Abstract
A new liposomes/chitosan scaffold/human fibrin gel composite system (LCSHFG), as a depot drug delivery system, was developed to deliver low-molecular weight hydrophilic drugs. An antithrombosis drug, Tirofiban, was used as a model drug. Human fibrin gels encapsulated Tirofiban loaded liposomes were formed within chitosan scaffolds to configure the LCSHFG. The in vitro release behaviors of Tirofiban from LCSHFG were studied by characterizing the constituents of LCSHFG. The results show that the release periods of Tirofiban from LCSHFG with 50 μm pores in the chitosan scaffolds are generally 20% or longer more than those with 200 μm pores. The following results were obtained for the system that comprised 50 μm pores. The release periods of Tirofiban from LCSHFG loaded with stearylamine (SA)-liposomes can sustain 20% longer and significantly less burst release (p < 0.01, n = 3) than with liposomes. The release profiles of Tirofiban from LCSHFG change markedly when 0.5 and 2.5% glutaraldehyde is used to cross-link the system. Additionally, for all liposomes, the release periods of Tirofiban from cross-linked LCSHFG with 2.5% glutaraldehyde are 40% or more longer time (e.g., 19 days) with significantly less burst release (p < 0.01, n = 3) than those of noncrosslinked LCSHFG. Notably, the bioactivity of released Tirofiban from LCSHFG that is crosslinked by 2.5% glutaraldehyde effectively inhibits adenosine diphosphate inducing platelet aggregation. The work also suggests that LCSHFG may have potential as a depot drug delivery system for low-molecular-weight hydrophilic drugs.
Developing a depot delivery system to control the release of biological active peptides or low-molecular weight hydrophilic drugs for medical applications is important. Many researchers have presented various microencapsulation approaches to achieve this objective (Cohen et al. Citation1991; Tabata et al. Citation1993; Blanco et al. Citation1998; Chung et al. 2002). For example, the incorporation of proteins into polyester-based microspheres such as of polylactide has been widely proposed to enable the release of proteins to be controlled by the diffusion characteristics of polymer matrix (Blanco and Alonso Citation1998; Chung et al. 2002). However, the disadvantages of the polymer-based technology may result in a decrease in the bioactivity of proteins during fabrication processes, such as heat of a sonicator or deactivation by an organic solvent (Tabata et al. Citation1993; Chung et al. Citation2002a; Manning et al. Citation1989; Sandor et al. Citation2002).
Liposomes, which are small lipid-based vesicles, can entrap or bind various hydrophilic drugs into interior reservoirs in a lipid membrane or hydrophobic drugs onto the lipid membrane. Polyethylene glycol (PEG)-modified liposomes protect liposomes and their encapsulated drugs from the removal of reticuloendothelial system and thereby increase the half-life of liposomes in the circulatory system (Allen et al. Citation1991; Moribe et al. Citation1999; Takeuchi et al. Citation2001). In clinical applications, drug loaded liposomes are usually injected into blood streams for anticancer therapy (Allen et al. Citation1991; Moribe et al. Citation1999; Takeuchi et al. Citation2001). As well as being injected into the bloodstream, liposomes that are encapsulated in a fibrin clot have been applied as a drug delivery system to sustain the release of peptides or proteins such as growth factors (Moribe et al. Citation1999; Takeuchi et al. Citation2001).
Fibrin clots or gels are blood coagulation products in vivo when thrombin catalyzes the cleavage of fibrinogen to fibrin segments, which polymerize to form fibrin gels, and are then stabilized in the presence of activated factor XIII in the blood. Since the fibrin gels are products of blood coagulation with high biodegradability and biocompatibility, they or their modified derivatives have been studied for use as drug carriers in delivery systems or as scaffolds in tissue engineering (Sakiyama-Elbert and Hubbell Citation2000; Meyenburg et al. Citation2000; Ye et al. Citation2000; Park et al. Citation2005).
Chitosan, an amino polysaccharide is derived from chitin by deacetylation, also is a biodegradable and biocompatible biomaterial that contains various reactive groups that can be modified to improve cell growth for use as a scaffold in tissue engineering (Madihally and Matthew Citation1999; Chung et al. Citation2003) or can be crosslinked by various agents to be used as a drug carrier (Shu et al. Citation2001). For instance, chitosan scaffolds with different pore sizes can be fabricated by a lyophilization method by controlling the freezing temperature using liquid nitrogen or liquid carbon dioxide and then grafting active peptides for tissue engineering applications (Chung et al. 2002; Madihally and Matthew Citation1999; Chung et al. Citation2003).
Tirofiban, a hydrophilic antithrombosis drug with a low molecular weight (such as 492 Da), is used for patients with unstable angina or acute myocardial infarction. Tirofiban blocks glycoprotein IIb/IIIa receptors on the platelet membrane to bind with fibrinogen and inhibits the aggregation of platelets (Restore et al. Citation1997; Prism-Plus et al. Citation1998). In therapeutic applications, an intravenous perfusion of a low concentration of Tirofiban in the bloodstream (10–20 μg/ml) for 36 hr or longer is usually used in such patients (Restore et al. Citation1997; Prism-Plus et al. Citation1998). The sustained release of Tirofiban by a delivery system may clinically benefit the patients, and this drug was thus selected as a model drug in our research.
In this work, various biodegradable, biocompatible, and drug-delivery properties of biomaterials, liposomes, chitosan scaffolds, and human fibrin gels (LCSHFG) were exploited to develop a new composite delivery system to deliver low-molecular weight hydrophilic drugs, based on Tirofiban as a model drug, for potentially clinical applications. LCSHFG is configured using human platelet poor plasma to produce human fibrin gels that encapsulate Tirofiban-loaded liposomes in a chitosan scaffold and interact with the scaffold electrostatically. The in vitro release behaviors of Tirofiban from the system were studied by varying such parameters as the surface charge of the liposomes, the pore sizes in the chitosan scaffolds, and the degree of crosslinking of the fibrin gels. Moreover, the preservation of the bioactivity of the released Tirofiban from LCSHFG was tested by confirming the ability of the drug to inhibit platelet aggregation Adenosine diphosphate induced by (ADP), a biomolecule that mediates chemical reactions between fibrinogen and glycoprotein IIb/IIIa receptors of platelet membrane and causes platelet aggregation.
MATERIALS AND METHODS
Preparation of Tirofiban-Loaded Liposomes
Egg phosphatidylcholine (PC) with a purity of 90%, stearylamine (SA), dicetyl phosphate (DP), cholesterol, and vitamin E were purchased from Sigma Chemical Co., (St. Louis, MO, USA). The procedures for preparing liposomes have been presented elsewhere (Castile and Taylor Citation1999; Chung et al. Citation2006). PC (e.g., 0.32 g), cholesterol, and vitamin E were all dissolved in 20 ml of 95% alcohol in a molar ratio of 11: 4: 0.1. The mixture was dried in a rotary evaporator to produce a lipid film, which was further purged using nitrogen gas. The lipid film was then hydrated with 100 ml sodium citrate buffer at 42°C and then sonicated using an ultrasonicator at 40W (GT 50T, Cole-Palmer Co., IL, USA) to yield liposome suspensions.
After centrifugation at 1500 g to remove unwanted lipid aggregates, the liposome suspensions were filtered through a 0.45 μm polycarbonate membrane to form homogeneous liposomes for applications. In preparing SA- or DP-liposomes, so-called positively or negatively charged liposomes, the molar ratios of PC, cholesterol, vitamin E, and the aforementioned components such as SA or DP were changed to 11: 4: 0.1:1, and the same preparation procedures as used for liposomes (Chung et al. Citation2006) were followed.
In preparing Tirofiban-loaded liposomes, 10 ml of 0.25 mg/ml of Tirofiban was added to liposome suspensions, which were extruded three times through polycarbonate membrane filters with 0.10 μm pores at 42°C before being put through 3 freeze-and-thaw cycles (Meyenburg et al. Citation2000), (Castile and Taylor Citation1999). After the above process, the suspensions were dialyzed through a flow-type membrane dialyzer with a cut-off molecular weight of 10 kDa (Spectrum Laboratory, USA) to remove unencapsulated Tirofiban and to concentrate liposome suspensions. The concentrated Tirofiban-loaded liposome suspensions were then ready for further testing.
Tirofiban Loaded Liposomes and Encapsulation Efficiency
The sizes and surface charges of Tirofiban-loaded liposomes in saline (0.9% of Nacl) solutions were determined using a particle size/ zeta potential analyzer (a Zeta 90 plus digital laser particle-sizer, Brookhaven Instrument Corp., USA). The dialysate obtained from the aforementioned flow-type membrane dialyzer was first collected to measure the concentration of Tirofiban using an HPLC method (Bergquist et al. Citation2001) to determine the encapsulation efficiency of Tirofiban-loaded liposomes. First, 20 μl of samples were injected into an HPLC (Jasco PU-1580, Kobe, Japan), equipped with a C18 column (Betasil, 4.6 mm × 25 cm ID, CA, USA) at 40°C. Second,ssss 10% acetonitrile dissolved in 90% 10 mM of potassium phosphate at a pH of 2.3 was the mobile phase with 0.8 ml/min flow rate. The quantity of Tirofiban then was revealed by the absorption intensity at a wavelength of 227 nm, which was analyzed using standard software built into the HPLC (Bergquist et al. Citation2001). The encapsulation efficiency of Tirofiban in liposomes was determined by subtracting the quantities of Tirofiban in the dialysate from the initially loaded Tirofiban and then dividing the result by the initially loading of Tirofiban.
Porous Chitosan Scaffold
Chitosan was purchased from Primex Ingredients ASA (Norway; batch #: TM 732, Mw. of 200–700 kDa) with 96% deacetylation and with a characteristic viscosity of 1% chitosan in 1% acetic acid solution of 57 mPa s. First 1.5 g chitosan was dissolved in 100 ml of 1% acetic acid solution and then filtered through a 0.45 μm polycarbonate membrane to yield the chitosan solution. Second this solution was poured into glass disks and then solidified into different frozen states using liquid nitrogen or carbon dioxide for 24–48 hr before it was lyophilized in a freeze-dryer to fabricate porous chitosan scaffolds with different pore sizes (Madihally and Matthew Citation1999; Chung et al. Citation2003, Citation2006). Third, to prepare scaffolds for SEM, they were dehydrated in a series of ethanol solutions and then sputter coated with gold/palladium in vacuum. The morphology of chitosan scaffolds was observed by a scanning electron microscopy (Hitachi S4700,Tokyo, Japan) and the pore sizes of the scaffolds of the SEM monographs were analyzed and determined using igital image software (Simple PCI, Compix Inc., PA, USA).
According to analyses, the mean pore sizes of chitosan scaffolds were ∼50 and 200 μm in this study. The porosity of the chitosan scaffolds was determined using the following equation.
where ρa is the apparent density of the porous scaffold and ρt is the density of nonporous chitosan materials.
In this work, the porosity of the chitosan scaffolds was ∼85%. The fabricated chitosan scaffolds were further fixed by 3.8% sodium citrate solution and dried at room temperature for the experiments. A chitosan scaffold with an area of 1 × 1 cm2 and a thickness of 3 mm was cut to fabricate LCSHFG.
LCSHFG Crosslinked by Different Concentrations of Glutaraldehyde
In preparing the platelet poor plasma (PPP) of normal subjects, 5 ml of fresh blood suspension mixed with 3.8% of sodium citrate (n = 3) was centrifuged at 1500 g for 10 min to separate blood cells and plasma, as in the authors' earlier investigation (Chung et al. 2002). After the upper level plasma suspension was separated, the platelets were counted using a platelet counter (Sysmex PDA-500, Toa Medical Electronic, Kobe, Japan). Fewer than 1.0 × 104 platelets per μl was defined as PPP in this work (Chung et al. 2002). Bovine thrombin (Sigma Chemical) was dissolved in sodium citrate buffer to prepare thrombin solution at a concentration of 50 unit / ml. First, 1.0 ml of PPP was gently mixed with 1.5 ml of Tirofiban-loaded liposomes. Second, 0.65 ml of mixed solution was injected into a chitosan scaffold and 0.1 ml of thrombin solution was then injected into the scaffold to initiate fibrin gels; the solution was allowed to stand for 30 min for stabilization. The total weight of LCSHFG without a chitosan scaffold was 0.745 g.
To prepare LCSHFG for SEM, the procedures were the same as those for chitosan scaffolds and the sample was then observed with the aforementioned microscopy. To prepare LCSHFG with different degrees of crosslinking of the fibrin gels, LCSHFG was dipped into 0.5 or 2.5% glutaraldehyde solution for 5 min and then washed with distilled water several times to remove residual glutaraldehyde.
In Vitro Release of Tirofiban of LCSHFG
The crosslinked and noncrosslinked LCSHFG containing Tirofiban-loaded liposomes were placed into an osmosis membrane bag with a cut-off molecular weight of 300 k Da (Spectrum Medical Industries, USA) to study the release behaviors of Tirofiban. The bag was suspended in a vial of 20 ml saline solution supplemented with 0.1 wt % of sodium azide, an antibacterial reagent, as a dissolution medium (Chung et al. 2002). The vial was shaken at 60 rpm at 25°C to obtain release profiles of Tirofiban (Chung et al. 2002). Next, 0.5 ml of the dissolution medium was periodically drawn out and the concentration of Tirofiban in the medium was determined by HPLC. After it had been drawn, the same volume of fresh medium was added to the vial. The cumulative concentrations of released Tirofiban were measured by HPLC. The release profiles of Tirofiban with different degrees of crosslinking of LCSHFG were examined under the same conditions used for the non-crosslinked LCSHFG.
Bioactivity of Released Tirofiban
The Tirofiban released on the 11th day of 2.5% glutaldehyde-crosslinked LCSHFG of DP-liposomes was collected to examine whether LCSHFG can preserve the bioactivity of Tirofiban, antiaggregation of platelets. To prepare the platelet samples, 1.0 ml of fresh plasma that contained ∼3.0 × 105 per μl of platelets was obtained from the blood of normal subjects. For the control group, the sample was preincubated with 1 ml of saline solution for 30 min. For the test group, the sample was preincubated with the same volume of released Tirofiban solution rather than saline solution. Saline solution that contained 6.25 μM (in final concentration) of ADP (Sigma Chemical Co) and 0.024 μM (in final concentration) CaCl2 solution were added to the platelet samples with gently stirring at 37°C to induce platelet aggregation of both groups (Maes et al. Citation1996). The morphology of ADP-inducing aggregated platelets of the samples were observed using a microscope to investigate the size distribution (Nikon TE-100, Tokyo, Japan), and counted using a platelet particle counter (Sysmex PDA-500, Toa Medical Electronics). The details of the counting procedures are presented elsewhere (Chung et al. 2002).
All the calculations were made using the Sigmastat statistical software (Jandel Science Corp., San Rafael, CA, USA). Statistical significance in the Students t-test corresponded to a confidence level of at least 95%. Data are presented as mean ± s.d., having been measured at least from triplicate measurements.
RESULTS
Characteristics of Liposomes that Encapsulated Tirofiban
The Tirofiban encapsulated in SA-, DP- and liposomes had first to be characterized before LCSHFG was fabricated. lists the zeta potentials, particle sizes, and encapsulation efficiencies of various Tirofiban-loaded liposomes. The zeta potentials of those liposomes indicate that the surface charges on the liposomes are consistent with their lipid formula. That is, the DP-liposomes have negative charges and the SA-liposomes have positive charges. The results also show that DP-liposomes have the highest encapsulation efficiency of Tirofiban (52.0 ± 1.4%) whereas liposomes have the lowest (41.7 ± 1.2%). The particles of SA-liposomes are the largest (309.9 ± 8.1 nm), while those of liposomes are the smallest (264.1 ± 6.6 nm)
TABLE 1 Particle sizes, encapsulation efficiency of Tirofiban and zeta potential of different surface charges of liposomes (n = 3)
Different Pore Sizes of Chitosan Scaffolds
The SEM micrograph presents the pore structures (or compartments) of chitosan scaffolds with pore sizes of ∼50 μm (). The pores in the scaffolds of sizes of ∼200 μm have the same structures as the 50 μm pores, but they have large compartments (data not shown).
Structure of Human Fibrin Gel
In this work, fresh PPP plasma rather than fibrinogen was employed to produce the gels. SEM micrographs of those areas were taken to observe the internal structure of fibrin gels in the chitosan scaffolds of LCSHFG. The micrographs show that several layers of fibrin gels with polydispersed pores (with diameters of 2–8 μm) adhere to the inner surface of the chitosan scaffolds ( and ). Additionally, the structures of fibrin gels are much denser than the network that is formed from bovine or human fibrinogen(Sakiyama-Elbert and Hubbell Citation2000; Meyenburg et al. Citation2000; Chung et al. Citation2006).
FIG. 2 (a) SEM micrograph of dense human fibrin gels that contain poly dispersed pores and adhere to chitosan scaffolds in an LCSHFG system (× 500). The square area of the gels is further observed as presented in . 76 × 57 mm (600 × 600 DPI). (b) SEM micrograph of fibrin structures in LCSHFG system comprised of several layers of the fibrin network with polydispersed pores (× 2000). 76 × 57 mm (600 × 600 DPI).
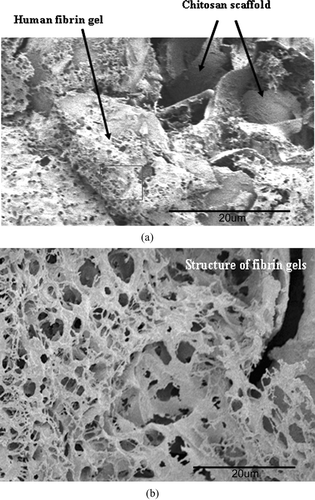
Effects of Pore Size in Chitosan Scaffolds
, , demonstrate that the size of pores such as 50 and 200 μm of chitosan scaffolds affect the cumulative release profiles of Tirofiban from LCSHFG, but the charges of liposomes (, , ) also affect the profiles. For instance, the differences between the Tirofiban release behaviors associated with the two pore sizes in the scaffolds are the greatest for SA-liposomes and the least for liposomes (neutral charge). Only an LCSHFG system that had been loaded with SA-liposomes comprised 50 μm pores exhibited a significant reduction (p < 0.01, n = 3) in the burst release of Tirofiban on the first day, and significant differences (p < 0.01, n = 3) in the cumulative release profiles of Tirofiban, by comparison with the results for the 200 μm pores ().
FIG. 3 (a) No effect of sizes of pores in chitosan scaffolds on cumulative release profiles of Tirofiban of liposome-loaded LCSHGF system. 153 × 116 mm (600 × 600 DPI). (b) Small effect of size of pores in chitosan scaffolds during the 3rd and 11th day on the cumulative release profiles of Tirofiban of DP-liposome-loaded LCSHGF system. 155 × 120 mm (600 × 600 DPI). (c) Strong effect of size of pores in chitosan scaffolds on cumulative release profiles of Tirofiban of SA-liposomes-loaded LCSHGF system. Scaffolds with 50 μm pores exhibit significantly less burst release of Tirofiban (*p < 0.01, n = 3) than those with 200 μm pores. 153 × 123 mm (600 × 600 DPI).
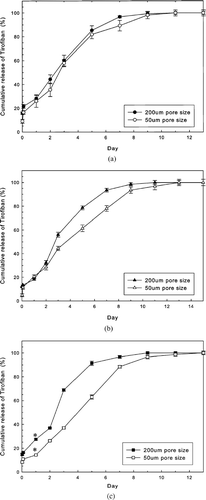
Notably, LCSHFG that comprises scaffolds with 50 μm pores can effectively sustain the release of Tirofiban for ∼20% (from 9 to 11 days for liposomes and SA-liposomes) or 36% (from 9 to 13 days for DP-liposomes) longer than can be achieved with 200 μm pores (, , ). Accordingly, LCSHFG with 50 μm pores in the chitosan scaffolds were examined.
Effect of Surface Charges of Liposomes
presents the effects of the charge on the liposomes loaded into LCSHFG on the release behaviors of Tirofiban. The cumulative profiles of Tirofiban from LCSHFG that contained charged liposomes differed somewhat. The release profiles of Tirofiban associated with LCSHFG that comprised charged liposomes differ from those of liposomes during the first 7 days as presented in . The release period of Tirofiban from LCSHFG that contains DP-liposomes is ∼20% longer sustained than that of liposomes. The release of Tirofiban from LCSHFG that contains SA-liposomes has a significantly lower burst release (p < 0.01, n = 3) on the first day than liposomes.
FIG. 4 Effect of charges on liposomes on cumulative release profiles of Tirofiban of LCSHFG. The release profiles of Tirofiban from LCSHFG with charged liposomes differ from that of liposomes during the first 6 days. The burst release of Tirofiban of LCSHFG that comprised SA-liposomes was significantly less (*p < 0.01, n = 3) than that of liposomes. 153 × 118 mm (600 × 600 DPI).
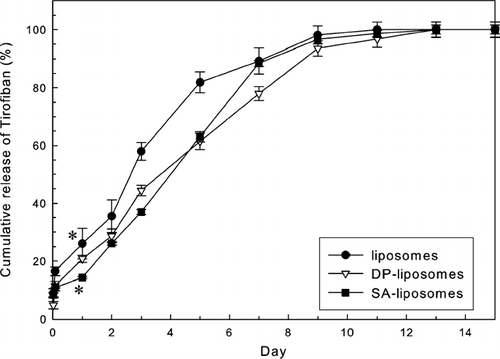
Effects of Degree of Crosslinking in Fibrin Gels
Both 0.5 and 2.5% glutaraldehyde solutions were used to crosslink LCSHFG with variously charged liposomes to examine the effects of LCSHFG crosslinked by glutaraldehyde on the release behaviors of Tirofiban (, , ). Using 0.5 and 2.5% glutaraldehyde to crosslink LCSHFG generally increased release time by 20% and 40%, respectively (19 days), over that associated with noncrosslinked LCSHFG (13 days) for all tested liposomes. Additionally, for all tested liposomes, the burst releases of theTirofiban in the crosslinked LCSHFG systems were significantly lower than those in the noncrosslinked systems. For example, the burst releases of Tirofiban of DP-liposome-loaded LCSHFG were significantly (p < 0.01 or better, n = 3, respectively) lower for 0.5 and 2.5% glutaraldehyde-crosslinked LCSHFG (12.5 ± 1.2% and 2.8 ± 0.8%, respectively) than for noncrosslinked (20.8 ± 1.5%) LCSHFG ().
FIG. 5 (a) Effect of degree of crosslinking in fibrin gels on release profiles of Tirofiban of liposome-loaded LCSHFG system. The burst release of Tirofiban in LCSHFG that was crosslinked by 2.5% glutaraldehyde are significantly reduced compared with noncrosslinked LCSHFG (*p < 0.01, n = 3), and the release period is increased from 11 days (e.g., noncrosslinked) to 19 days. 153 × 123 mm (600 × 600 DPI). (b) Effect of degree of crosslinking in fibrin gels on release profiles of Tirofiban of DP-liposome-loaded LCSHFG system. The burst release of Tirofiban of LCSHFG that is crosslinked by 2.5% glutaraldehyde is significantly lower than that of noncrosslinked LCSHFG (*p < 0.01, n = 3), and the release period is increased from 13 days (e.g., noncrosslinked) to 17 days. 153 × 123 mm (600 × 600 DPI). (c) Effect of degree of crosslinking of fibrin gels on release profiles of Tirofiban of SA-liposome-loaded LCSHFG system. The cumulative releases of Tirofiban of LCSHFG that are crosslinked by 2.5% glutaraldehyde are significantly lower than those of noncrosslinked LCSHFG (*p < 0.01, n = 3), and the release period is increased from 13 days (e.g., noncrosslinked) to 17 days. 153 × 123 mm (600 × 600 DPI). (d) Cumulative releases of Tirofiban from LCSHFG crosslinked with 0.5% glutaraldehyde. The burst releases of Tirofiban between liposome-loaded and SAliposome- loaded LCSHFG systems differ significantly (*p < 0.01, n = 3). 153 × 118 mm (600 × 600 DPI). (e) Cumulative releases of Tirofiban from LCSHFG crosslinked with 2.5% glutaraldehyde are approximately equal and thus independent of the charges on the liposomes that are loaded into the system. 153 × 116 mm (600 × 600 DPI).
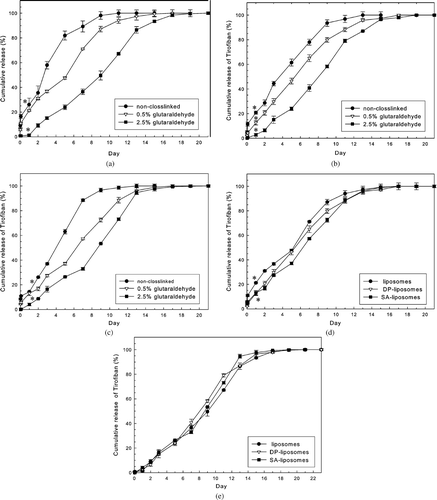
Moreover, for all tested liposomes, the decreased releases of Tirofiban from crosslinked LCSHFG correspond to the concentrations of glutaraldehyde that are used to crosslink fibrin gels (, , ). For instance, the cumulative releases of Tirofiban of the LCSHFG that is loaded with DP-liposomes on the ninth day were 93.5 ± 1.5%, 79.9 ± 2.0%, and 58.3 ± 0.8% for noncrosslinked, 0.5 and 2.5% glutaraldehyde-crosslinked fibrin gels (). Interestingly, the release profiles of Tirofiban from 0.5% glutaraldehyde-crosslinked LCSHFG systems vary slightly with the charge on the liposomes () while those of the 2.5% glutaraldehyde-crosslinked systems are independent thereof ().
Bioactivity of Tirofiban
The released Tirofiban from 2.5% glutaldehyde-crosslinked LCSHFG that contained DP-liposomes was collected to examine whether the bioactivity of Tirofiban, the antiaggregation of platelets, was preserved. According to HPLC measurements, the cumulative concentration of released Tirofiban on the 11th day was 11.5 μg/ml. This was the concentration collected for the test because it is in the range of clinical doses. Observing the ADP-added platelet samples with a fluorescent microscope revealed numerous platelet aggregates. In contrast, only a very few platelet aggregates were observed in the samples that had been preincubated with the collected released Tirofiban (data not shown).
The mean particle size of platelets of ADP-added platelet samples (3.25 ± 0.28 μm) significantly (p < 0.025, n = 4) exceeded that of samples without added ADP (e.g., 2.39 ± 0.58 μm) and of samples preincubated with released Tirofiban (e.g., 2.34 ± 0.53 μm). Notably, no difference existed between the mean particle sizes of platelets in samples preincubated with released Tirofiban and those in groups to which no ADP had been added. This suggests that the released Tirofiban significantly inhibited platelet aggregation. Hence, using 2.5% glutaraldehyde to crosslink LCSHFG does not affect the bioactivity of released Tirofiban.
DISCUSSION
In this work, an LCSHFG system was developed as a new depot drug delivery system for hydrophilic drugs with low molecular weight, such as Tirofiban. Furthermore, the drug was loaded into liposomes and then encapsulated into scaffolds by fibrin gels to produce the system. No toxic organic solvent or ultrasonication was employed to ensure the stability of the loaded drugs and to avoid the disadvantages of delivering drugs by polyester-based micro- or nanoparticles fabricated by emulsion-solvent evaporation or diffusion methods (Cohen et al. Citation1991; Tabata et al. Citation1993; Blanco and Alonso Citation1998; Chung et al. 2002; Sandor et al. Citation2002).
The main purpose of liposome-encapsulated Tirofiban in the system was to provide reservoirs for Tirofiban and prevent the drug from biochemically reacting with human fibrin gel. Moreover, interactions between charged liposomes and fibrin gels may occur, as reported in the authors' earlier study (Chung et al. Citation2006). According to our earlier study, liposomes that encapsulated a water-soluble agent, such as quinacrine, could only sustain release for 24–36 hr (Chung et al. Citation2006), which depended weakly on the sizes, encapsulation efficiencies, and surface charges of liposomes. Liposome-encapsulated hydrophilic low-molecular-weight drugs may not be suitable for a long-lasting drug delivery for a week or longer.
The encapsulation efficiencies of Tirofiban did not correspond to the sizes of the liposomes (). This result differs from that in another work (Chapman et al. Citation1990) perhaps because the two works used different drugs or lipid compositions. We reported that the molecular weight of hydrophilic drugs importantly affected sustained release when drugs were delivered by liposomes (Yang Citation2003). In this work, Tirofiban is a low-molecular weight hydrophilic drug encapsulated in liposomes for delivery. Accordingly, such other parameters as the sizes of liposomes and the encapsulation efficiencies of Tirofiban in liposomes would only slightly influence the release of Tirofiban from liposomes. The results presented in and support our statements of release behaviors of Tirofiban from LCSHFG.That is, neutral liposomes have the smallest size and lowest encapsulation efficiencies of Tirofiban () whereas they reveal the faster release of Tirofiban from LCSHFG than others do.
Although the contents of lipids, such as Distearoyl phosphatidylcholine (DSPC) and cholesterol, may influence the stability of liposomes and their drug release behaviors when they are injected into a circulatory system, they did not do so herein because the liposomes are entrapped in the fibrin gels (Meyenburg et al. Citation2000) of LCSHFG. The possible interactions between the surface charges of liposomes and fibrin gels in the system affect the release behaviors of Tirofiban from the system.
The chitosan scaffolds contain numerous compartments () that provide reservoirs/void spaces in which Tirofiban-loaded liposomes can be encapsulated in fibrin gels. Possible interactions between the chitosan surfaces and fibrin gels are expected. Fibrinogen comprises two pairs of three polypeptide chains, with a zeta potential of the fibrinogen of −38.5 mV in a solution with pH 7.3 (Yang Citation2003). The change in the fibrin gels/network is negative because the gels are the final products of the enzymatic reactions of thrombin and fibrinogen in blood coagulation (Ichinose et al. Citation1983). More precisely, thrombin cleaves the terminal NH2 of the 3 polypeptide chains of fibrinogen into 6 small polypeptide chains, which contain the carboxyl-terminal segments, and are polymerized to produce fibrin gels in calcium ions (Ichinose et al. Citation1983). Positively charged chitosan scaffolds that contain numerous amine groups would interact with carboxyl-terminated segments of fibrin gels electrostatically. In fact, a force was required to tear the fibrin gels off chitosan surfaces, although it was not quantified (Yang Citation2003).
Because fresh human PPP was used to construct fibrin gels of the LCSHFG system, incorporating such other plasma proteins as globulin and albumin into the gels contributed to the dense structure of the gel ( and ), which was denser than the fibrin network fabricated using fibrinogen only (Meyenburg et al. Citation2000; Ye et al. Citation2000; Chung et al. Citation2006). Although the SEM micrographs did not reveal encapsulated liposomes within the fibrin network, liposomes may have remained in an intact state (Meyenburg et al. Citation2000).
The release periods of Tirofiban from LCSHFG with 50 μm pores in the chitosan scaffolds were longer than those with 200 μm pores since the former scaffold may have stronger electrostatic forces between the gels and the chitosan scaffolds (, , ). Interestingly, the differences between the release profiles of Tirofiban from scaffolds with 50 and 200 μm pores were most evident in LCSHFG with charged liposomes (, , ). Moreover, the greatest difference between the release profiles of Tirofiban was evident in the system of positively charged liposomes (e.g., SA-liposomes), perhaps because of the electrostatic interactions between liposomes and the negatively charged fibrin gels (). The electrostatic interactions between liposomes and fibrin gels of LCSHFG also partially explain the difference release patterns of Tirofiban of the systems with different zeta potentials of liposomes ().
The use of coagulation factor XIII (Meyenburg et al. Citation2000; Ichinose et al. Citation1983; Schense and Hubbel Citation1999) or glutaraldehyde to crosslink the fibrin network has been proposed to increase the stability of the bovine fibrin network for drug delivery systems such as associated with the resistance of hydrolysis of the network (Yang Citation2003; Chapman et al. Citation1990; Ichinose et al. Citation1983; Schense and Hubbell Citation1999; Ho et al. Citation1994). Accordingly, the stability of fibrin gels for drug delivery systems affects release characteristics of drugs 24–28. Although coagulation factor XIII was present in the plasma fibrin gels herein, the Tirofiban release behaviors of LCSHFG (, , ) were still related to the stability of the gels, as stated in our earlier report on a similar system (Yang Citation2003).
In this work, the macroscopic morphology of the system remained intact for all LCSHFG until end of studying period, although few lipids and fibrin products released from LCSHFG were present and their concentrations semiquantitatively measured during the release periods of Tirofiban for noncrosslinked systems (Yang Citation2003). Generally, the results on the stability of noncrosslinked bovine fibrin systems, evaluated by measuring fibrin products and chitosan polymers released from the system presented elsewhere (Chung et al. Citation2006), are similar to the results in this investigation. Notably, the quantities of released lipids and fibrin products from crosslinked LCSHFG to the dissolution medium were very low and in the middle stage (8–12 days) of the release period of Tirofiban (Yang Citation2003). Hence, the crosslinked LCSHFG systems are more stable than the noncrosslinked ones.
Similar resulted have been obtained for crosslinked fibrin delivery systems (Chung et al. Citation2006). In this respect, using 2.5% glutaraldehyde to crosslink LCSHGF produced highly crosslinked fibrin gels, and crosslinks between fibrin gels and the chitosan scaffolds increased the stability of the system and the period of sustained release of Tirofiban above that of noncrosslinked LCSHGF (, , ). Additionally, since using 2.5% glutaraldehyde to crosslink the system markedly enhanced the stability of the system, the effects of the charge on the liposomes on the release of Tirofiban in crosslinked LCSHFG was negligible (). Accordingly to aforementioned analysis, many factors rather than molecular diffusion affect the release of Tirofiban from LCSHFG system, using a simple diffusion model (Ritger et al. 1987) (or plotting release of drug versus t1/2) cannot clearly explain the release patterns of our systems (data not shown).
The bioactivity tests demonstrated that using 2.5% glutaraldehyde crosslinking LCSHFG does not affect the bioactivity of Tirofiban in inhibiting platelet aggregation. This effect, perhaps, results, because of the encapsulation of Tirofiban in liposomes since the lipid membranes prevent the biochemical interactions to produce fibrin gels and crosslinking reactions between the Tirofiban and fibrin gels that involve thrombin and glutaraldehyde, respectively.
CONCLUSION
A new developed LCSHFG system, as a depot drug delivery system, was investigated. Differently charged liposomes served as reservoirs that encapsulated a low-molecular-weight hydrophilic model drug, Tirofiban, and may prevent loss of bioactivity of the drug (when fibrin gels are crosslinked with 2.5% glutaraldehyde). The 50 μm pores in chitosan scaffolds provided large voids for the formation of human fibrin gels and also provided electrostatic interactions between the chitosan matrix and the gels of LCSHFG. Using 0.5% and 2.5% glutaraldehyde to crosslink LCSHFG can sustain the release of Tirofiban for 19 days without loss of bioactivity of the drug. The results of our work suggest that LCSHFG as a depot drug delivery system may have the potential for delivering low-molecular-weight hydrophilic drugs. In-vivo animal tests for delivering Tirofiban with LCSHFG systems are planned in the near future.
ACKNOWLEDGMENT
The authors thank the National Science Council of the Republic of China, Taiwan, for financially supporting this research under contract no. NSC-92-2329-B-224-01 and NSC-93-2213-E-224-009.
REFERENCES
- Allen M., Hansen C., Martin F., Redemann C., Young A. Y. Liposome containing synthetic lipid derivatives of poly (ethylene glycol) show prolonged circulation half-lives in vivo. Biochem. Biophs. Acta 1991; 1066: 29–36
- Bergquist P. A., Manas D., Hunke W. A., Reed R. A. Stability and compatibility of Tirofiban hydrochloride during simulated Y-site administration with other drugs. Am. J. Health. Syst. Pharm. 2001; 58: 1218–1223
- Blanco D., Alonso M. J. Protein encapsulation and release from poly (lactide-co-glycolide) microspheres: effect of the protein and polymer properties and of the co-encapsulation of surfactants. Eur. J. Pharm. Biopharm. 1998; 45: 285–294
- Castile J. D., Taylor K. M. G. Factors affecting the size distribution of liposomes produced by freeze-thaw extrusion. Int. J. Pharm 1999; 188: 87–95
- Chapman C. J., Erdahl W. L., Taylor R. W., Pfeiffer D. R. Factor affecting solute entrapment in phospholipid vesicles prepared by the freeze-thaw extrusion method: a possible general method for improving the efficiency of entrapment. Chem. Phys. Lipids 1990; 55: 73–83
- Chung T. W., Huang Y. Y., Tsai Y. L., Liu Y. Z. Effects of solvent evaporation rate on the properties of protein-loaded PLLA and PDLLA microspheres fabricated by emulsion-solvent evaporation process. J. Microencap. 2002a; 19: 463–471
- Chung T. W., Tyan Y. C., Hsieh J. H., Wang S. S., Chu S. H. Shear stress-induced aggregation of oxidized platelets. Thromb Res. 2002b; 105: 325–329
- Chung T. W., Lu Y. F., Wang S. S., Lin Y. S., Chu S. H. Growth of human endothelial cells on the photochemically grafted Gly-Arg-Gly-Asp (GRGD) chitosans. Biomaterials. 2003; 23: 4803–4809
- Chung T. W., Yang M. G., Tsai W. J. A fibrin encapsulated liposomes- in-chitosan matrix (FLCM) for delivering water-soluble drugs, Influences of the surface properties of liposomes and crosslinked fibrin network. Int. J. Pharm. 2006; 311: 122–129
- Cohen S., Yoshioka T., Lucarrelli M., Hwang L. H., Langer R. Controlled delivery systems for proteins based on poly (lactic/glycolic acid) microspheres. Pharm. Res. 1991; 8: 713–720
- Ho H. O., Hsiao C. C., Chen C. Y., Sokoloski T. D., Sheu M. T. Fibrin-based drug delivery systems, II. The preparation and characterization of micro-beads. Drug. Dev. Ind. Pharm. 1994; 20: 535–546
- Ichinose A., Tamaki T., Aoki N. Factor XIII-mediated cross-linking of NH2-terminal peptide of α2-plasmin inhibitor to fibrin. FEBS Lett 1983; 152: 369–371
- Madihally S. V., Matthew H. W. T. Porous chitosan scaffolds for tissue engineering. Biomaterials 1999; 20: 1133–1142
- Maes M., Planken M. V., Gastel A. V., Desnyder R. Blood coagulation and platelet aggregation in major depression. J. Affect. Disord 1996; 40: 35–40
- Manning M. C., Patel K., Borchardt R. T. Stability of protein pharmaceuticals. Pharm. Res. 1989; 6: 903–918
- Meyenburg S., Lilie H., Panzner S., Rudolph R. Fibrin encapsulated liposomes as protein delivery system studies on the in vitro release behavior. J. Contr. Rel. 2000; 69: 159–168
- Moribe K., Maruyama K., Iwatsuru M. Molecular localization and state of amphotericin B in PEG liposomes. Int. J. Pharm. 1999; 193: 97–106
- Park S. H., Park S. R., Chung S. I., Pai K. S., Min B. H. Tissue-engineering cartilage using fibrin / hyaluronan composite gel and its in vivo implantation. Artif. Organs. 2005; 29: 838–845
- Prism-Plus Investigators. Inhibition of the platelet glycoprotein IIb/IIIa receptor: inhibition with Tirofiban in unstable angina and non-Q-wave myocardial infarction. N. Engl. J. Med. 1998; 38: 1488–1497
- Restore Investigators. Effects of platelet glycoprotein IIb/ IIIa blockade with Tirofiban on adverse cardiac events in patients with unstable angina or acute myocardial infarction undergoing coronary angioplasty. Circulation 1997; 96: 1445–1453
- Sakiyama-Elbert S., Hubbell J. Development of fibrin derivatives for controlled release of heparin-binding growth factors. J. Contr. Rel. 2000; 65: 389–402
- Sandor M., Riechel A. I., Kaplan A., Mathiowitz E. Effect of Lecithin and MgCO3 as additives on the enzymatic activity of carbonic anhydrase encapsulated in poly (lactide-co-glycolide) (PLGA) microspheres. Biochim. Biophys. Acta. 2002; 1570: 63–74
- Schense J. C., Hubbell J. A. Cross-linking exogenous bifunctional peptides into fibrin gels Factor XIIIa. Bioconj. Chem. 1999; 10: 75–81
- Shu X. Z., Zhu K. J., Song W. Novel pH-sensitive citrate cross-linked chitosan film for drug release. Int. J. Pharm. 2001; 212: 19–28
- Tabata Y., Gutta S., Langer S. Controlled delivery systems for proteins using polyanhydride microspheres. Pharm. Res. 1993; 10: 487–496
- Takeuchi H., Kojima H., Yamamoto H., Kawashima Y. Evaluation of circulation profiles of liposomes coated with water-soluble polymers having different molecular weights in rats. J. Contr. Rel. 2001; 75: 83–91
- Yang M. G. Porous Chitosan Membrane for Controlled Drug Release from a Liposome/Fibrin System. Master Thesis, Dept. of Biomedical Engineering, Chung-Yuan Christian University, Chung Li. Taiwan, ROC 2003
- Ye Q., Zund G., Benedikt P., Jockennoevel S., Hoerstrup S. P., Sakyama S., Hubbell J. A., Turina M. Fibrin gel as a three dimensional matrix in cardiovascular tissue engineering. Eur. J. Cardiothor. Surg. 2000; 17: 587–591