for skin disease therapy [Citation18–20]. Using nanoencapsulation, the dosages needed to achieve biological activity are reduced, side effects are minimized because of targeted administration, and novel compounds may now be employed pharmacologically. As a result, nanoencapsulation may aid in raising the apparent solubility of the drugs and reduce skin permeability while boosting the drug’s residence duration in the SC and viable epidermic and dermal layers [Citation21–23]. Increased bioavailability and less systemic toxicity can be achieved with this method. As an added benefit, therapeutic molecules may be released in a controlled manner in response to physiological cues like pH, temperature, enzyme activity, and other stimuli using nano-based formulations that respond to inputs [Citation24].
The FDA-approved polymer polylactide-co-glycolide acid (PLGA) has been widely employed in tissue engineering for biocompatibility and biodegradability, medical therapy, and drug delivery [Citation25–27]. PLGA’s hydrophobic nature makes it suitable for loading hydrophobic drugs like PDS. Peptide-coated lignin (PLGA) has been shown to release drugs rapidly when heated to 36.5°C [Citation28–30]. Sustained-release properties of PLGA nanoparticles have been explored by coating them with CS [Citation31–33]. They may also easily approach the cell membrane thanks to the cationic polymer nature of CS, which gives them excellent formability, bioadhesive, and biodegradability [Citation34–36]. With PLGA-coated CS nanoparticles and poloxamer-based thermoresponsive hydrogels, a sustained-release and bio adhesive stimuli-response system with sustained-release capabilities was developed [Citation37–39]. Polymers like poly lactic-co-glycolic acid (PLGA) and chitosan (CS) are often used to overcome the several issues, and they also help accomplish mucoadhesive ocular controlled drug delivery [Citation40]. For example, cationic CS is a biodegradable polymer with positively charged amino groups. Chitosan (CS) possesses antibacterial, bio-adhesive, and haemostatic properties, making it a perfect candidate in wound healing systems [Citation41–43]. CS was reported to promote wound healing by enhancing the migration of fibroblasts and collagen deposition in the wound area [Citation44]. Further, CS is a perfect carrier for different wound healing agents that protect these agents from side reactions in the wound microenvironment that may cause their deactivation while simultaneously increasing their absorption and targeting [Citation45–47]. Based on previous advantages, we are focusing on CAD management in the future. Aiming to develop and analyse PDS-loaded chitosan-coated nanoparticles utilizing the emulsion/evaporation process and subsequent diffusion into poloxamer hydrogel, this work was performed. On the one hand, we assessed the chemical characteristics of the NPs. Further, we examined the hydrogel’s mechanical and structural properties. MTT and ROS generation tests measured the toxicity of a drug’s skin penetration into human belly skin.
Experimental section
Materials
All other chemicals were obtained from Sinopharm Chemical Reagent Co. Ltd. (Shanghai, China). Polyvinyl alcohol (PVA), Low-molecular-weight chitosan, and Polylactide-co-glycolide acid (PLGA) were purchased from Aladdin (Shanghai, China). Prednisolone (PDS) was ordered from the Beyotime Institute of Biotechnology (Shanghai, China). Cell assay kits and 3-(4,5-dimethylthiazol-2-yl)-2,5-diphenyltetrazolium bromide (MTT) were purchased from Gen-View Scientific Inc. A ROS Assay Kit was ordered from Beyotime Biotechnology. The freeze-dried scaffolds’ structure, porous morphology, and elemental composition were investigated using a field emission scanning electron microscope (FE-SEM, Zeiss 300 Gemini, Germany). Dynamic light scattering (DLS) and ζ- potential results were recorded on a Zetasizer Nano ZS instrument (Malvern).
Nanoparticles fabrication and characterizations
The emulsification/evaporation method developed by Zhou et al. [Citation48] was used to fabricate PDS@NPs. It was essential to generate an organic phase from 100 mg of PLGA and 40 mg of Myritol 318 and PDS in 10 mL of acetone, methylene chloride in 20 mL, and acetone in 10 mL. When the organic phase was homogenized with 50 mL of an aqueous suspension PVA (3 mg/mL) for 8 min, the ultraturrax (14,000 rpm) was used to disperse it. Evaporation of 5 mL of organic solvent was carried out in the next step using a Rotatory Evaporator. Chitosan solution (0.5%) was added to the colloidal dispersion and agitated for 1 h. PDS was used at a final concentration of 0.4% in the final nanoformulation [Citation49].
Cell proliferation assay
Normal human primary keratinocytes and fibroblasts were cultured in a DMEM medium (supplemented with 10% FBS, 100 IU/mL penicillin, 100 μg/mL streptomycin, and 2 mM L-glutamine). All cells were maintained under standard conditions for 24 h. Then, 0, 5, 25, and 50 μg/mL concentrations of PDS, PLGA-NPs, and PDS@PLGA-NPs were added, and cells were incubated for 24 and 48 h. After treatment, the DMEM medium was replaced with a 0.5 mg/mL MTT solution and incubated for 4 h at 37°C. Then, 100 μL of DMSO was added to dissolve the precipitated formazan, and the mixture was shaken for 20 min [Citation50–54]. A microplate reader was used to measure the absorbance (570 nm). Eventually, the IC50 was calculated for samples [Citation49].
Reactive oxygen species (ROS) assay
The cells were seeded into 24-well plates at 0.5 × 104 cells/well, and the cells were maintained under standard conditions for 24 h. Then, 0, 5, 25, and 50 μg/mL concentrations of PDS, PLGA-NPs, and PDS@PLGA-NPs were added, and cells were incubated for 24 and 48 h. After hydrogen peroxide (H2O2) (100 μM) for 30 min, the cells were incubated for positive control. The incubated cells were treated with oxidative stress CellROX reagent (5 μM) for 15 min in the dark. PBS washed the cells thrice to wash out any superficially bound nanoformulation or dye. After, the media was changed, and cells were analysed for internalization using the ACEA NovoCyte 1000 FACS (ACEA Biosciences, Inc., San Diego, CA, U.S.A) [Citation35].
Fabrication of NPs-loaded Poloxamer 407 hydrogels
The cold approach was used to fabricate Poloxamer 407 hydrogels with a weight percentage of 40%. Suitable poloxamer 407 was distributed in water for 8 h until the polymer was dissolved entirely in an ice bath for hydrogel formations. It will take 12 h to dissolve the hydrogel at 4°C completely. Enough hydrogel and PLGA-NPs or non-encapsulated PDS (NE@PDS) were used to achieve the last concentration of 30% and 0.1%, individually, in the hydrogel. A similar ratio of NPs and water were used to fabricate hydrogels containing NPs (control, without drugs) and hydrogels containing control NPs (control, without NPs), respectively [Citation55].
Rheological investigation
All hydrogel rheological tests used the oscillatory Kinexus rheometer with a cone-plate shape. Oscillatory measurements and flow characteristics were performed on all hydrogels. The temperature was maintained at 32.5°C while frequency sweep analysis was conducted at 0.1 to 10 Hz. When doing a temperature sweep analysis, the temperature was varied from 10 to 50°C while the frequency was continuous at 1 Hz. For each nanoformulation, oscillatory measurements were taken to determine the elastic module (G′), viscous module (G′′), viscosity (η), and sol-gel temperature transition.
Nanoparticles morphological investigation
SEM analysis can be used for the morphological investigation of NPs and hydrogels. On the silicon substrate that had been previously treated, the NPs were dropped for this study (glow discharge). It was then placed on an SEM sample holder and coated with a 10 nm carbon layer in an argon environment. The system was set to 2kV and used a 5-mm spot size with an approximate 10-mm working distance to obtain the results. Images were analysed at various magnifications to determine the morphology and form of the NPs. The Gwyddion 2.54 was used to measure the size of 30 NPs and provide the size distribution.
Examination of in vitro PDS release and mathematic modeling
Encapsulated benzocaine, non-encapsulated benzocaine, and PDS@NPs were investigated in a two-compartment method for in vitro release profiles of benzocaine. A cellulose membrane with a molecular exclusion pore size of 1000 Da separates the compartment donor (1 mL) from the compartment acceptor (100 mL) in this model. Each time an aliquot was taken, the concentration of PDS was measured using high-performance liquid chromatography-tandem mass spectrometry (HPLC). Phosphate buffer 0.1 M pH 7.4 replaced the volume removed from the medium to sustain a similar volume during the investigation.
Examination of skin penetration across removed skin
A swab was used to remove the excess of the sample after the incubation period. The epidermis and dermis were heated for 1 min at 60°C to separate the two layers of skin. In a microtube, sodium fluoride (500 µL) solution was administered to the dermis while it was freezing in a straight posture for one day. 50-µM slices of the dermis were then sliced horizontally and combined in a sodium fluoride (500 µL) solution. The sample extracts were combined when the ethyl acetate extractions were mixed and evaporated under decreased pressure to get the acetonitrile solution. EC-C18 column (diameter: 3 × 50-mm, pore size: 1.95 μm, temperature: 30°C) was used to measure the PDS concentration in each sample. In the mobile phase, deionized water (solvent A) and acetonitrile (solvent B) with 0.1% formic acid were utilized. Agilent’s 1290 Infinity UHPLC was used with an AB Sciex QTrap 5500 hybrid linear ion-trap trinucleotide mass spectrometer to detect the metabolites. PDS transitions were 431.3 > 323.1, whereas internal standard transitions were 439.30 > 173.2 [Citation56].
Ex vivo permeation assays
All animals used in this study were kept under ethical considerations of the Institutional Animal Care and Use Committee (Ethical code #2020/48–568) of the Guangzhou Institute of Dermatology, Institute of Dermatology, Guangzhou Medical University, China.
TEWL is a reliable index to assess the skin barrier function in vivo [Citation57]. TEWL was determined from the skin utilizing DermaLab TEWL Probe (cyberDERM, Broomall, PA). The data were expressed in gm−2 h−1. Vertical Franz diffusion cells with a compartment donor of 1.7 cm2 diffusion area and a compartment acceptor (4.5 mL capacity) were used for skin samples in both intact and impaired situations and were constantly agitated at 32.5°C. The sinking state was maintained throughout the experiment using phosphate buffer 0.1 M, pH 7.4 [Citation58]. The excised ears were histologically examined by staining the vertical slices with Hematoxylin and Eosin (H&E). The mice’s ears were removed after 4 hours of therapy. The ears were immersed in paraffin and cut into tiny slices (0.005 mm) after being treated with 10% formaldehyde. After staining the skin slices with H&E, two pathologists performed a double-blind microscopic inspection and photographed the results to look for oedema and epidermal disruption. A binocular microscope with an image analyser was used to take the photomicrographs [Citation59].
Results and discussion
Characterization of the nanoparticles
The pharmacokinetic profile of drugs can be altered using a nano-based drug delivery technology, which has been widely explored [Citation60]. The therapeutic index of nano-based drug delivery systems can be improved even further by inclusion into hydrogels creating hybrid biomaterials. Chitosan (CS)-coated PLGA nanoparticles encapsulated prednisolone (PDS) and co-encapsulated to poloxamer hydrogel were established in this study for chronic actinic dermatitis (CAD) (). PDS@NPs and CTL@NPs were tested for their physical and chemical characteristics and colloidal stability. summarizes the physical and chemical parameters of NPs with and without PDS, including the particle size, polydispersity index (PDI), zeta-potential, particles concentrations, and encapsulation efficiency of the NPs.
Figure 1. Graphical illustration of the chitosan (CS)-coated PLGA nanoparticles encapsulated prednisolone (PDS) and co-encapsulated to poloxamer hydrogel to enhance the treatment of chronic actinic dermatitis (CAD).
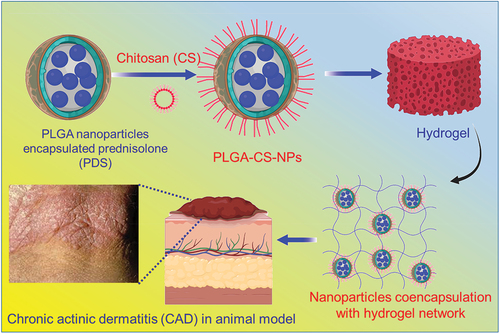
Figure 2. Nanoparticles characterization. a and c) Scanning electron microscopy of PLGA nanoparticles formulations. a) CTL@NPs (Control NPs). c) PLGA nanoparticles loading prednisolone (PDS). b and d) Respective Size distribution analysis by DLS measurement. e-f) Assessment of chitosan-coated onto PLGA-NPs surface determined by zeta potential.
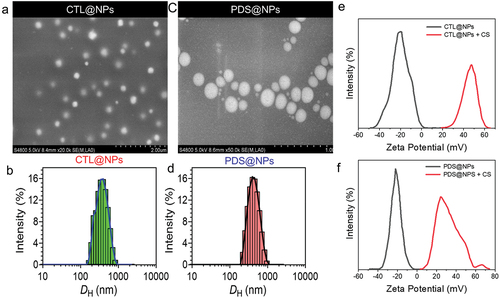
show that for CTL@NPs and PDS@NPs, the mean NPs diameter determined by DLS was 412.4 ± 5.4 nm and 415.2 ± 6.2 nm, respectively. In contrast, the particle size of both NPs assessed by NTA was less than the particle size reported by DLS for both samples. Both DLS and NTA may determine the diffusion coefficient of NPs. However, DLS verifies the diffusion coefficient of NPs by reading the modification of scattered light, whereas NTA estimates the diffusion coefficient of specific nanoparticles. In addition, the polydispersity index for both nanoformulations was approximately 0.2, and the potential was over 21 mV, indicating that the nanocarrier system was stable. The NTA concentrations of NPs suspension were 1014 particles/mL for nanoformulation. Due to the high affinity between PDS and the PLGA-NPs oily core, which was made up of the Myritol 318, a high encapsulation ratio (99.1 ± 0.03%) was attained in the experiment. Nanoformulations were also tested for their ability to coat the NPs by taking potential measurements before and after the addition of CS in the nanoformulation. After coating with chitosan, the NPs potential graph was shown in .
Unmodified PLGA-NPs had a negative potential of −23.1 ± 0.5 mV for CTL@NPs and −21.9 ± 0.6 mV for PDS@NPs, respectively (). On the surface of NPs, the carboxyl end groups of PLGA molecules contribute to the negative potential. A positive potential was observed for CTL@NPs and PDS@NPs when CS was added (), showing CS-coated PLGA-NPs. The size distribution of control NPs and PDS@NPs does not change significantly (). SEM was employed to explore further the shape and size distribution of both CTL@NPs and PDS@NPs (). It was discovered through morphological analysis that NPs are perfectly round, with a very smooth surface. Due to the lack of water around the NPs, the mean SEM diameter for CTL@NPs was 135 ± 18 nm and for PDS@NPs was 150 ± 13 nm. In terms of diameter, the PLGA-NPs had a range of 190 to 220 nm, a positively charged potential (+18 mV), and a spherical shape [Citation61].
In vitro proliferation assay
The toxicity of nano-based drug delivery systems has long been controversial. Using toxicity tests, testing the toxicity of nano-based formulations on both target and non-target species is achievable. Because of their biocompatibility and biodegradability, PLGA and CS have long been considered safe alternatives. Thus, we examined PDS proliferation, unloaded NPs, and PDS@NPs in the primary human keratinocytes and fibroblasts for 48 h (). The concentration of NE@PDS that was harmful to both fibroblasts and keratinocytes was not tested. It was shown that NPs loaded with PDS or not had no harmful effects on the animals. Cell viability in both cell lines is unaffected by concentration within the same cell population. Based on this study’s findings, the biocompatibility of CS-coated PLGA-NPs was acceptable. PLGA-NPs were slightly unsafe at all doses, with cell viability above 80% at all concentrations [Citation62].
Reactive oxygen species (ROS) generation
Reactive oxygen species (ROS) can induce oxidative stress, which results in cells failing to maintain normal redox-regulated functions. Nanomaterials have been shown to cause ROS production, which plays a vital role in directly or indirectly genotoxicity. We used the CellROX Orange dye test, which uses flow cytometry to measure the levels of the superoxide anion, propionitrile anion, hydroxyl peroxide, and hydroxyl radical in primary human cells (keratinocytes and fibroblasts) to learn more about the oxidative effects of NPs. FGF and keratinocytes treated with PDS at all doses (5, 25, and 50 µg/mL) considerably enhanced ROS production compared to the untreated (). Furthermore, the ROS generated by the two kinds of cells is very identical. Kim et al. [Citation63] found a dose-dependent increase in mitochondrial ROS generation in HeLa cells treated with PDS, consistent with our findings. Unloaded and PDS@NPs enhanced ROS generation in both keratinocytes and fibroblasts, even though the MTT experiment did not interfere with NPs’ metabolic function. Positively charged NPs have been shown in the past to increase ROS. Although unloaded and PDS-loaded NPs increased the ROS generation, the NPs themselves will not be in direct contact with the skin as they will be immobilized in P407-based hydrogels, which likely will reduce these toxic effects.
Figure 4. ROS generation with PDS alone, control NPs (Ctl@nps), prednisolone-loaded NPs (Pds@nps), and nonencapsulated PDS (NE@PDS). a) primary human keratinocytes (KC) various concentrations (5, 25, and 50 μg/mL). C and D) primary human Fibroblasts (FB) various concentrations (5, 25, and 50 μg/mL).
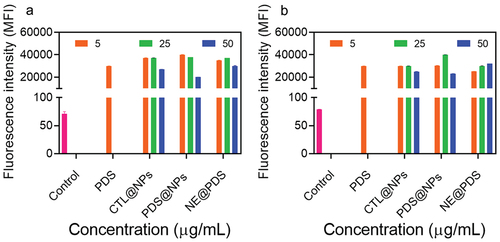
Rheological behaviour of hydrogels
Semisolid nanoformulation can benefit from the rheological study since it can deliver important details about their pharmaceutical characteristics and predict in vivo actions. Semisolid nanoformulation flow characteristics were previously known to alter the duration spent at the administration site and the pace at which the encapsulated drugs are released. PDS (P407-PDS), PLGA-NPs (named as P407-CTL@NPs), and PDS-loaded PLGA-NPs (denoted as P407-PDS@NPS) were used to study the rheological behaviour of poloxamer P407 hydrogels, which are known to be affected by the physico-chemical parameters of both the encapsulated drugs and the nanoparticle framework [Citation64].
Using a cone-plate rheometer, we measured storage (G′) and loss modulus (G′′), as well as the sol-gel transition temperature (Tsol-gel). As depicted in , the gel-solid transition temperature for hydrogels containing PDS (P407-PDS) and PDS-loaded PDS nanoparticles (P407-PDS@NPs) is displayed in contrast to hydrogels without PDS (P407-PDS). The Tsol-gel of thermosensitive design has been regarded as appropriate for skin applications if it is less than 32.5°C in temperature. A liquid preparation remains on the skin site if the transition sol-gel temperature exceeds 32.5°C, resulting in nanoformulation leaking from the administration site. With modest variations based on their composition, all nanoformulation demonstrated a transition sol-gel temperature lower than 32.5°C in all tests ().
Figure 5. Rheological evaluation of 30% poloxamer hydrogels as a function of temperature (10–50°C). a) Control hydrogel (P407), b) hydrogel containing PDS (P407-PDS), c) hydrogel containing PLGA-NPs (P407-CTL@NPs), d) hydrogel containing PDS-loaded PLGA-NPs (P407-PDS@NPs).
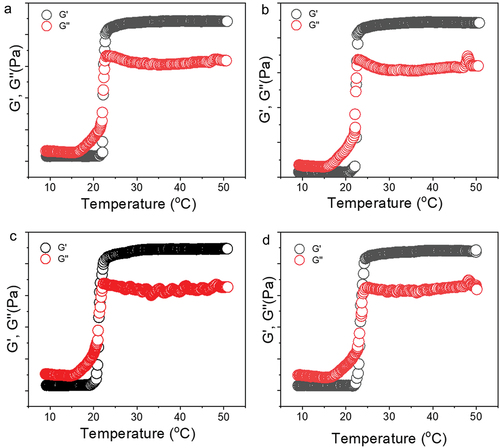
The storage moduli of P407–30%, P407-PDS, and P407-PDS@NPs are lower than the viscous moduli of P407-PDS@NPs at temperatures below 22.24°C, 21.2°C, or 23.35°C (). This indicates that the solution exhibits liquid-like qualities at this point. Alternatively, beyond this temperature, the moduli (G′) begin to rise above the vicious moduli (G′′), which indicates that the nanoformulation starts to display soft to challenging gel properties. Known as the sol-gel transition temperature (Tsol-gel), this point is followed by a fast rise in G′ and G′′ values until the temperature hits 24°C, at which point it begins to plateau. Another interesting finding from this study is that after G′ behaviour, the rheological characteristics of all formulations increased (), which is consistent with previous thermoresponsive poloxamer P407 hydrogel components. All hydrogel nanoformulation was suitable for skin sites since they all demonstrated thermosensitive behaviour [Citation65].
Figure 6. Viscosity evaluation of 30% poloxamer hydrogels as a function of temperature (10–50°C). a) Control hydrogel (P407), b) hydrogel containing PDS (P407-PDS), c) hydrogel containing PLGA-NPs (P407-CTL@NPs), d) hydrogel containing PDS-loaded PLGA-NPs (P407-PDS@NPs).
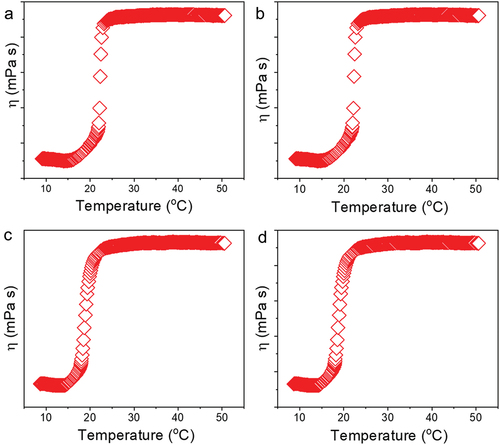
The storage moduli (G′) of all nanoformulation were more significant than the viscous moduli (G′′) in all the frequency limits examined, according to frequency sweep analysis (). The shows the viscosity (η) values of all hydrogels decreasing with an increase in shear rate (), indicating that this nanoformulation becomes less viscous, making it easier for them to flow and distribute over the skin. This further emphasizes the formulation’s adaptability. Pseudo-plasticity is desirable for topical formulations to ensure that the nanoformulation is evenly distributed into the skin. We observed the rheological patterns consistent with those reported by other researchers using NP-loaded poloxamer hydrogels. PDS-loaded or unloaded PLGA-NPs were shown to reduce hydrogel viscosity compared to plain hydrogels P407. For the G′/G′′ relationship, P407-PDS@NPs was shown to lower this value, but non-encapsulated PDS and bare NPs enhanced this association. However, the Tsol-gel of hydrogels containing non-encapsulated PDS or NPs does not vary from the hydrogel control in terms of Tsol-gel [Citation66].
Figure 7. Rheological evaluation of 30% poloxamer hydrogels as a function of frequency (0.1–10 Hz). a) Control hydrogel (P407), b) hydrogel containing PDS (P407-PDS), c) hydrogel containing PLGA-NPs (P407-CTL@NPs), d) hydrogel containing PDS-loaded PLGA-NPs (P407-PDS@NPs).
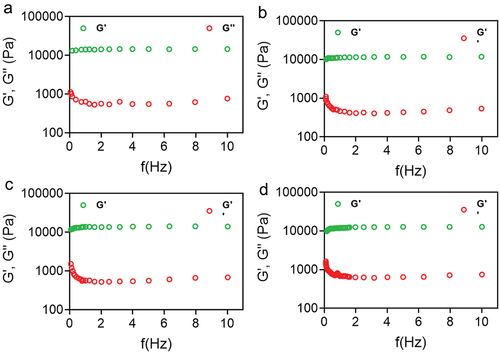
Figure 8. Rheological evaluation of 30% poloxamer hydrogels as a function of frequency (0.1–10 Hz). a) Control hydrogel (P407), b) hydrogel containing PDS (P407-PDS), c) hydrogel containing PLGA-NPs (P407-CTL@NPs), d) hydrogel containing PDS-loaded PLGA-NPs (P407-PDS@NPs).
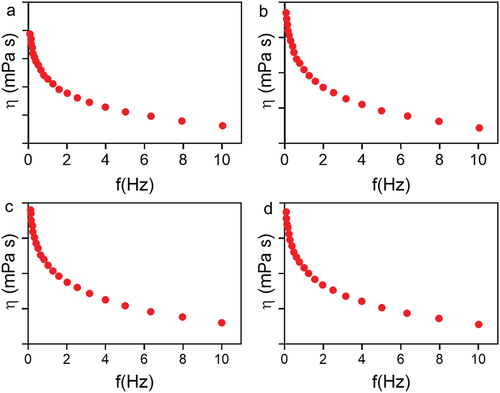
All hydrogel nanoformulation were studied in this study for their rheological properties, but we also looked at their flow curves (). The demonstrates the connection among the shear stress and the shear rate for the various nanoformulation. The shear stress improves as the shear rate increases and reduces as the shear rate lowers. For example, the overlapping of the upward and downward peaks indicated that hydrogels’ structural integrity was maintained in both situations. There is no thixotropic behaviour in all nanoformulation with flow indexes less than 1. They are consistent with prior studies on poloxamer hydrogels that have been done before.
Figure 9. Flow curvature changes the shear rate as a function of the shear stress of poloxamer 407 hydrogel at 32.5°C. a) Control hydrogel (P407), b) hydrogel containing PDS (P407-PDS), c) hydrogel containing PLGA-NPs (P407-CTL@NPs), d) hydrogel containing PDS-loaded PLGA-NPs (P407-PDS@NPs). e) Drug release of PDS from the PLGA-NPs and NPs incorporated into hydrogels.
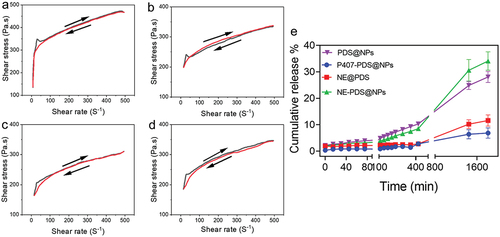
In vitro PDS release
Measurements were done in vitro utilizing the Franz cell set up to study the in vitro release of PDS@NPs and PDS@NPs integrated into hydrogels (e.g. P407-PDS and P407-PDS@NPs). It’s easy to see in how different nanoformulation PDS release characteristics change over time. The water solubility of PDS (29 mg/mL) meant that the non-encapsulated PDS (control group) had to be emulsified with PVA. The shows that the cumulative release of non-encapsulated PDS had a prolonged profile with a drug release rate of 10.1% to 1.9% during 30 h. We formed micelles during the emulsification with PVA, which may be regarded as a release method for PDS. PDS emulsified with PVA, on the other hand, when integrated into poloxamer hydrogels (), is released at a rate of 30 1.6% after 30 h, which is three times greater than that of pure PDS. Water absorption by hydrogels may have contributed to the rapid drug release and probable interactions between P407 and PVA that have destabilized the structural organization of PVA micelles, explaining the significant increase. For PDS@PLGA-NPs, the maximum release rate in the first hour was 3.2 ± 0.06% of PDS (). PDS was discharged at a rate of 25.5 ± 1.1% after 30 h of testing. On the other hand, compared to PDS@NPs, the release of PDS was significantly reduced following the integration of PDS@NPs into the poloxamer hydrogel (). In the first hour, 2 ± 0.06% of PDS was released, 1.6-fold more diminutive than the PDS@NPs. After 30 h, NPs placed with P407 hydrogels released fewer than 5% of their PDS.
Patients with skin disorders are more likely to develop a contact allergy to corticosteroids. Corticosteroids such as triamcinolone acetonide, flunisolide, dipropionate, and beclomethasone are less potent than PDS, a high-effectiveness non-halogenated corticoid with superior local action. PDS has also been added to the European standard for corticosteroid sensitive patch tests since it is a suitable indicator for another corticoid allergic reaction due to its physicochemical features. Corticosteroid PDS was the most susceptible to female and male participants evaluated in a prior study examining contact hypersensitivity to corticoids in chronic inflammatory dermatosis candidates [Citation67]. Because the breakdown product of PDS binds to arginine, hypersensitivity might occur.
Although PDS is a corticoid with significant anti-inflammation action, its usage for the therapy of CAD is limited owing to its adverse effects. Because of this, nanoformulations that minimize their negative effects and boost their pharmacological activity are needed to make them more accessible. It was possible in this study to control the drug release profile by using PDS nanoencapsulation in hybrid systems (hydrogel+NPs). This also allows for unconventional molecules, such as PDS, to treat CAD despite its known side effects. It is possible to combine more than one or more drugs in a single polymeric delivery frame to increase the pharmacological response of patients with skin disorders by utilizing nanoencapsulation. However, co-encapsulation is a promising method of treating skin disorders. Still, this procedure is complicated because drugs contend for the cellular matrix and may impair the encapsulation capacity of one or two drugs [Citation68].
Investigation of skin penetration
To have a local impact on the skin, a drug must first be released from the carrier, penetrate the stratum corneum, and then be partitioned to specific areas in the epidermis and dermis. This complex process has three key phases. These processes are directly influenced by the physicochemical characteristics of both the drugs and the medium. To determine the impact of nanoencapsulation on the PDS penetration profile into excised human skin, permeation tests were carried out. The epidermis of patients who received the non-encapsulated drugs (PDS) had the highest concentration of PDS (125.4 µg/mL). The dermis had the lowest concentration (23.2 µg/mL), with the highest and lowest concentrations of PDS occurring in the first and final layers, respectively. On the other hand, PDS concentrations in the two intermediate layers were virtually identical (). This indicates that hydrogel integration slows drug division among the tissue and nanoformulation, so the PDS is less concentrated in the epidermis layer (27.1 µg/mL) and the dermis layer (2.0 µg/mL) mL) when matched to PDS alone. Furthermore, minimal levels of PDS were found in the dermis’ deeper layers (). The epidermis layer’s encapsulated, and non-encapsulated PDS concentrations were 2.09- and 36-fold lower, respectively, in the skin treated with PDS-loaded nanoparticles (PDS@NPs) and PDS-loaded hydrogels (P407-PDS@NPs). In addition, when the hybrid system (P407-PDS@NPs) was used, only traces of PDS could be detected in the dermis. The drug’s prolonged release and impact on skin percutaneous modulation may reduce PDS concentration in the epidermis and dermis after incorporating it into the hydrogels, PDS-NPs, or dual systems (hydrogel+NPs).
Figure 10. PDS skin penetration assay. a) Quantity of PDS measured in the epidermis and (b-e) dermis after 6 h of topical function of various formulations.
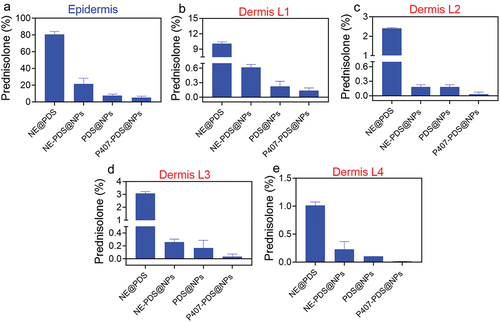
As displayed in , the excised skin tissues from the left and right application areas were histologically stained with H&E and Masson’s trichome (MTC). Histopathological analysis of H&E staining showed no epidermal atrophy. The normal structure of the epidermal and dermal layers was observed as nuclei were detected in the cells (). Purple-coloured nuclear chromatin material and pink-coloured collagen fibres were observed in the tissue. Additionally, melanin pigment is produced and stored in the epidermis and acts as a barrier.
Figure 11. H&E staining and MTS staining of skin damaged sites after treatment with control, NE@PDS, P407-CTL@NPs, PDS@NPs, and NE-PDS@NPs. b-c) PDS permeations from the NPs and PLGA-NPs are incorporated into hydrogels. The permeation assay was achieved using the vertical diffusion cell b) Full-width ear skin and c) damaged ear skin.
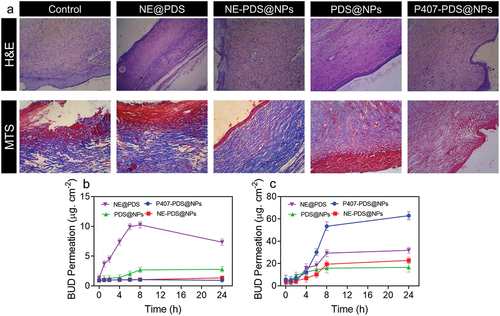
Glucocorticoid side effects, including thinned skin, might be less prevalent because of the slower absorption and lower dermal concentrations due to the gradual release. Patients with cardiovascular disease are more likely to benefit from using a hybrid system (hydrogel+NPs) to treat their condition since it is less likely to cause an immunological reaction if used for long periods. Patients with chronic inflammatory skin conditions were examined for their contact sensitivity to glucocorticosteroid (patch testing). PDS, clobetasol propionate and betamethasone valerate were shown to be the most sensitizing glucocorticosteroid in 78% of patients, followed by clobetasol and betamethasone. NPs in this study were too large to pass through the stratum corneum, which is the skin’s natural barrier. Because we employed excised human skin instead of follicles following surgical skin removal, this technique is no longer applicable because follicles are closed once the skin is surgically removed. However, it is worth noting that the nonpenetration of NPs in the skin is a good feature, as these NPs will only come into touch with the stratum corneum.
Ex vivo permeation
A drug must be applied to the skin with a weak epidermal layer to treat inflamed dermatoses, which is predicted to alter the drug’s ability to penetrate the skin. PLGA nanoparticles loaded with PDS were tested to penetrate intact and compromised skin barriers, as were PLGA nanoparticles loaded with PDS embedded in hydrogels. The shows the results of permeation studies. For all studied nanoformulation, more excellent drug absorption may be observed over time when the epidermal barrier is intact (). For the 24 h period, the maximum penetration value was found following treatment with non-encapsulated PDS (drug dispersed in an emulsion) and then with PDS loaded in the PLGA nanoparticles (PDS@NPs) As a result of the incorporation of both non-encapsulated and loaded polylactide nanoparticles (PLGA-NPs) into P407 hydrogels, substantial reductions in PDS penetration of 9.7-fold and 2.3-fold, respectively, were reported.
All nanoformulation investigated showed increased permeability as a function of time for the damaged skin barrier model. However, when both nanoemulsion and PLGA-NPs were added to the hydrogels, PDS penetration was enhanced. There was a 4-fold increase in PDS permeation when treated with 24 h and then the intact skin barrier, an 84.2-fold rise for P407-PDS@NPs, a 5-folds addition for PDS@NPs, and a 19.3-fold increase for P407-PDS@NPs permeation. When compared to PDS that had not been encapsulated, even after the skin barrier had been compromised, the combination of PDS with NPs reduced penetration through the skin considerably. We found that PDS penetration in intact human skin was reduced when NPs were added to the PDS solution, consistent with this study’s findings. Both undamaged and damaged ear skin were used to construct the linear regressions curve permeations parameters of all nanoformulation.
The PDS flow and penetrability co-efficient for PDS and PDS@NPs dropped considerably after the hydrogels were incorporated into the intact skin. Additional evidence suggests that only tiny quantities of PDS may penetrate the skin and enter the acceptor compartment in both hydrogel nanoformulation. PDS flow and permeability coefficient increased while lag time decreased when stratum corneum was injured in CAD patients’ skin. This indicates that the stratum corneum has been damaged, facilitating drug penetration. It is essential to point out the importance of PDS flux and permeability coefficient reduction in the case of hydrogels, which are particularly relevant for the treatment of CAD because of their low predicted percutaneous drug penetration, developing in possible diminished side effects.
Conclusions
Our investigation found that PDS-loaded PLGA-NPs had excellent colloidal characteristics and high drug EE. A lack of penetration of the stratum corneum means that the nanoparticles will not come into touch with any living cells, decreasing the potential for harm. The PDS penetration pattern was altered when encapsulated in hydrogels and NPs, reducing the number of drugs accessible in the skin, preventing percutaneous absorptions, and reducing the known side effects in CAD patients. NPs integrated inside poloxamer hydrogels released PDS with a prolonged release profile, increasing the drug’s therapeutic effectiveness. To the best of our knowledge, chronic actinic dermatitis (CAD) therapy with glucocorticoids, such as those used to treat acne and eczema, has not yet been reported using hydrogels containing PDS nanocapsules.
Authors’ contributions
Quan Chen, Huiyan Deng, Bihua Liang, Runxiang Li, Huaping Li, Yanan Ke supported synthesis, characterization, molecular and biochemical analysis, data curation, formal analysis, and validation. Prof. Huilan Zhu helped with supervising the research.
Ethics approval and consent to participate
All experimental procedures were carried out according to the protocol and international standards of care for animals to adhere to the requirements established by the ethical committee of the Guangzhou Institute of Dermatology; Institute of Dermatology, Guangzhou Medical University, Guangzhou -510,095, Guangdong, China (W-22-10254A1).
Availability of data and materials
The datasets used and/or analysed during the current study are available from the corresponding author on request.
Disclosure statement
No potential conflict of interest was reported by the authors.
Data availability statement
All data generated or analysed during this study are included in this submitted article. The raw data shall be made available upon request to the corresponding author.
Additional information
Funding
References
- Artz CE, Farmer CM, Lim HW. Chronic actinic dermatitis: a review. Curr Dermatol Rep. 2019;8(3):104–15. doi:10.1007/s13671-019-0263-z
- Verma L, Pratt M. A case report of therapeutically challenging chronic actinic dermatitis. SAGE Open Med Case Rep. 2019;7:2050313X19845235. doi:10.1177/2050313X19845235.
- Chen J-C, Lian C-H. Chronic actinic dermatitis in an old adult significantly improved by dupilumab. Photodermatol Photoimmunol Photomed. 2022;38(2):176–177. doi:10.1111/phpp.12731.
- Lei D, Lv L, Yang L, et al. Genome-wide analysis of mrna and long noncoding rna profiles in chronic actinic dermatitis. In: BioMed Research International [Internet]. editor, Papatheodorou K. 2017Vol. 2017 p. 7479523 doi;10.1155/2017/7479523.
- Huang CM, Asai Y. Chronic actinic dermatitis. Can Med Assoc J. 2018;190(10):E297–E297. http://www.cmaj.ca/content/190/10/E297.abstract
- EJ J, Anubhav M, Jessica W, et al. Tissue-specific contributions of Tmem79 to atopic dermatitis and mast cell-mediated histaminergic itch. Proc Natl Acad Sci [Internet]. 2018;115:E12091–E12100. doi: 10.1073/pnas.1814132115
- De Vuyst E, Salmon M, Evrard C, et al. Atopic Dermatitis Studies through in vitro Models [Internet]. Frontiers in Medicine. 2017;4:119. https://www.frontiersin.org/article/10.3389/fmed.2017.00119.
- Ramos Campos EV, PLDF P, Doretto-Silva L, et al. Trends in nanoformulations for atopic dermatitis treatment. Expert Opin Drug Delivery. 2020;17(11):1615–1630. doi:10.1080/17425247.2020.1813107.
- Chan JM, Xu C. Perspectives in micro-and nanotechnology for biomedical applications. World Scientific: 2016. 10.1142/p1085.
- Wang CX, Belsito DV. Chronic actinic dermatitis revisited. Dermatitis®. 2020;31(1):68–74. https://journals.lww.com/dermatitis/Fulltext/2020/01000/Chronic_Actinic_Dermatitis_Revisited.8.aspx
- Chen J, Li H, Zhu H. Successful treatment of chronic actinic dermatitis with dupilumab: a case report and review of the literature. Clin Cosmet Investig Dermatol. 2021;14:1913–1917. https://pubmed.ncbi.nlm.nih.gov/35002273
- SC-S H, Lan C-C. Tungsten lamp and chronic actinic dermatitis. Australas J Dermatol. 2017;58(1):e14–e16. doi:10.1111/ajd.12382
- Ichihashi M, Nakamura Y, Muto M, et al. A case of chronic actinic dermatitis that responded completely to treatment with oral colostrum-macrophage-activating factor (colostrum-MAF). Photodermatol Photoimmunol Photomed. 2019 april 29;35(4):290–292. https://pubmed.ncbi.nlm.nih.gov/30951213
- Wang T, Gong Y, Rong W, et al. Ultraviolet a rush hardening for chronic actinic dermatitis: pilot treatment outcomes. J Dermatol. 2021;48(3):385–388. doi:10.1111/1346-8138.15667.
- Sinha A, Pathania V, Sood A, et al. Mycosis fungoides with photosensitivity mimicking chronic actinic dermatitis. Indian Dermatol Online J. 2020;12(2):337–339. https://pubmed.ncbi.nlm.nih.gov/33959539
- Pawar M, Zawar V. Chronic actinic dermatitis: an only presenting manifestation of human immunodeficiency virus in a young Indian male. Indian J Sex Transm Dis AIDS. 2020 Nov 11;41(2):207–210. https://pubmed.ncbi.nlm.nih.gov/33817598
- Ma L, Zhang Q, Hu Y, et al. Evaluation of narrow band ultraviolet B phototherapy in the treatment of chronic actinic dermatitis in Chinese patients. Dermatologic Therapy. 2017;30:e12528. doi:10.1111/dth.12528.
- Paracha MM, Noor SM. METHOTREXATE IN CHRONIC ACTINIC. 2018;10:124–126.
- Ali K, Wu L, Lou H, et al. Clearance of chronic actinic dermatitis with dupilumab therapy in Chinese patients: a case series. Front Med (Lausanne). 2022;9:803692. https://pubmed.ncbi.nlm.nih.gov/35280879
- Majid I, Akhtar S. Tofacitinib in resistant chronic actinic dermatitis: a case series. Dermatologic Therapy. 2022;35(6):e15467. https://doi.org/10.1111/dth.15467
- Lahouel M, Ben Kahla M, Aounallah A, et al. Severe chronic actinic dermatitis treated successfully with Thalidomide. Photodermatol Photoimmunol Photomed. 2020;36(6):493–495. doi:10.1111/phpp.12588.
- Chandwani B, Lunge S, Sardesai V. Successful treatment of actinic reticuloid with nonsteroidal immunosuppressive drugs. Indian Journal Of Drugs In Dermatology. 2021;7(2):101.
- Teran VA, McHargue CA, Gru AA. Photodistributed Rash Progressing to Erythroderma: answer. Am J Dermatopathol. 2020;42(6):463–465. https://journals.lww.com/amjdermatopathology/Fulltext/2020/06000/Photodistributed_Rash_Progressing_to_Erythroderma_.16.aspx
- Nassim D, Alajmi A, Jfri A, et al. Apremilast in dermatology: a review of literature. Dermatologic Therapy. 2020;33:e14261. doi:10.1111/dth.14261.
- Samrat K, Chandraprabha MN, Hari Krishna R, et al. Synthesis, characterisation and in vivo wound healing activity of chemogenic sulphur nanoparticles. Mater Technol. 2022;37:3025–3040. doi:10.1080/10667857.2022.2115757.
- Shete MB, Deshpande AS, Shende P. Silybin-based herbal nanocarriers: an advancement in anticancer therapy. Mater Technol. 2022;37:2832–2852. DOI:10.1080/10667857.2022.2081286.
- Comba A, Cicek B, Comba B, et al. Investigation of in-vitro biocompatibility and in-vivo biodegradability of AM series Mg alloys. Mater Technol. 2022;37:2819–2831. doi:10.1080/10667857.2022.2081115.
- Singh J, Dwivedi A, Ray L, et al. PLGA nanoformulation of sparfloxacin enhanced antibacterial activity with photoprotective potential under ambient UV-R exposure. Int J Pharmaceut. 2018;541:173–187. https://www.sciencedirect.com/science/article/pii/S0378517318301091
- Singh S, Behl T, Sharma N, et al. Targeting therapeutic approaches and highlighting the potential role of nanotechnology in atopic dermatitis. Environ Sci Pollut Res. 2022;29(22):32605–32630. doi:10.1007/s11356-021-18429-8.
- Parekh K, Mehta TA, Dhas N, et al. Emerging Nanomedicines for the Treatment of Atopic Dermatitis. AAPS Pharm Sci Tech. 2021;22:55. doi:10.1208/s12249-021-01920-3.
- Tan S, Huang Y, Lan J, et al. Preparation and characterization of graphene oxide-Chitosan composite film for ultrathin hyaluronic acid hydrogels. Mater Technol. 2022;37:2692–2701. doi:10.1080/10667857.2022.2059045.
- Wang S, Zhang Y. A functional triboelectric nanogenerator based on the LiCl/PVA hydrogel for cheerleading training. Mater Technol. 2022;37:2752–2757. doi:10.1080/10667857.2022.2073117.
- Zhou W, Han F, Li C, et al. Gelatin/Polydopamine/Zn2+ composite microspheres-based cream gel for wound treatment. Mater Technol. 2022;37:2874–2884. doi:10.1080/10667857.2022.2084025.
- Hsiao C-Y, Yang S-C, Alalaiwe A, et al. Laser ablation and topical drug delivery: a review of recent advances. Expert Opin Drug Delivery. 2019;16:937–952. doi:10.1080/17425247.2019.1649655.
- Donnelly RF, Larrañeta E. Microarray patches: potentially useful delivery systems for long-acting nanosuspensions. Drug Discovery Today. 2018;23:1026–1033. https://www.sciencedirect.com/science/article/pii/S1359644617302337
- Vollono L, Falconi M, Gaziano R, et al. Potential of Curcumin in Skin Disorders [Internet]. Nutrients. Dermatology Unit, Department of “Medicina dei Sistemi”, University of Rome Tor Vergata, Via Montpellier, 1–00133 Rome, Italy; 2019. Available from: http://europepmc.org/abstract/MED/31509968.
- Todke P, Shah VH. Psoriasis: implication to disease and therapeutic strategies, with an emphasis on drug delivery approaches. Int J Dermatol. 2018;57(11):1387–1402. doi:10.1111/ijd.14047
- Zhang H, Zhao H, Zhao X, et al. Biocompatible Light Guide-Assisted Wearable Devices for Enhanced UV Light Delivery in Deep Skin. Adv Funct Mater. 2021;31(23):2100576. doi:10.1002/adfm.202100576.
- Krishnan V, Mitragotri S. Nanoparticles for topical drug delivery: potential for skin cancer treatment. Adv Drug Delivery Rev. 2020;153:87–108. https://www.sciencedirect.com/science/article/pii/S0169409X20300442
- Arafa MG, Girgis GNS, El-Dahan MS. chitosan-Coated PLGA Nanoparticles for Enhanced Ocular Anti-Inflammatory Efficacy of Atorvastatin Calcium. Int J Nanomed. 2020;Volume 15:1335–1347.
- Miguel SP, Moreira AF, Correia IJ. Chitosan based-asymmetric membranes for wound healing: a review. Int j biol macromol. 2019;127:460–475.
- Fredenberg S, Wahlgren M, Reslow M, et al. The mechanisms of drug release in poly (lactic-co-glycolic acid)-based drug delivery systems—a review. Int J Pharmaceut. 2011;415:34–52.
- Badran MM, Alomrani AH, Harisa GI, et al. Novel docetaxel chitosan-coated PLGA/PCL nanoparticles with magnified cytotoxicity and bioavailability. Biomed Pharmacother. 2018;106:1461–1468. https://www.sciencedirect.com/science/article/pii/S0753332218328063
- Baghirova L, Kaya Tilki E, Öztürk AA. Evaluation of Cell Proliferation and Wound Healing Effects of Vitamin a Palmitate-Loaded PLGA/Chitosan-Coated PLGA Nanoparticles: preparation, Characterization, Release, and Release Kinetics. ACS Omega. 2023;8:2658–2668. doi:10.1021/acsomega.2c07232.
- Soorbaghi FP, Isanejad M, Salatin S, et al. Bioaerogels: synthesis approaches, cellular uptake, and the biomedical applications. Biomed Pharmacother. 2019;111:964–975.
- Suh J-K, Matthew HWT. Application of chitosan-based polysaccharide biomaterials in cartilage tissue engineering: a review. Biomaterials. 2000;21:2589–2598.
- Shariatinia Z, Jalali AM. Chitosan-based hydrogels: preparation, properties and applications. Int j biol macromol. 2018;115:194–220.
- ZHOU Z, YE J, CHEN L, et al. Simultaneous Determination of Ropivacaine, Bupivacaine and Dexamethasone in Biodegradable PLGA Microspheres by High Performance Liquid Chromatography. Yakugaku Zasshi. 2010;130:1061–1068.
- Edlich A, Volz P, Brodwolf R, et al. Crosstalk between core-multishell nanocarriers for cutaneous drug delivery and antigen-presenting cells of the skin. Biomaterials. 2018;162:60–70. https://www.sciencedirect.com/science/article/pii/S0142961218300784
- Giriraj K, Mohamed Kasim MS, Balasubramaniam K, et al. Various coordination modes of new coumarin Schiff bases toward Cobalt (III) ion: synthesis, spectral characterization, in vitro cytotoxic activity, and investigation of apoptosis. Appl Organomet Chem. 2022;36(3):e6536. doi:10.1002/aoc.6536.
- Sathiya Kamatchi T, Mohamed Subarkhan MK, Ramesh R, et al. Investigation into antiproliferative activity and apoptosis mechanism of new arene Ru(ii) carbazole-based hydrazone complexes. Dalton Trans. 2020;49(32):11385–11395. doi:10.1039/D0DT01476A.
- Kalaiarasi G, Mohamed Subarkhan M, Fathima Safwana CK, et al. New organoruthenium(II) complexes containing N, X-donor (X = O, S) heterocyclic chelators: synthesis, spectral characterization, in vitro cytotoxicity and apoptosis investigation. Inorganica Chimica Acta. 2022;535:120863. https://www.sciencedirect.com/science/article/pii/S0020169322000755
- Wang Y, Jin J, Shu L, et al. New Organometallic Ruthenium(II) Compounds Synergistically Show Cytotoxic, Antimetastatic and Antiangiogenic Activities for the Treatment of Metastatic Cancer. Chem Eur J. 2020;26(66):15170–15182. doi:10.1002/chem.202002970.
- Pilliadugula R, Haribabu J, Mohamed Subarkhan MK, et al. Effect of morphology and (Sn, Cr) doping on in vitro antiproliferation properties of hydrothermally synthesized 1D GaOOH nanostructures. J Sci. 2021;6:351–363. https://www.sciencedirect.com/science/article/pii/S2468217921000174
- Schmolka IR. Artificial skin I. Preparation and properties of pluronic F-127 gels for treatment of burns. J Biomed Mater Res. 1972;6(6):571–582. doi:10.1002/jbm.820060609
- Coll S, Monfort N, Matabosch X, et al. Budesonide use and misuse in sports: elimination profiles of budesonide and metabolites after intranasal, high-dose inhaled and oral administrations. Drug Test Anal. 2020;12(5):629–636. doi:10.1002/dta.2678.
- Campos EVR, Proença PLF, da CT, et al. Hydrogels Containing Budesonide-Loaded Nanoparticles to Facilitate Percutaneous Absorption for Atopic Dermatitis Treatment Applications. ACS Applied Polymer Materials. 2021;3(9):4436–4449. doi:10.1021/acsapm.1c00021.
- Simonsen L, Fullerton A. Development of an in vitro Skin Permeation Model Simulating Atopic Dermatitis Skin for the Evaluation of Dermatological Products. Skin Pharmacol Physiol. 2007;20(5):230–236. https://www.karger.com/doi/10.1159/000104421
- Rubio L, Alonso C, López O, et al. Barrier function of intact and impaired skin: percutaneous penetration of caffeine and salicylic acid. Int J Dermatol. 2011;50:881–889. doi:10.1111/j.1365-4632.2010.04819.x.
- Kamaly N, Yameen B, Wu J, et al. Degradable Controlled-Release Polymers and Polymeric Nanoparticles: mechanisms of Controlling Drug Release. Chem Rev. 2016;116(4):2602–2663. doi:10.1021/acs.chemrev.5b00346.
- Patra JK, Das G, Fraceto LF, et al. Nano based drug delivery systems: recent developments and future prospects. J Nanobiotechnol. 2018;16(1):71. doi:10.1186/s12951-018-0392-8.
- Grillo R, V DF, Querobino SM, et al. Influence of hybrid polymeric nanoparticle/thermosensitive hydrogels systems on formulation tracking and in vitro artificial membrane permeation: a promising system for skin drug-delivery. Colloids Surf B Biointerfaces. 2019;174:56–62. http://www.sciencedirect.com/science/article/pii/S0927776518307471
- Kim S-R, Song J-H, Ahn J-H, et al. Antiviral and anti-inflammatory activity of budesonide against human rhinovirus infection mediated via autophagy activation. Antiviral Res. 2018;151:87–96.
- Lee CH, Moturi V, Lee Y. Thixotropic property in pharmaceutical formulations. JControlled Release. 2009;136:88–98.
- Alves T, Souza J, Rebelo M, et al. Formulation and evaluation of thermoresponsive polymeric blend as a vaginal controlled delivery system. J Sol Gel Sci Techn. 2018;86(3):536–552. doi:10.1007/s10971-018-4662-6
- Freitas Mariano KC, Do Nascimento MH M, Querobino SM, et al. Influence of chitosan-tripolyphosphate nanoparticles on thermosensitive polymeric hydrogels: structural organization, drug release mechanisms and cytotoxicity. Int J Polym Mater Polym Biomater. 2020;69(9):592–603. doi:10.1080/00914037.2019.1596909.
- Kot M, Bogaczewicz J, Kręcisz B, et al. Contact allergy in the population of patients with chronic inflammatory dermatoses and contact hypersensitivity to corticosteroids. Advances in Dermatology and Allergology/Postępy Dermatologii i Alergologii. 2017;34:252–259. doi:10.5114/ada.2017.67848.
- Takeuchi I, Suzuki T, Makino K. Skin permeability and transdermal delivery route of 50-nm indomethacin-loaded PLGA nanoparticles. Colloids Surf B Biointerfaces. 2017;159:312–317. https://www.sciencedirect.com/science/article/pii/S0927776517305192