Abstract
New thermosensitive hydrogels of poly(N-isopropylacrylamide) (PNIPAAm) with chitosan (CPN) were prepared and evaluated for use in the delivery of the platinum drugs, cisplatin and carboplatin. The effects of polymers containing different ratios of chitosan on the physicochemical and drug release characteristics were examined. The sol-gel transition temperature of the hydrogels was determined by differential scanning calorimetry (DSC) and viscometry. Discrepancies in the transition temperature among the various polymer systems were more pronounced when determined by viscosity compared by DSC, with the CPN showing a higher transition temperature than PNIPAAm. The cross-sectional structure and surface topography of the hydrogels were examined by scanning electronic microscopy (SEM) and atomic force microscopy (AFM), respectively. The incorporation of chitosan further increased the entanglement of the hydrogel network. An increase in the chitosan ratio in the polymers (CPN-H) also increased the cross-linking structure. A smoother surface of hydrogel matrices was observed for CPN compared with PNIPAAm. All hydrogels tested significantly reduced drug release compared with an aqueous solution. The release rate of platinum drugs from PNIPAAm was retarded at the late stage. CPN matrices could continuously deliver platinum drugs during the experiment. The rate of release from CPN-H was generally slower than that from hydrogels and had a lower chitosan ratio (CPN-L), presumably due to the more-tortuous pathways in the hydrogels. Thermosensitive hydrogels like those prepared in this study may be a promising carrier for the delivery of platinum drugs, as the drug release can be controlled and sustained using CPN networks.
INTRODUCTION
Cisplatin is one of the most potent anticancer agents for treating testicular, ovarian, head, neck, skin, and lung cancer (Xiao et al. Citation2004). Because of its notable renal and auditory toxicity, cisplatin has almost completely been withdrawn from clinical trials (Avgoustakis et al. Citation2002). Carboplatin is another platinum drug that has been used as an alternative to cisplatin, mainly because of its lower non-hematological toxicity (Desoize and Madoulet Citation2002). All platinum derivatives available for clinical use are administered intravenously. However, the problems associated with the intravenous administration of platinum drugs are the systemic side-effects, low drug concentrations at the cancerous site, and short half-life (Konishi et al. Citation2003). Polymeric hydrogels have been extensively explored as drug delivery carriers with the goal of achieving higher drug concentrations at the tumor tissue and better controlled-release profiles for extended time periods. Much interest has been focused on polymer systems that show a phase transition in response to temperature (Yoshizawa et al., Citation2005). This stimulus-responsive property is particularly useful for the targeted delivery of anticancer drugs (Liu et al. Citation2004).
Poly(N-isopropylacrylamide) (PNIPAAm) is widely used to prepare hydrogels that show a transition from the swollen to the collapsed state at temperatures above 32°C. However, the clinical applications of PNIPAAm are limited due to the carcinogenic or teratogenic toxicity of the monomer used in its synthesis (Yoshizawa et al. Citation2005). Natural polymers are desirable for biomedical applications because of their proven biocompatibility and biodegradability (Bhattarai et al. Citation2005). Chitosan has been incorporated with PNIPAAm in a grafted or interpenetrating form to prepare thermosensitive hydrogels (Kim et al. Citation2000; Wang et al. Citation2001; Lee et al. Citation2004b). This combination of synthetic and natural polymers can help maintain the temperature sensitivity of PNIPAAm, while improving its biocompatibility and biomedical properties. This copolymer is used as a drug delivery system for caffeine and pilocarpine (Lee et al. Citation2004a). The swelling rates of the interpenetrating network for reaching an equilibrium state are relatively slow (Wang et al. Citation2001). The direct grafting method using NIPAAm monomers and grafting agents has the disadvantages of excessive formation of PNIPAAm homopolymers (Prabaharan and Mano Citation2006). Moreover, the properties of chitosan can be changed due to the need to open the chitosan ring structure. The PNIPAAm chains of this present study were first prepared and then grafted by forming amide linkages with chitosan as an efficient way to graft and control the grafted regions and chain length (Lee et al. Citation2004c). The purpose of this study was to elucidate the effect of polymer ratios on the characteristics and platinum drug release rates of chitosan-based hydrogels. The sol-gel transition temperature by DSC and viscosity, equilibrium water content, morphological examination, as well as release abilities of cisplatin and carboplatin were studied for hydrogels prepared with different ratios of grafted polymers.
MATERIALS AND METHODS
Materials
Cisplatin, carboplatin, α -azobis-isobutyronitrile (AIBN), 2-morpholineolinoethane sulphonic acid (MES), and N-isopropylacrylamide (NIPAAm) were obtained from Sigma Chemical (St. Louis, MO, USA). 1-Ethyl-3-(3-dimethylaminopropyl)-carbodiimide (EDC) and N-hydroxylsuccinimide (NHS) were obtained from Acros Organics (Geel, Belgium). Chitosan (with a molecular weight [MW] of 150 kDa and a deacetylation degree of 98%) and mercaptoacetic acid (MAA) were purchased from Fluka Chemie (Buchs, Germany).
PNIPAAm Synthesis
The recrystallized monomer (50 g) of N-isopropylamide (NIPAAm) was dissolved in 250 ml of benzene with 10 mol% of NIPAAm MAA and 0.5 mol% of NIPAAm recrystallized in AIBN. After polymerization by stirring in a water bath at 60°C for 24 h under positive nitrogen pressure, the solvent was evaporated. The crude solid was vacuum-dried, dissolved in acetone, and precipitated by the dropwise addition of ether. After being filtered and dried, the polymer was dialyzed against double-distilled water (ddH2O) for 7 days (Sepectra/pro3, MW cutoff 3500) and then freeze-dried, and the polymer was obtained. The number average MW of the polymer was determined by an end-group analysis (Stevens Citation1990).
CPN Synthesis
PNIPAAm (5 g) and chitosan (0.25 for CPN-L and 1 g for CPN-H) were dissolved in 50 ml of 0.1 M MES (pH 5.0). EDC and NHS were added to the above solution. The stoichiometric ratio of -COOH in PNIPAAm/EDC/NHS was 1:10:50. The conjugation reaction was carried out for 12 h. Then a 3 M NaCl (30 ml) solution was added to a water bath at 50°C for 30 min, centrifuged (at 13,500 rpm and 40°C) for 20 min, and diluted and dissolved in MES buffer (50 ml). The above steps were repeated three times. The resulting product was dialyzed for 4 days (Cellu Sep H1, MW cutoff 50,000) and then freeze-dried, and CPN-L and CPN-H were yielded at 63.2% and 80.5%, respectively.
Hydrogel Preparation
The synthetic polymers were mixed with ddH2O to a concentration of 15% (w/w). The resulting solution was left overnight in a refrigerator at 4°C. The mixtures were allowed to reach room temperature (∼ 25°C) before the experiments.
Measurement of Equilibrium Water Content
Aqueous solutions (1 ml) of polymers were gelled in a oven at 37°C for 1 h. Then, 1 ml of ddH2O was added to the samples. Twenty-four hours later, the solid matrices of the gels were removed and weighed. The weight was recorded, and the hydration ratio of the test samples was calculated from the following expression:
where WH is the weight of the hydrated gel and W0 is the weight of the dried test sample.
Differential Scanning Calorimetric (DSC) Analysis
The DSC analysis was carried out on a Q10 DSC (TA Instruments, New Castle, DE, USA). The thermal analysis profiles of the aqueous solutions of the polymers were obtained as the temperature was increased from 25 to 45°C at a rate of 2°C/min under nitrogen.
Sol-Gel Phase Transition Determined by Viscosity
The temperature effect on hydrogel gelation was studied by measuring the viscosity as a function of temperature from 25 to 34°C. The viscosities of these systems were determined using a Carri-Med CSL2 100 rheometer (TA Instruments). The diameters of both the cone and plate spindle were 60 mm. The determination mode was set to a flow-step fashion, with shear rates from 0 to 1000 1/s. The shear stress was set to 2 Pa.
Scanning Electronic Microscopic (SEM) Examination
Hydrogels were frozen at −80°C and then lyophilized by a freeze-drying method. Samples were fractured in liquid nitrogen and sputter-coated with gold. The resulting dried samples were examined using a Hitachi S-2400 SEM (Tokyo, Japan). All analyses were performed in a blinded fashion.
Atomic Force Microscopic (AFM) Examination
The surface image of the matrix of the hydrogels was examined by AFM. The hydrogel matrix (without excess water) was dried in a vacuum, after which the samples were examined using an AFM (OBJ-204C, Industrial Technology Research Institute, Hsinchu, Taiwan) using a commercial Si3N4 probe tip. Surface images with a scanning size of 11 × 11 μ m were obtained by a tapping mode. The scanning speed was 1.2 s/line.
In Vitro Release of Platinum Drugs
Drug release from hydrogels was measured using a Franz diffusion cell. A cellulose membrane (Spectrapor®3, with a MW cutoff of 3500, Spectrum Laboratories, USA) was mounted between the donor and receptor compartments. The donor medium consisted of 0.5 ml of a hydrogel formulation (with 1.67 μ mol cisplatin or carboplatin). The receptor medium consisted of 5.5 ml of pH 7.4 citrate-phosphate buffer. The available diffusion area between cells was 1.13 cm2. The stirring rate and temperature were maintained at 600 rpm and 37°C, respectively. At appropriate intervals, 300 μ l aliquots of the receptor medium were withdrawn and immediately replaced with an equal volume of fresh buffer. The amount of drugs was determined by measuring the absorbance by graphite furnace (flameless) atomic absorption spectrophotometry (Z-5000, Hitachi, Japan).
Drug Loading Percentage
Aqueous solutions (1 ml) of polymers were gelled in a oven at 37°C for 1 h. The supernatant (aqueous phase) was withdrawn from the network. The drug content in the supernatant was determined by atomic absorption spectrophotometry. The drug loading percentage was calculated as follows:
where WT is the total drug content in the gel and WS is the drug content in the supernatant.
Statistical Analysis
Statistical analysis of differences between different treatments was performed using an unpaired Student t-test. Statistical significance was set at p < 0.05. An analysis of variance (ANOVA) test was also used.
RESULTS
Polymer Synthesis
PNIPAAm was first synthesized by adding the -COOH group for further conjugation with chitosan. There is only one carboxyl group in every PNIPAAm molecule. The MW of PNIPAAm can be estimated by the titration method. As shown in , the MW of PNIPAAm was ∼ 0.11 × 105 Da as determined by the end-group analysis (Stevens Citation1990). The carboxyl group of PNIPAAm and the amine group of chitosan were conjugated to form an amide group in the synthesis of CPN. The structure of the CPN polymer was identified by FTIR in our previous report (Chen and Cheng Citation2006). The efficiency of grafting (%) was calculated by the equation: [(WCPN- WC)/WPNIPAAm] × 100, where WCPN is the weight of the freeze-dried graft copolymer, and WC and WPNIPAAm are the weights of chitosan and PNIPAAm in the feed, respectively. The results are summarized in . The estimated MWs of CPN-L and CPN-H were 19.9 × 105 and 7.2 × 105 Da respectively based on the grafting ratio of immobilized PNIPAAm to the initial chitosan (Lee et al. Citation2004b). The grafting ratio was calculated as (WCPN- WC)/MWPNIPAAmdivided by WC/MWC. The MW of CPN-L was greater than that of CPN-H. Since chitosan forms the substrate backbone of CPN polymers, a greater amount of PNIPAAm could graft onto a chitosan molecule in the case of CPN-L, which had a higher PNIPAAm/chitosan ratio.
TABLE 1 The efficiency of grafting, estimated molecular weight (MW), and water content of thermosensitive hydrogels
Measurement of the Equilibrium Water Content
The water contents (hydration ratio) of the polymers in a 15% (w/v) concentration in aqueous solution are shown in . CPN could retain more water in the structure compared to PNIPAAm by three- to four fold. CPN-H showed the highest hydration capacities among all of the polymers tested.
Sol-Gel Transition of the Hydrogels
The hydrogel phase transition was characterized through changes in the thermodynamic function by DSC and viscosity. shows DSC thermograms of the hydrogels. The hydrogels exhibited a single peak of heat capacity in the temperature range of the phase transition. PNIPAAm showed an endothermic peak at 31.83°C (). Significant endothermic peaks were also observed for CPN at ∼ 32°C.
FIG. 1 Differential scanning calorimetric (DSC) heating curves of PNIPAAm, CPN-L, and CPN-H hydrogels (15%, w/w). The curves are displaced along the ordinate for better visualization.
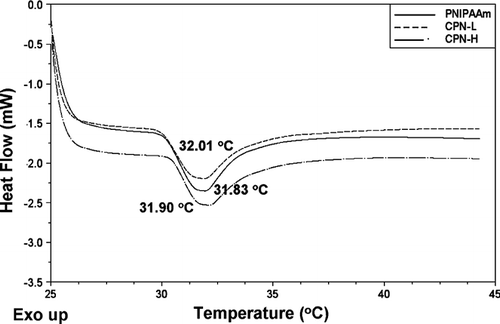
illustrates the temperature-dependent sol-gel transition of PNIPAAm, where an abrupt increase in viscosity at ∼ 31.6°C marks the onset of the gelation process. The gelling temperature increased in the order of CPN-H > CPN-L > PNIPAAm. It was found that hydrogels with a higher ratio (CPN-H, 33.1°C) gelled more slowly than those with a lower ratio (CPN-L, 32.6°C).
Morphology of Hydrogels by SEM and AFM
The material structures of cross-sections of the hydrogels were examined by SEM as shown in . The image clearly shows the appearance of a highly-porous structure in the PNIPAAm hydrogel (). and show the structures of CPN-L and CPN-H, respectively. One remarkable characteristic of CPN is the deep pores. The micrograph shows that the spherical pores were well interconnected throughout the scaffold matrix. The network structure of CPN-L was less condensed than that of CPN-H.
FIG. 3 Cross-sectional scanning electron microscopic (SEM) images (magnification, ×1000) of hydrogels after the freeze-drying process composed of (A) PNIPAAm, (B) CPN-L, and (C) CPN-H at a concentration of 15% (w/w).
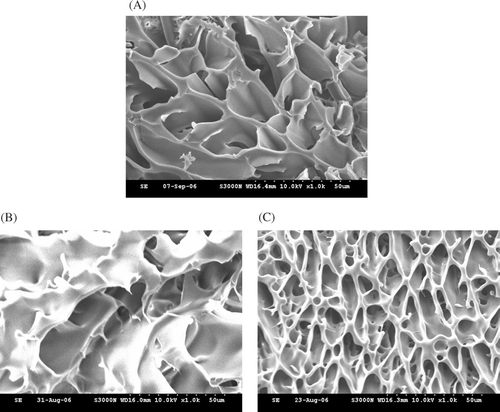
The outer surface topography was further determined by AFM. AFM is known to be a powerful tool for studying the surface properties of polymers in aqueous environments. shows the surface topographic images of these hydrogels of transversely scanned 11-μ m-wide regions. The roughness was determined by a relative height value, which was calculated by the distance between the highest and lowest regions. The results showed a significantly more-irregular surface for the PNIPAAm hydrogel surface compared to the CPN systems (p < 0.05).
In Vitro Release of Platinum Drugs
The in vitro cisplatin release from thermosensitive hydrogels of different polymers is given in . An aqueous solution of ddH2O was used as the control. The release of cisplatin from aqueous solution was virtually complete at 5 h. The entrapment of cisplatin in the hydrogels resulted in a significant reduction in drug release (p < 0.05). Since the thermosensitive hydrogels were divided into the polymer matrices and the aqueous space outside the matrices above the LCST, the drug loading percentage in the polymer matrices was further examined as shown in . The CPN-H and PNIPAAm hydrogels, respectively, showed the highest and lowest cisplatin entrapment levels (p < 0.05) among the hydrogels tested. The rate of release could be divided into an initial phase (0 to 2 h) and a terminal phase (2 to 5 h), with the initial phase of cisplatin release being faster than the terminal phase. Approximately 13%∼ 20% of the cisplatin was released in the first 3 h, and thereafter no or only a little release was observed. The trend of cisplatin release increased in the order of CPN-L ≥ CPN-H > PNIPAAm.
FIG. 5 Release percentage (%)-time profiles of cisplatin across a cellulose membrane from PNIPAAm, CPN-L, and CPN-H hydrogels (15%, w/w). Each value represents the mean ±SD (n = 4).
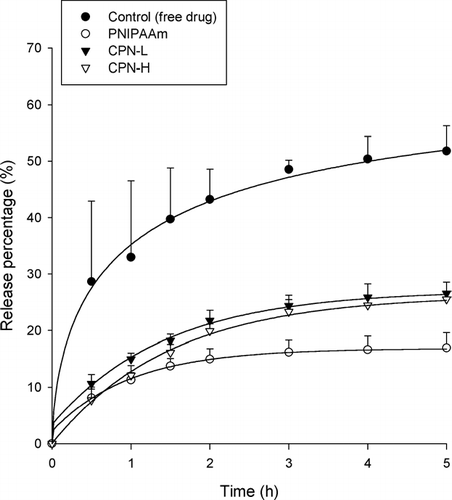
TABLE 2 Drug loading percentage (%) in the matrices of thermosensitive hydrogels with 15% polymers
A similar trend of cisplatin release was observed as for carboplatin (). It was seen that ∼ 80% of the carboplatin was released from the aqueous solution (control), whereas only 60%∼ 70% of the carboplatin was released from the hydrogels. Hydrogels showed comparable entrapment extents for both cisplatin and carboplatin (p > 0.05, ).
DISCUSSION
The predominant polymer exhibiting thermoresponsive ability of hydrogels examined in this study was PNIPAAm. PNIPAAm exists as individual chains with a coil conformation at temperatures below 32°C, but undergoes a sharp coil-to-globule transition at higher temperatures to form inter- and intra-chain associations, resulting in precipitation (Ohya et al. Citation2001). The strong hydrophobic-hydrophobic interactions of PNIPAAm indicates the strong tendency for the polymer to self-aggregate (Liu et al. Citation2004), resulting in the low water content of the PNIPAAm matrix. CPN showed a greater water content compared to PNIPAAm. The addition of hydrophilic polymers can increase water uptake and swelling (Kashyap et al. Citation2005). The increased -CONH- groups in CPN are regarded as being highly hydrated. The hydration force of chitosan allows water molecules to penetrate into the hydrogel network (Li and Xu Citation2002). The high proportion of chitosan in CPN-H seems to be responsible for its high level of hydration.
It has been suggested that lower critical solution temperature (LCST) behavior is caused by a critical hydrophilic/hydrophobic balance of polymer side groups. The DSC endothermic peak of PNIPAAm slightly increased following the incorporation of chitosan. Grafting of hydrophilic polymers reduces the amount of hydrophobic groups and increases the hydrophilicity of the system. This leads to an increase in the LCST, since the hydrophobic interactions, which increase with temperature, are compensated at higher temperatures by an increase in polymer-water interactions. However, all hydrogels tested showed a temperature for the phase transition close to that of the pure PNIPAAm hydrogel. It is reasonable to believe that the intrinsic properties of PNIPAAm subchains in CPN, in particular the transition free energy, are very close to those of the reference hydrogel (Alvarez-Lorenzo et al. Citation2005).
Discrepancies in LCST of the polymer systems determined by viscosity were larger than those determined by DSC. This indicates that the gelling temperature of the hydrogels differed from that for breaking hydrogen bonds between water molecules surrounding hydrophobic moieties on the polymer. The increased physical cross-linking in the polymer structure induces a higher sol-gel transition temperature (Huh et al. Citation2004; Liu et al. Citation2004). This slower response of CPN is probably due to the grafted structure, which sterically hinders the self-aggregation of PNIPAAm. CPN showed a significantly positive charge. The charged group in the polymer structure may produce strong interactions with water (Feil et al. Citation1993). This contributed to the higher gelling temperature of CPN than PNIPAAm. This may also indicate that although the hydrogen binding was broken early as determined by DSC, aggregates of CPN hydrogels were subsequently formed. CPN-H showed a higher gelling temperature than did CPN-L. This may have been due to the higher charges of the CPN polymers with the higher chitosan content.
CPN exhibits a cross-linked structure with smaller pores than PNIPAAm. The possible chain entanglements likely further enhance the formation of the hydrogel network (Li and Xu Citation2002). The surface roughness of devices in direct contact with living systems influences their biological reactivity and effectiveness. Because the sample for AFM does not need to be electrically conductive, no metallic coating is required as for SEM. Therefore, some of the surface irregularities observed under conventional SEM that are artifacts of the critical point dehydration and subsequent metallic coating process, were not seen in AFM samples (González-Méijome et al. Citation2006). All surfaces of hydrogels had a nano-ordered concavo-convex structure over the scanning area. As illustrated in , the incorporation of chitosan in the hydrogels produced a smoother surface. A flatter surface may result in a relatively lower surface area, which may prolong the residence time of the hydrogels in the biological systems because of the reduced frequency of hydrolysis and disintegration.
A Franz cell was used to examine the release of drugs as in the other studies (Kierstan et al. Citation2001; Morales et al. Citation2004). Approximately 50% of the cisplatin in the aqueous solution (control) was released to the receptor by the end of the experiment (5 h). The limited amount released may have been due to the use of the Franz diffusion assembly. Since drugs are released to a definitive space of the receptor (5.5 ml in this study), the drug loading in the receptor medium is limited (Fang et al. Citation2006). There may no longer be a concentration gradient between the donor and receptor. Although the Franz cell showed limited loading in the receptor, this method is still useful for differentiating the release capabilities of various formulations. Cisplatin release leveled off during 5 h. This result is consistent with those of a previous study, in which cisplatin showed rapid diffusion characteristics (Avgoustakis et al. Citation2002).
Cisplatin and carboplatin appeared to show a rapid initial (burst) release from the hydrogels. This was consistent for all formulations, and is most likely due to the untrapped drugs outside the polymer matrices and the drugs loosely entrapped in the hydrogel matrices upon incubation (Kim and Park Citation2002). The initial rapid release may have functional use in providing an initial dose during drug delivery, minimizing the lag period (Lim et al. Citation2000). This phase is followed by a controlled-release terminal phase. The PNIPAAm hydrogel showed the lowest cisplatin release. There was almost no further cisplatin or carboplatin released from the PNIPAAm systems after the initial burst effect. On the other hand, CPN continuously delivered platinum drugs until the end of the release experiment. This suggests the sustained release formulation for drugs in CPN. As observed in , the low cisplatin entrapment in the PNIPAAm matrix did not produce high drug release. This indicates that there was no relationship between drug release and entrapment efficiency. The release of drugs from thermosensitive formulations is probably controlled by diffusion as a result of partitioning between the matrices and the outer aqueous phase at 37°C (Soga et al. Citation2005). Cisplatin occupied the water channels of the hydrogel matrices from which it was released by diffusion along the concentration gradient between the matrices and outer solution. Although cisplatin was easily released by the unentrapped form from the outer solution to the receptor medium, it is difficult for cisplatin to be released from the PNIPAAm matrices to the outer solution because of the lower drug concentration in the inner matrices compared to the outer space.
In general, the effective free volume of polymer hydrogels is derived from the free volume of water, and the transport of solutes is presumed to permeate through the free water region (Fang et al. Citation1998; Yoshizawa et al. Citation2005). The small amount of water present in the hydrogel matrices may produce low drug diffusion. The lowest water content of the PNIPAAm matrix may also possibly have resulted in slow cisplatin release. The kinetics of drug release can potentially be controlled by exploiting drug-hydrogel interactions. It is reported that cisplatin is coordinately associated with carboxyl groups of polymer materials (Zhang et al. Citation2002; Konishi et al. Citation2003). PNIPAAm hydrogels have many carboxyl groups in their molecules. As a result, firmer interactions between cisplatin and PNIPAAm would reduce the amount of cisplatin initially released by simple diffusion.
Cisplatin is a platinum-chelated complex with four ligands: two ammonias and two chlorides. According to previous studies (Yokoyama et al. Citation1996; Yotsuyanagi et al. Citation2002), the two chloride ligands are gradually substituted with H2O molecules in the presence of water. Both Pt(NH3)2Cl(OH2)+ and Pt(NH3)2Cl(OH2)22 + can be formed by the aqueous complex based on the cisplatin dose used in this study. The CPN backbone has a high positive charge density. The repulsion forces between the cationic cisplatin and CPN hydrogels may promote the escape of cisplatin by diffusion from the system. Carboplatin is produced by substituting 1,1-cyclobutane dicarboxylate. Hence carboplatin cannot form the aqueous complex. Nevertheless, the trend of the release kinetics of carboplatin was similar to that of cisplatin. This phenomenon confirms that repulsion forces between the drug and hydrogel are not the sole factor influencing drug release. It is conceivable that an increase in the extent of cross-linking decreases the mobility of polymer molecules, resulting in reduced susceptibility of cisplatin to interactions with polymers (Konishi et al. Citation2005).The rate of drug release depends on the polymer ratios, decreasing with an increase in the proportion of chitosan in CPN. This may be attributable to the material density. With a denser matrix and greater pore numbers, CPN-H may produce tortuous pathways through which platinum drugs are released within the hydrogels.
Carboplatin release in the aqueous solution (control) reached a maximum at 24∼ 30 h. Carboplatin is more lipophilic than cisplatin (Screnci et al. Citation2000). Moreover, formation of the aqueous complex may further increase the hydrophilicity of cisplatin. A previous study demonstrated that the release of a series of drug derivatives is a function of the hydrophilicity, with slower and more-sustained release with more-lipophilic drugs (Luo et al. Citation2000). The reduction in carboplatin release through the use of hydrogels was not as pronounced compared to that of cisplatin release. Based on the theory cited by Topp and colleagues (Joshi and Topp Citation1992; Sung and Topp Citation1995), lipophilic solutes may diffuse through hydrophobic as well as hydrophilic pathways in a hydrated polymer environment. The effect of hydrogel entrapment on drug release was thus reduced. This suggests that the major permeation pathway of solutes with a hydrophilic nature such as cisplatin is the hydrated portion of the hydrogel.
CONCLUSIONS
It has been well established that the ratios of different polymers for grafting play an important role in controlling their physicochemical properties and drug delivery characteristics. Injectable, thermosensitive hydrogels were fabricated by chemically grafting PNIPAAm and chitosan in different ratios. Cisplatin and carboplatin were used as model drugs. The transition temperature determined by DSC was not significantly affected by the addition of the chitosan networks. However, the sol-gel temperature determined by viscometry increased after chitosan incorporation. A porous network of the cross-section of the hydrogels with a flat surface appearance was observed. CPN-H showed a denser cross-linked structure with more-tortuous pathways compared to CPN-L. The release of platinum drugs increased in the order of CPN-L ≥ CPN-H > PNIPAAm. There was even no increase in the amount of drug released from PNIPAAm hydrogels at the late stage of the release experiments. The water content, drug concentration gradient between matrices and outer aqueous solution, charge density, and polymer backbone mobility may have contributed to the trends observed in drug release from the different hydrogels. The results in this present study demonstrate that the delivery of cisplatin and carboplatin can be well controlled by incorporating them into different hydrogel systems.
REFERENCES
- Alvarez-Lorenzo C., Concheiro A., Dubovik A. S., Grinberg N. V., Burova T. V., Grinberg V. Y. Temperature-sensitive chitosan-poly(N-isopropylacrylamide) interpenetrated networks with enhanced loading capacity and controlled release properties. J. Control. Release 2005; 102: 629–641
- Avgoustakis K., Beletsi A., Panagi Z., Klepetsanis P., Karydas A. G., Ithakissios D. S. PLGA-mPEG nanoparticles of cisplatin: in vitro nanoparticle degradation, in vitro drug release and in vivo drug residence in blood properties. J. Control. Release 2002; 79: 123–135
- Bhattarai N., Matsen F. A., Zhang M. PEG-grafted chitosan as an injectable thermoreversible hydrogel. Macromol. Biosci. 2005; 5: 107–111
- Chen J. P., Cheng T. H. Thermo-responsive chitosan-graft- poly(N-isopropylacrylamide) injectable hydrogel for cultivation of chondrocytes and meniscus cells. Macromol. Biosci. 2006; 6: 1026–1039
- Desoize B., Madoulet C. Particular aspects of platinum compounds used at present in cancer treatment. Crit. Rev. Oncol. Hematol. 2002; 42: 317–325
- Fang E., Cheng Q., Lu X. B. Kinetics of in vitro drug release from chitosan/gelatin hybrid membranes. J. Appl. Polym. Sci. 1998; 68: 1751–1758
- Fang J. Y., Chen J. P., Leu Y. L., Wang H. Y. Characterization and evaluation of silk protein hydrogels for drug delivery. Chem. Pharm. Bull. 2006; 54: 156–162
- Feil H., Bae Y. H., Feijen J., Kim S. W. Effect of comonomer hydrophilicity and ionization on the lower critical solution temperature of N-isopropylacrylamide copolymers. Macromolecules 1993; 26: 2496–2500
- González-Méijome J. M., López-Alemany A., Almeida J. B., Parafita M. A., Refojo M. F. Microscopic observation of unworn siloxane-hydrogel soft contact lenses by atomic force microscopy. J. Biomed. Mater. Res. B: Appl. Biomater. 2006; 76: 412–418
- Huh K. M., Cho Y. W., Chung H., Kwon I. C., Jeong S. Y., Ooya T., Lee W. K., Sasaki S., Yui N. Supramolecular hydrogel formation based on inclusion complexation between poly(ethylene glycol)-modified chitosan and α -cyclodextrin. Macromol. Biosci. 2004; 4: 92–99
- Joshi H. N., Topp E. M. Hydration in hyaluronic acid and its esters using differential scanning calorimetry. Int. J. Pharm. 1992; 80: 213–221
- Kashyap N., Kumar N., Ravi Kumar M. N. V. Hydrogels for pharmaceutical and biomedical applications. Crit. Rev. Therap. Drug Carrier Syst. 2005; 22: 107–149
- Kierstan K. T., Beezer A. E., Mitchell J. C., Hadgraft J., Raghavan S. L., Davis A. F. UV-spectrophotometry study of membrane transport processes with a novel diffusion cell. Int. J. Pharm. 2001; 229: 87–94
- Kim M. R., Park T. G. Temperature-responsive and degradable hyaluronic acid/Pluronic F-127 composite hydrogels for controlled release of human growth hormone. J. Control. Release 2002; 80: 69–77
- Kim S. Y., Cho S. M., Lee Y. M., Kim S. J. Thermo- and pH-responsive behaviors of graft copolymer and blend based on chitosan and N-isopropylacrylamide. J. Appl. Polym. Sci. 2000; 78: 1381–1391
- Konishi M., Tabata Y., Kariya M., Hosseinkhani H., Suzuki A., Fukuhara K., Mandai M., Takakura K., Fujii S. In vivo anti-tumor effect of dual release of cisplatin and adriamycin from biodegradable gelatin hydrogel. J. Control. Release 2005; 103: 7–19
- Konishi M., Tabata Y., Kariya M., Suzuki A., Mandai M., Nanbu K., Takakura K., Fujii S. In vivo anti-tumor effect through the controlled release of cisplatin from biodegradable gelatin hydrogel. J. Control. Release 2003; 92: 301–313
- Lee C. F., Wen C. J., Lin C. L., Chiu W. Y. Morphology and temperature responsiveness-Swelling relationship of poly(N-isopropylamide-chitosan) copolymers and their application to drug release. J. Polym. Sci. A: Polym. Chem. 2004a; 42: 3029–3037
- Lee J. W., Jung M. C., Park H. D., Park K. D., Ryu G. H. Synthesis and characterization of thermosensitive chitosan copolymer as a novel biomaterial. J. Biomater. Sci. Polym. Edn. 2004b; 15: 1065–1079
- Lee S. B., Ha D. I., Cho S. K., Kim S. J., Lee Y. M. Temperature/pH-sensitive comb-type graft hydrogels composed of chitosan and poly(N-isopropylacrylamide). J. Appl. Polym. Sci. 2004c; 92: 2612–2620
- Li J., Xu Z. Physical characterization of a chitosan-based hydrogel delivery system. J. Pharm. Sci. 2002; 91: 1669–1677
- Lim S. T., Martin G. P., Berry D. J., Brown M. B. Preparation and evaluation of the in vitro drug release properties and mucoadhesion of novel microspheres of hyaluronic acid and chitosan. J. Control. Release 2000; 66: 281–292
- Liu X. M., Wang L. S., Wang L., Huang J., He C. The effect of salt and pH on the phase-transition behaviors of temperature-sensitive copolymers based on N-isopropylacrylamide. Biomaterials 2004; 25: 659–5666
- Luo Y., Kirker K. R., Prestwich G. D. Cross-linked hyaluronic acid hydrogel films: new biomaterials for drug delivery. J. Control. Release 2000; 69: 169–184
- Morales M. E., Lara V. G., Calpena A. C., Doménech J., Ruiz M. A. Comparative study of morphine diffusion from sustained release polymeric suspensions. J. Control. Release 2004; 95: 75–81
- Ohya S., Sonoda H., Nakayama Y., Matsuda T. Thermoresponsive artificial extracellular matrix for tissue engineering: hyaluronic acid bioconjugated with poly(N-isopropylacrylamide) grafts. Biomacromolecules 2001; 2: 856–863
- Prabaharan M., Mano J. F. Stimuli-responsive hydrogels based on polysaccharides incorporated with thermo-responsive polymers as novel biomaterials. Macromol. Biosci. 2006; 6: 991–1008
- Screnci D., McKeage M. J., Galettis P., Hambley T. W., Palmer B. D., Baguley B. C. Relationships between hydrophobicity, reactivity, accumulation and peripheral nerve toxicity of a series of platinum drugs. Br. J. Cancer 2000; 82: 966–972
- Soga O., van Nostrum C. F., Fens M., Rijcken C. J. F., Schiffelers R. M., Storm G., Hennink W. E. Thhermosensitive and biodegradable polymeric micelles for paclitaxel delivery. J. Control. Release 2005; 103: 341–353
- Polymer Chemistry, Chapter 2, Molecular weight and polymer solutions, M. P. Stevens. Oxford University Press, New York 1990; 40–69
- Sung K. C., Topp E. M. Effect of drug hydrophilicity and membrane hydration on diffusion in hyaluronic acid ester membranes. J. Control. Release 1995; 37: 95–104
- Wang M., Fang Y., Hu D. Preparation and properties of chitosan-poly(N-isopropylacrylamide) full-IPN hydrogels. React. Funct. Polym. 2001; 48: 215–221
- Xiao C., Qi X., Maitani Y., Nagai T. Sustained release of cisplatin from multivesicular liposomes: potentiation of antitumor efficacy against S180 murine carcinoma. J. Pharm. Sci. 2004; 93: 1718–1724
- Yoshizawa T., Shin-ya Y., Hong K. J., Kajiuchi T. pH- and temperature-sensitive release behaviors from polyelectrolyte complex films composed of chitosan and PAOMA copolymer. Eur. J. Pharm. Biopharm. 2005; 59: 307–313
- Yokoyama M., Okano T., Sakurai Y., Suwa S., Kataoka K. Introduction of cisplatin into polymeric micelle. J. Control. Release 1996; 39: 351–356
- Yotsuyanagi T., Usami M., Noda Y., Nagata M. Computational consideration of cisplatin hydrolysis and acid dissociation in aqueous media: effect of total drug concentrations. Int. J. Pharm. 2002; 246: 95–104
- Zhang J. S., Imai T., Suenaga A., Odagiri M. Molecular-weight dependent pharmacokinetics and cytotoxic properties of cisplatin complex prepared with chondroitin sulfate A and C. Int. J. Pharm. 2002; 240: 23–31