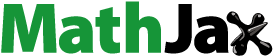
ABSTRACT
The aim of this study is to control the release of methotrexate (MTX) from a cellulose-based molecularly imprinted polymer (MIP) film containing melanin using near-infrared (NIR) laser irradiation. Methacrylic acid (MAA) as a functional monomer for the recognition of MTX was grafted to carboxymethyl cellulose through free radical polymerisation. Templating molecule MTX was then attached via hydrogen bonding to MAA. Melanin was then added to the MIP film. The MIP film selectively recognised MTX over folic acid (with a similar structure to MTX). After 808 nm NIR laser irradiation at 0.7 W/cm2 for 10 min, the surface temperature of the MIP film rose above 60°C and the MTX release and permeation rates increased rapidly. After release of the MTX from the MIP film, more uniformly sized pores were observed than seen in the non-molecularly imprinted film. These results indicate that the MIP film is suitable for transdermal drug delivery systems.
Introduction
The treatment of diseases requires minimising the side effects of drugs and continuously maintaining their efficacy at the disease site, so novel drug delivery systems are continually being sought [Citation1]. Unfortunately, orally administered drugs can be inactivated by the acidic environment of the stomach and various enzymes secreted into the gastrointestinal tract. To overcome these problems, recent attention has been focused on the development of transdermal drug delivery system (TDDS) as a non-invasive drug administration route [Citation2]. Furthermore, TDDS has the advantages of reducing side effects, improving patient compliance by reducing the number of administrations, continuously releasing the drug to maintain the plasma concentration, and avoiding the first-pass effect [Citation3,Citation4]. Many attempts at developing TDDSs have been concentrated on manufacturing patches using natural biodegradable polymers. Among them, cellulose (a polysaccharide that is the most abundant polymer produced in nature) has a structure in which hundreds to thousands of D-glucose units are linked by β(1→4) glycosidic bonds to make rigid and mechanically stable chains. It also has excellent characteristics such as biocompatibility and biodegradability, non-toxicity, affordability, and recyclability. Therefore, cellulose is suitable as an effective polymer backbone for TDDS [Citation5,Citation6]. Cellulose derivates such as hydroxypropyl cellulose, hydroxypropyl methylcellulose, and carboxymethyl cellulose have been widely used for controllable drug release [Citation7].
Methods to control drug release at a specific site using external stimuli such as light, pH, redox, and heat from responsive biomaterials to increase the specificity and efficiency of the drug and to minimise the potential side effects and toxicity are being continually sought [Citation8–10]. Among the various stimuli, light is non-invasive and temporally and spatially controllable. In particular, near-infrared (NIR) light has excellent tissue penetration ability and is very safe to use [Citation11]. Thus, biomaterials that respond to NIR light are widely used for drug release. For example, we reported that drug release from alginate hydrogels could be induced by using the photothermal effect in which heat is generated under NIR irradiation [Citation12]. Photothermal agents that absorb light and release heat include carbon nanotubes, graphene, and gold nanoparticles [Citation13–15]. Although these materials have good efficiency in converting light into heat, their biomedical application in vivo is limited because they have poor biocompatibility. Thus, the search for photothermal agents with excellent biocompatibility has recently led to melanin being in the spotlight. Melanin with excellent biodegradability and biocompatibility is a pigment produced by melanocytes. In particular, melanin has a chromophore that effectively absorbs a wide range of light and converts it into heat [Citation16–18].
Molecularly imprinted polymers (MIPs) are good candidates for a specific and effective drug delivery [Citation19]. The use of MIP in drug delivery systems (DDSs) has an attractive advantage such as high specificity to recognise target biomolecules and ability to introduce stimuli-responsiveness [Citation20,Citation21]. MIP-based TDDSs can rapidly release drugs in response to a stimulus (e.g. light, pH, heat, etc.) [Citation22,Citation23]. In particular, drug release from MIP-based hydrogels can be modulated by elevating the temperature, leading to disruption of the hydrogen bonds between the template (drug) and the polymer’s functional groups [Citation24]. Therefore, when a MIP-based TDDS contains a photothermal agent, light irradiation can induce localized and temporized drug release from a specific site.
In the present study, a cellulose-poly(methacylic acid)-based MIP film containing melanin was developed for the effective release rate of MTX under NIR 808 nm laser irradiation. MTX inhibits working of the enzyme dihydrofolate reductase in DNA synthesis and is used to treat cancers such as skin, breast, and head and inflammatory diseases such as rheumatoid arthritis [Citation25]. Methacrylic acid (MAA) as a functional monomer for the molecular recognition of MTX was copolymerised with carboxymethylcellulose (CMC) (). CMC is one of the cellulose derivatives and is used widely in various applications such as edible coating films on food, hydrogels in agriculture, and drug delivery in pharmaceutics [Citation26]. The main reasons for the use of CMC are its transparency, good film-forming characteristics, large mechanical strength, non-toxicity, and biodegradability [Citation27,Citation28]. To investigate drug release profiles by photothermal effect, melanin was physically added to the CMC-polyMAA MIP film, and MTX was attached to MAA via hydrogen bonding. The selective adsorption of MTX to the MIP film was evaluated using folic acid (FA), which is structurally similar to MTX. In addition, to evaluate the light-to-heat conversion characteristics of the MIP film, temperature changes were observed upon irradiating with an NIR laser at various intensities. Finally, the drug release behaviour from the MTX@MIP film upon NIR laser irradiation was evaluated at pH 7.4 and 5.5.
Materials and methods
Materials
Carboxymethyl cellulose (CMC, molecular weight 90 kDa, CAS No.: 9004-32-4), potassium persulphate (KPS, CAS No.: 7727-21-1), methacrylic acid (MAA, CAS No.: 79-41-4), methotrexate (MTX, CAS No.: 59-05-2), ethylene glycol dimethacrylate (EGDMA, CAS No.: 97-90-5), melanin (eumelanin, CAS No.: 8049-97-6), acetonitrile (CAS No.: 75-05-8), and folic acid (FA, CAS No.: 59-30-3) were purchased from Sigma-Aldrich Co. (St. Louis, U.S.A.). Ethanol (CAS No.: 64-17-5) and dimethyl sulphoxide (CAS No.: 67-68-5) were obtained from Dukan-Reagents Co. (Ansan, South Korea). Phosphate buffered in saline (PBS, CAS No.:) was purchased from LONZA (Basel, Switzerland). PermeaPad barriers were obtained from InnoME (Espelkamp, Germany).
Preparation of the CMC-polyMAA MIP film
CMC-polyMAA was prepared in two steps using a modified free radical polymerization method reported previously [Citation29]. Briefly, CMC (62.5 mg) was dissolved in 5 mL of distilled water in a stirrer adjusted to 60 C and degassed. The temperature of the stirrer was then re-adjusted to 70°C, and 5 ml of KPS solution (0.25 mg/mL) and MAA (91.6 mg) were sequentially added, followed by sufficient stirring until the solution became opaque. To remove excess KPS and MAA, the solution was dialysed through a dialysis membrane (MWCO 12,000 Da, Sigma-Aldrich, St. Louis, MO, U.S.A.) for 3 days and then freeze-dried (freeze dryer, Ilshinbiobase Co., Ltd., Dongducheon, Korea). After dissolving the resulting CMC-polyMAA (62.5 mg) in 5 mL of distilled water, MTX (5 mg) was added, followed by sonication in a water bath sonicator (NXP-1002, Hansol Tech Co. Ltd., Gimpo, Korea) for a sufficient time to allow imprinting between MTX and MAA in CMC-polyMAA. After degassing the mixture, EGDMA (2.1 mg) was added to promote crosslinking, followed by stirring for 1 h. The resulting mixture was dialysed for 3 days to remove excess MTX and EGDMA. After dispersing the melanin (1 mg) in solution, the mixture was dried for 24 h through a casting method in an oven at 40°C to form a film. Finally, to remove the template molecule MTX, the film was placed in a mixture of ethanol, and acetonitrile (8:2, v/v) five times at room temperature until MTX could no longer be detected using a UV spectrophotometer (T60U, PG Instruments Limited, UK) at 345 nm. A film (0.5 × 0.5 cm2) was soaked in MTX solution to recognize MTX with the film. As a control, a NIP film on which MTX was not imprinted was prepared in the same way without the last step.
Characterization of the CMC-polyMAA MIP film
Chemical functional groups in CMC, MAA, EGDMA, MTX, and the CMC-polyMAA MIP film were characterised by using Fourier transform infrared (FT-IR) spectroscopy (VERTEX70, Bruker Co. Ltd., MA, U.S.A.) at the range of 4500–500 cm−1. To observe the surface of CMC-polyMAA MIP film, field emission-scanning electron microscopy (FE-SEM) was performed using a Sigma 500 system (Carl ZEISS Co., Ltd., Oberkochen, Germany). In addition, elemental analysis using an electrical-dispersive X-ray spectroscope (EDS) (ULTIM MAX 100, Oxford Instruments, Abingdon, United Kingdom) coupled to FE-SEM was performed to assess the presence of MTX in the film. The acceleration voltage of 10 kV was used for images and EDS analyses, respectively.
Adsorption of MTX onto the CMC-polyMAA MIP film
To achieve adsorption of MTX, a MIP film (0.5 × 0.5 cm2) was soaked in MTX solution (0.02–0.80 mg/mL in dimethyl sulphoxide) under shaking for 12 h. To confirm the selective adsorption of MTX, FA having a similar chemical structure to MTX was adsorbed on the film using the same method. The concentrations of MTX and FA before and after adsorption were measured using a UV–vis spectrophotometer at 345 nm for MTX and 280 nm for FA. The amounts of MTX and FA adsorbed [Q, mg/g (1)] were calculated using the following equation [Citation30]:
where C0 and Ceq are the initial and equilibrium concentrations (mg/mL), respectively; m is the mass of the film (g), and V is the solution volume (mL).
In addition, the imprinting factor [IF (2)] was calculated using the following equation [Citation31]:
where Qm and Qn (mg/mL) are the adsorped concentrations of MTX and FA on the MIP and NIP films, respectively.
Photothermal effect of CMC-polyMAA MIP film
To investigate the photothermal properties of the CMC-polyMAA MIP film, a piece (0.5 × 0.5 cm2) was fixed to the holder and irradiated with an NIR 808 nm laser (LDH840-30-3, Laserlab Co., Ltd., Seoul, Korea) at a distance of 5 cm from the film for 10 min at various intensities (0.5, 0.7 or 0.9 W/cm2). The temperature change over time was observed using an infrared camera (C2, FLIR System Inc., Sweden). To confirm the effect of repetitive photothermal conversion on the CMC-polyMAA MIP film, a piece was fixed as mentioned above and irradiated with the NIR 808 nm laser 0.7 W/cm2 five times (10 min on and 5 min off). The temperature change during each cycle was observed using an infrared camera.
The release profile of MTX from the CMC-polyMAA MIP film
A modified Franz diffusion cell method was conducted to investigate the influence of the photothermal effects on the MTX release from CMC-polyMAA MIP film as shown in [Citation31]. MTX release testing was performed in 10 mL of PBS at pH 7.4 or 5.5. A dialysis membrane used as a permeation barrier was fixed between the donor (the CMC-polyMAA MIP film (0.5 × 0.5 cm2)) and the acceptor (a compartment filled with PBS buffer). After irradiation with the NIR 808 nm laser at 0.7 W/cm2 for 10 min (once or 4 times) or not irradiated under stirring, the absorbance by the PBS buffer in the acceptor compartment was measured at 345 nm using a UV spectrophotometer at regular time intervals. The released amount of MTX was calculated by using a standard curve for MTX.
Statistical analysis
All experimental data were calculated in triplicate (n = 3) and reported as the mean ± standard deviation (mean ± S.D.). Statistical analysis was performed by using student t-tests. A p value < 0.01 was considered statistically as a significant difference.
Results and discussion
FT-IR analysis
Changes in the chemical structure of a material can be determined by the shape, disappearance, position, and variations in shape and intensity of particular absorption bands in an FT-IR spectrum. The chemical functional groups in the CMC-polyMAA MIP film were investigated through FT-IR analysis. Thereby, the composition of the MIP film and coupling between the MIP film and MTX could be confirmed [Citation32]. shows FT-IR spectra for MAA, EGDMA, a CMC film, and a CMC-polyMAA MIP film. The FT-IR spectrum for CMC shows characteristic peaks at 1096 cm−1 (-C-O stretching), 1675 cm−1 (-C=O stretching), and 3488 cm−1 (-O-H stretching). Meanwhile, MAA shows characteristic bands at 1202 cm−1 (-C-O stretching), 1632 cm−1 (-C=C- stretching), and 1690 cm−1 (-C=O stretching), respectively.
Field emission-SEM and EDS analyses
The surface of the CMC-polyMAA MIP film was observed using FE-SEM. shows the SEM images of the surfaces of the CMC, NIP, and CMC-polyMAA MIP films before and after MTX recognition. Whereas the surfaces of the CMC and NIP films are smooth, the presence of cavities can be seen on the surface of the CMC-polyMAA MIP film before MTX recognition, which were filled with MTX after recognition.
Figure 4. (a) Field emission-SEM images of the CMC, NIP, MIP, and MTX@MIP films. (b) EDS spectra for the MIP and MTX@MIP films.
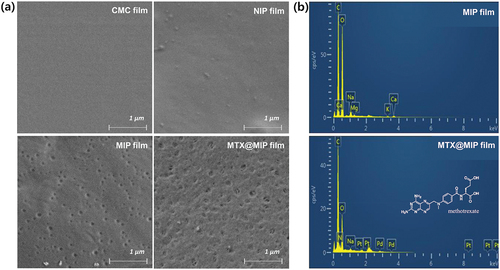
EDX analysis was performed to investigate the elements in the CMC-polyMAA MIP film before and after MTX recognition. The EDX spectra in show carbon and oxide peaks without MTX recognition and a nitrogen peak afterwards. These results indicate that the CMC-polyMAA MIP film recognised MTX and can contain the drug therein.
Adsorption of MTX on the MIP film
One of the most important factors for a MIP film is its specific adsorption capacity for the drug of interest [Citation33]. shows the adsorption capacity obtained at 12 h after loading MTX onto the MIP film. The amount of MTX adsorbed on the MTX@MIP film increased rapidly with increasing MTX concentration in the immersion solution. On the other hand, the adsorption of MTX on the NIP film without MTX binding sites did not significantly increase even as the concentration of MTX was increased. When the concentration of MTX was 0.8 mg/mL, the amounts of MTX adsorbed on the MIP and NIP films were 10.4 0.3 and 4.0
0.1 mg/g, respectively. In addition, the imprinting factor values for the MIP film versus the NIP film for MTX and FA were 2.62 and 1.06, respectively. To confirm the specific adsorption of MTX, FA with a similar structure was used to evaluate the adsorption capacity under the same conditions. Only a small amount of FA was adsorbed on the MIP and NIP films due to physical non-specific binding. Additionally, because the melanin physically contained in the films has a high affinity for polycyclic aromatic compounds, MTX and FA can be thought to be adsorbed on the MIP or NIP film [Citation34,Citation35]. These results indicate that unlike the NIP film, the MIP film specifically recognises MTX.
Heat generation in the MTX@MIP film upon NIR laser irradiation
Melanin absorbs light over a wide range of wavelengths. In particular, it absorbs the near-infrared light, thereby generating heat via the photothermal effect [Citation36]. It was hypothesised that heat generation by melanin in the MTX@MIP film upon laser irradiation would change the physical structure of the film, thereby releasing MTX. show photographs of the MTX@MIP film taken with a thermal camera. The temperature of the film increased rapidly 1 min after 808 nm laser irradiation. The temperature of the film at 1 min post-irradiation was 64.6, 71.8, and 85.8°C when irradiated at 0.5, 0.7, and 0.9 W/cm2, respectively (). In addition, repeated laser irradiation-initiated heat generation in the film produced the same results without loss of heat (). These results indicate that MTX@MIP films can efficiently generate heat within a short period of time after 808 nm laser irradiation and can generate heat repeatedly.
Figure 6. (a) IR thermal camera images of the MIP film irradiated with an 808 nm laser at (a) 0.5, (b) 0.7, and (c) 0.9 W/cm2 power densities for 10 min. Temperature plots (d) according to time for each laser intensity and (d) after repeated irradiation of the MIP film for five cycles upon 808 nm laser irradiation at 0.7 W/cm2.
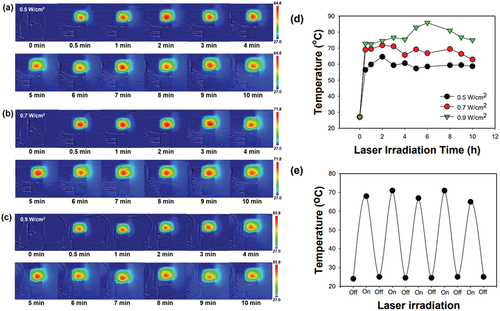
MTX release from the MTX@MIP film upon laser irradiation
The assumption of this study is that MTX release from the MIP film increases with physical changes in the film due to the heat generated by 808 nm laser irradiation. We investigated the difference in the MTX release rate from MIP films in neutral or acidic pH with or without laser irradiation. As shown in , the MTX release rate from the film at pH 5.5 was higher than that at pH 7.4. The reason for the rapid release of MTX from the MIP film is that hydrogen bonds between MTX and MIP film are hydrolysed more quickly at an acidic environment of pH 5.5 than at neutral pH 7.4 [Citation37]. To further confirm that the MTX release rate increased due to heat generation by laser irradiation, the MTX@MIP film was irradiated several times at 0, 1, 2, and 3 h and the MTX release rate therefrom was evaluated (). The drug release rate increased rapidly every time the film was irradiated. The MTX release profile from the MIP film could be regulated by suppressing the formation of hydrogen bonds due to heat induced by the photothermal effect [Citation38]. These results indicate that laser irradiation induces heat generation by melanin, which in turn releases the MTX adsorbed on the MIP film. Field emission-SEM and EDS analyses were performed to confirm this. Pores on the MIP film can be observed in the SEM image in . As shown in , the reason for the change in the structure of the film may be due to that photothermal effect can lead to partial or complete swelling and shrinkage of the film structure [Citation39]. Meanwhile, the EDS spectrum in shows carbon and oxide peaks due to the MIP film, whereas the nitrogen peak indicating the presence of MTX was not observed. These results indicate that the release of MTX adsorbed on the MIP film was induced by the photothermal effect through laser irradiation.
Figure 7. (a) MTX release profiles from the MTX@MIP film upon or without 808 nm laser irradiation one time at pH 5.5 or 7.4 for 12 h (*p < 0.01 and **p < 0.001). (b) MTX release from the MTX@MIP film at pH 5.5 or 7.4 upon 808 nm laser irradiation several times at 0, 1, 2, and 3 h. The red arrows indicate the point in time when the laser was irradiated to the film.
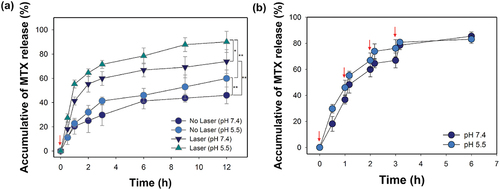
Conclusions
The results of the present study show that MTX specifically adsorbed on the MIP film containing melanin could be released via the photothermal effect generated by NIR laser irradiation. MTX was well adsorbed by specific molecular recognition onto the MIP film. Moreover, repeated NIR laser irradiation increased the release rate of MTX adsorbed on the MIP film. Through these results, we suggest that the target drug could be recognised specifically by the MIP film, and drug release from the MIP film could be controlled by applying NIR laser irradiation.
Disclosure statement
No potential conflict of interest was reported by the author(s).
Additional information
Funding
References
- Joeng GW, Nah JW, Park JK. Application of various hydrophobic moiety-modified chitosan nanoparticle as a drug delivery carrier. Appl Chem Eng. 2017;28:404–8. doi: 10.14478/ace.2017.1041
- Monica R, Vijay S, Sayali K, et al. Design of transdermal patch of ketoprofen by full factorial design for treatment of rheumatoid arthritis. J Drug Delivery Ther. 2019;9(2):197–205. doi: 10.22270/jddt.v9i2.2549
- Huang D, Sun M, Bu Y, et al. Microcapsule-embedded hydrogel patches for ultrasound responsive and enhanced transdermal delivery of diclofenac sodium. J Mater Chem B. 2019;7(14):2330–2337. doi: 10.1039/C8TB02928H
- Hardainiyan S, Kumar K, Nandy BC, et al. Design, formulation and in vitro drug release from transdermal patches containing imipramine hydrochloride as model drug. Int J Pharm Pharm Sci. 2017;9(6):220–225. doi: 10.22159/ijpps.2017v9i6.16851
- Fareghi AR, Moghadam PN, Khalafy J. Synthesis and characterization of a cellulose-based molecularly imprinted polymer in aqueous solution: the study of furosemide slow release. Starch Stärke. 2017;69(11–12):e201700002. doi: 10.1002/star.201700002
- Ma J, Li X, Bao Y. Advanced in cellulose-based superabsorbent hydrogels. RSC Adv. 2015;5:59745–59757. doi: 10.1039/C5RA08522E
- Esuendale D, Gabriel T. Cellulosic on transdermal drug delivery system: a review. J Drug Delivery Ther. 2016;6(5):57–64. doi: 10.22270/jddt.v6i5.1275
- Wells CM, Harris M, Choi L, et al. Stimuli-responsive drug release from smart polymers. J Funct Biomater. 2019;10(3):34. doi: 10.3390/jfb10030034
- Li R, Peng F, Cai J, et al. Redox dual-stimuli responsive drug delivery systems for improving tumor-targeting ability and reducing adverse side effects. Asian J Pharm Sci. 2020;15(3):311–325. doi: 10.1016/j.ajps.2019.06.003
- Karimi M, Zangabad PS, Ghase A, et al. Temperature-responsive smart nanocarriers for delivery of therapeutic agents: applications and recent advances. ACS Appl Mater Interfaces. 2016;8(33):21107–21133. doi: 10.1021/acsami.6b00371
- Zan M, Li J, Huang M, et al. Near-infrared light-triggered drug release nanogels for combined photothermal-chemotherapy of cancer. Biomater Sci. 2015;3(7):1147–1156. doi: 10.1039/C5BM00048C
- Singh R, Torti SV. Carbon nanotubes in hyperthermia therapy. Adv Drug Deliv Rev. 2013;65(15):2045–2060. doi: 10.1016/j.addr.2013.08.001
- Wu J, Li Z, Li Y, et al. Photothermal effects of reduced graphene oxide on pancreatic cancer. Technol Cancer Res Treat. 2018;17:1533034618768637. doi: 10.1177/1533034618768637
- Yao C, Zhang L, Wang J, et al. Gold nanoparticle mediated phototherapy for cancer. J Nanomater. 2016;2016:1–29. doi: 10.1155/2016/5497136
- Slominski RM, Zmijewski MA, Slominski AT. The role of melanin pigment in melanoma. Exp Dermatol. 2015;24(4):258–259. doi: 10.1111/exd.12618
- Eom T, Woo K, Shim BS. Melanin: a naturally existing multifunctional material. Appl Chem Eng. 2016;27(2):115–122. doi: 10.14478/ace.2016.1029
- Riley PA. Melanin. Int J Biochem Cell Biol. 1997;29(11):1235–1239. doi: 10.1016/S1357-2725(97)00013-7
- Kamel AH, Mohammad SG, Awwad NS, et al. Survey on the integration of molecularly imprinted polymers as artificial receptors in potentiometric transducers for pharmaceutical drugs. Int J Electrochem Sci. 2019;14(2):2085–2124. doi: 10.20964/2019.02.23
- Ensafi AA, Nasr-Esfahani P, Rezaei B. Simultaneous detection of folic acid and methotrexate by an optical sensor based on molecularly imprinted polymers on dual-color CdTe quantum dots. Anal Chim Acta. 2017;996:64–73. doi: 10.1016/j.aca.2017.10.011
- Ayari MG, Kadhirvel P, Favetta P, et al. Synthesis of imprinted hydrogel microbeads by inverse Pickering emulsion to controlled release of adenosine 5′‑monophosphate. Mater Sci Eng C. 2019;101:254–263. doi: 10.1016/j.msec.2019.03.102
- Sedghi R, Yassari M, Heidari B. Thermo-responsive molecularly imprinted polymer containing magnetic nanoparticles: synthesis, characterization and adsorption properties for curcumin. Colloids Surf B Biointerfaces. 2018;162:154–162. doi: 10.1016/j.colsurfb.2017.11.053
- Zaidi SA. Molecular imprinting: a useful approach for drug delivery. Mater Sci Energy Technol. 2020;3:72–77. doi: 10.1016/j.mset.2019.10.012
- Bărăian A, Lacob BC, Bodoki AE, et al. In vivo applications of molecularly imprinted polymers for drug delivery: a pharmaceutical perspective. Int J Mol Sci. 2022;23(22):14071. doi: 10.3390/ijms232214071
- Bodoki AE, Lacob BC, Bodoki E. Perspectives of molecularly imprinted polymer-based drug delivery systems in cancer therapy. Polymers. 2019;11(12):2085. doi: 10.3390/polym11122085
- Guðmundsdóttir JS, Fredheim EG, Koumans CI, et al. The chemotherapeutic drug methotrexate selects for antibiotic resistance. EBioMedicine. 2021;74:103742. doi: 10.1016/j.ebiom.2021.103742
- Yildirim-Yalcin M, Tornuk F, Toker OS. Recent advances in the improvement of carboxymethyl cellulose-based edible films. Trends Food Sci Technol. 2022;129:179–193. doi: 10.1016/j.tifs.2022.09.022
- Lei K, Wang X, Li X, et al. The innovative fabrication and applications of carvacrol nanoemulsions, carboxymethyl chitosan microgels and their composite films. Colloids Surf B Biointerfaces. 2019;175:688–696. doi: 10.1016/j.colsurfb.2018.12.054
- Ezati P, Rhim JW, Moradi M, et al. CMC and CNF-based alizarin incorporated reversible pH-responsive color indicator films. Carbohydr Polym. 2020;246:116614. doi: 10.1016/j.carbpol.2020.116614
- Mandal B, Rameshbabu AP, Dhara S, et al. Nanocomposite hydrogel derived from poly(methacrylic acid)/carboxymethyl cellulose/Au NPs: a potential transdermal drugs carrier. Polymer. 2017;120:9–19. doi: 10.1016/j.polymer.2017.05.042
- Jia C, Zhang M, Zhang Y, et al. Preparation of dual-template epitope imprinted polymers for targeted fluorescence imaging and targeted drug delivery to pancreatic cancer BxPC-3 cells. ACS Appl Mater Interfaces. 2019;11(35):32431–32440. doi: 10.1021/acsami.9b11533
- Kusum Devi V, Saisivam S, Maria GR, et al. Design and evaluation of matrix diffusion controlled transdermal patches of verapamil hydrochloride. Drug Dev Ind Pharm. 2003;29(5):495–503. doi: 10.1081/DDC-120018638
- Ruela ALM, Figueiredo EC, Pereira GR. Molecularly imprinted polymers as nicotine transdermal delivery systems. Chem Eng J. 2014;248:1–8. doi: 10.1016/j.cej.2013.12.106
- Kang MS, Cho E, Choi HE, et al. Molecularly imprinted polymers (MIPs): emerging biomaterials for cancer theragnostic applications. Biomater Res. 2023;27(1):45. doi: 10.1186/s40824-023-00388-5
- Tsuchiya M, Hayasaka S, Mizuno K. Affinity of ocular acid-insoluble melanin for drugs in vitro. Invest Ophthalmol Vis Sci. 1987;28(5):822–825. https://iovs.arvojournals.org/article.aspx?articleid=2177800
- Wilczok T, Stȩpień K, Buszman E, et al. Interaction of methotrexate with melanins and melanosomes from B16 melanoma. Biophy Chem. 1990;35(2–3):265–270. doi: 10.1016/0301-4622(90)80014-X
- Kim MA, Yoon SD, Lee JS, et al. Melanin-PEG nanoparticles as a photothermal agent for tumor therapy. Mater Today Communi. 2020;25:101575. doi: 10.1016/j.mtcomm.2020.101575
- Duan X, Yang X, Li C, et al. Highly water-soluble methotrexate-polyethyleneglycol-rhodamine prodrug micelle for high tumor inhibition activity. AAPS PharmScitech. 2019;20(6):6. doi: 10.1208/s12249-019-1462-4
- Li Y, Ding J, Zhu J, et al. Photothermal effect-triggered drug release from hydrogen bonding-enhanced polymeric micelles. Biomarocmol. 2018;19(6):1950–1958. doi: 10.1021/acs.biomac.7b01702
- Xing Y, Zeng B, Yang W. Light responsive hydrogels for controlled drug delivery. Front Bioeng Biotechnol. 2022;1075670. doi: 10.3389/fbioe.2022.1075670