Abstract
The major mechanism of removing cyanide from the body is its enzymatic conversion by a sulfurtransferase, e.g. rhodanese, to the less toxic thiocyanate in the presence of a sulfur donor. Earlier results demonstrated that externally administered encapsulated rhodanese significantly enhances the in vivo efficacy of the given sulfur donor. Present studies are focused on liposomal carrier systems encapsulating rhodanese. Physicochemical properties, e.g. membrane rigidity, size distribution, surface potential, osmolarity, and viscosity, were determined for various liposomal lipid compositions and hydrating buffers to establish in vitro stability and in vivo fate. Lipid composition was also optimized to achieve maximum encapsulation efficiency.
Introduction
Rhodanese (Rh) (thiosulfate: cyanide sulphurtransferase, E.C. 2.8.1.1) is one of the sulfurtransferases related to the biotransformation of cyanide (CN) to the less toxic thiocyanate (SCN) in the presence of a sulfur donor (CitationHimwich & Saunders, 1948; CitationBaskin et al., 1999). Rh was isolated first by Sörbo (CitationSörbo, 1953), and its kinetic mechanism was first evaluated by Volini and Westley (CitationVolini & Westley, 1996). The complete amino acid sequence was published in 1978 (CitationRussell et al., 1978), and a high resolution X-ray crystallographic structure was determined in 1983 (CitationHol et al., 1983). The Rh reaction—sulfane sulfur transfer to CN—is well understood at a detailed chemical level, including the conformational changes during catalysis (CitationChow et al., 1985).
One of the antidotal mechanisms against CN involves employment of externally administered highly purified recombinant CN-metabolizing enzymes with high catalytic activity encapsulated within an appropriate enzyme carrier (CitationWay et al., 1985). This antidotal approach is based on the idea of destroying the toxicant before it reaches the target organs. However, these enzymes need to be placed in a suitable delivery system so that the physiological disposition of the protein molecules can be controlled. Earlier investigations were focused on administration of free Rh and the sulfur donor thiosulfate (TS) directly into the bloodstream (CitationClemedson, Hultman, & Sorbo, 1954; CitationAtkinson, Rutter, & Sargeant, 1974; CitationFrankenberg, 1980; CitationWeber et al., 1962). Unfortunately, the free enzyme was rapidly destroyed by the body’s immune system, which makes the efficacy of this approach quite limited. The first efforts to overcome the limitations of the potential therapeutic value of such free enzymes with bacterial origin as antidotes were based on the results of CitationIhler et al. (1973). They first reported that enzymes can be entrapped successfully within resealed and annealed erythrocytes (red blood cells; RBCs) by hypotonic dialysis. In the 1970s and 1980s, loaded erythrocytes were used in prolonged anti-tumor therapy (CitationUpdike & Wakamiya, 1983), and enzyme (e.g. glucocerebrosidase) replacement therapy for the congenital metabolic disorder termed Gaucher’s disease (CitationBeutler et al., 1977). RBCs encapsulating metabolizing enzymes were successfully employed in CN antagonism (CitationWay et al., 1985; CitationLeung et al., 1991; CitationPetrikovics et al., 1994, Citation1995) and in organophosphate (OP) antagonism (CitationPei et al., 1994; CitationPei, Petrikovics, & Way, 1995). Although RBCs have an advantage of long circulation time (i.e. in humans, 92 days (CitationSilbert, 1983)), they are not considered to be a practical carrier system due to the necessity of prior blood typing, the fragility of the cells, and the need for a sophisticated encapsulation technique.
The sterically stabilized (stealth) liposomal carrier system (SL) has been shown to be a promising enzyme carrier candidate, based on its many favoruable features/parameters, and the previous positive results with organophosphorus (OP) hydrolyzing enzymes in OP antagonism (CitationPetrikovics et al., 1999, Citation2000, Citation2004). Liposomes are widely employed therapeutically for encapsulating various types of drugs: in the SL system, the drug molecules are encapsulated in biocompatible carrier vesicles that can circumvent the body’s immune defenses, thereby preventing rapid uptake by the macrophage cells of the reticuloendothelial system (CitationSzoka & Papahadjopoulos, 1980; Papahadjapoulos et al., Citation1991; CitationWoodle & Lasic, 1992; CitationAllen, 1994). Pharmacokinetic studies in animal models with various liposomal-encapsulated drugs show evidence of the enhanced residence time in the circulation over the unencapsulated ‘free’ drugs when administered intravenously (i.v.). Other properties of the SL system such as chemical and physical stability and in vivo tolerance also depend on the lipid composition, surface modification, size, and the dose. Generally, small liposomes rich in unsaturated acyl chains are well tolerated when injected i.v. at a dose of up to 300 mg/kg. However, at higher doses (∼ 1000 mg/kg) they induced transient plasma increase for certain liver enzymes in an animal model (CitationZomber, Bogin, & Barenholz, 1996).
Present studies are focused on the physicochemical characterization of the SL delivery systems for encapsulated Rh. These parameters are essential in order to generate information on their in vitro stability and in vivo fate determined by the molecular interactions between the given carrier system and the encapsulated Rh. Osmolarity, pH, viscosity, encapsulation efficiency, size distribution, and the surface potential of the liposomes were determined. The membrane rigidity and the impact of Rh on it were studied by electron- paramagnetic resonance (EPR) spectroscopy using spin probes. Optimization of the liposomal lipid composition and the hydrating buffer was also performed.
Materials and methods
Chemicals
All chemicals employed were of the highest purity commercially available: potassium cyanide, (TS), HEPES (free acid), ethanol, NaCl, concentrated hydrochloric acid, and NaOH were purchased from J.T. Baker, (Phillipsburg, NJ), formaldehyde and ferric nitrate were purchased from Fisher Scientific (Pittsburgh, PA), the liposome components (1-palmitoyl-2-oleoyl-sn-glycero-3-phosphocholine (POPC), 1, 2-dioleoyl-sn-glycero-3-phosphocholine (DOPC), 1,2-dipalmitoyl-sn-glycero-3-phosphocholine (DPPC), lecithin (LEC), cholesterol (CHOL) and Rh were purchased from Sigma-Aldrich, (St. Louis, MO), and 1,2-dipalmitoyl-sn-glycero-3-phosphoethanolamine-N-[methoxy(polyethylene glycol)-2000] ammonium salt (PEG-PE-2000) was purchased from Avanti Polar Lipids (Alabaster, AL).
Preparation of sterically stabilized liposomes encapsulating Rh (SL-Rh)
POPC, DOPC, DPPC, LEC, PEG-PE-2000, and CHOL dissolved in ethanol were applied in various molar ratios () in order to determine the optimal lipid composition. The liposomes were prepared by the thin-film hydration method (Papahadjapoulos et al., 1991). Rh, either in isotonic HEPES buffer (pH = 7.4–7.7) or in 5% (w/w) aqueous solution of glucose (GLU) (pH = 4.2–7.8), was added to the dry lipid films. Hydrating solutions for the experiments were prepared daily before each experiment. The Rh concentration varied in the range of 0.1–0.5 mg/ml, and the lipid concentration (lipids and CHOL together) was 10.0 mg/ml. Osmolarity of the hydrating solutions was measured by an Osmomat 030-D osmometer (Medizintechnik GmbH., Austria). In order to obtain a homogenous population of small unilamellar vesicles (SUV), the multilamellar vesicles (MLV) were extruded through polycarbonate filters (100 and 400 nm) with an extruder (Avanti Polar Lipids Inc. Alabaster, AL). Extrusions were repeated five times for each membrane unless otherwise indicated.
Figure 1. Relative Rh activity values for various liposomal compositions in HEPES buffer (pH = 7.4). (Error bars represent standard deviations). (1) 47.5% POPC + 47.5% CHOL + 5.0% PEG-PE-2000; (2) 57.0% POPC + 38% CHOL + 5.0% PEG-PE-2000; (3) 66.5% POPC+ 28.5% CHOL + 5.0% PEG-PE-2000; (4) 57.0% DPPC + 38% CHOL + 5.0% PEG-PE-2000; (5) 66.5% DPPC + 28.5% CHOL + 5.0% PEG-PE-2000; (6) 85.5% DPPC + 9.5% CHOL + 5.0% PEG-PE-2000; (7) 66.5% LEC + 28.5% CHOL + 5.0% PEG-PE-2000; (8) 85.5% LEC + 9.5% CHOL + 5.0% PEG-PE-2000.
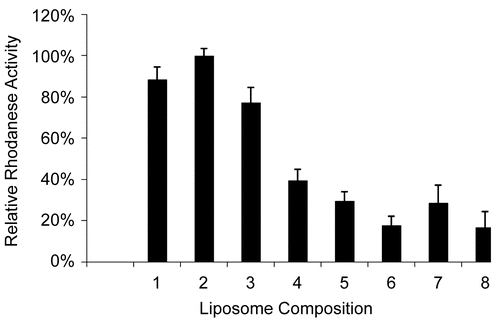
Determination of rhodanese activity
The formation of SCN was measured spectrophotometrically (Genesys 10UV, Thermo Electron Corporation, Waltham, MA) by the method of CitationWestley (1981), with minor modifications (CitationPetrikovics et al., 1995). One unit of Rh was defined as the amount of enzyme that forms 1 μmol of SCN in 1 min.
Optimal lipid composition determination for SL-Rh
Optimal lipid composition for SL-Rh was determined based on the highest enzyme activity achieved by the same encapsulation process. Un-encapsulated Rh was separated from SL-Rh by gel filtration on a G-100 Sephadex™ gel column (0.7cm × 10 cm) (GE Heathcare BioSciences AB, Sweden). Measurements were carried out in isotonic HEPES buffer at pH = 7.4. Rh activity for the fractions was determined as described above. Encapsulation efficiency (%) = (activity of SL-Rh/total Rh activity) × 100. Relative Rh activity (%) = activity of SL-Rh with a given lipid composition related to the highest activity achieved with the ‘optimal lipid composition’ × 100. (The relative Rh activity of the SL-Rh with the ‘optimal lipid composition” = 100). For the spectrophotometric assays, 50 μl liposomal samples were used. All measurements were performed at least in triplicate.
Viscosity measurements
Viscosities of the liposomal samples were measured at 20°C and 37°C with an AMVn automated microviscometer (Anton Paar Hungary Ltd., Budapest, Hungary) on 0.5 ml samples. The AMVn utilizes the rolling/falling ball principle to determine the dynamic viscosity of the test sample, and it is proportional to the time required for a ball to fall through the sample in a precise and temperature controlled glass tube (CitationCho, Hartnett, & Lee, 1984).
Size distribution and surface potential measurements
Mean diameter (zave) and polydispersity index (PDI) of the liposomal preparations were monitored by dynamic light scattering (DLS). The zeta-potential and size measurements were carried out with a Zetasizer 3000 HSA (Malvern Instruments, Westborough, MA). The instrument was equipped with a He-Ne laser operating at a wavelength of 633 nm. The size analysis was determined at a lipid concentration of 0.05 mg/ml, at 25°C. Samples were stored at 4°C between the measurements. Samples were diluted with freshly filtered (0.2 μm pore size, Corning, Corning Incorporated, Corning, NY) HEPES buffer or GLU before the measurement. The zeta-potentials for the enzyme-free and for Rh-containing liposomes were determined at a lipid concentration of 0.5 mg/ml.
EPR measurements
A spin labeled l-palmitoyl-2-stearoyl-(5-doxyl)-sn-glycero-3-phosphocholine (SL-SPPC-5) (Avanti Polar Lipids Inc. Alabaster, AL) was co-dissolved with the lipids in absolute ethanol. The molar ratio of the spin label-to-lipid molecules was 1:100. EPR spectra were generated with an EMX6 Bruker X-band on-line spectrometer (Billerica, MA). Temperature dependence of the spectra was measured by controlling the temperature within the sample with a precision of 0.1°C; while a 100 kHz modulation frequency and maximum 2 gauss modulation amplitude were used. The applied microwave power was 15 mW; and scan speeds of 167.77 or 335.5 s with 2048 points on 100 gauss field interval were set. To characterize the fluidity of the lipid membrane, the outer peak separation (2Azz) was determined. The greater is the 2Azz the more rigid is the lipid membrane, and vica versa.
Results
shows the relative Rh activity for liposomes with various lipid compositions when the same amounts of Rh (0.5 mg/ml) were encapsulated in each liposomal prepration with the same process. ‘Optimal lipid composition’ for SL-Rh was determined based on the highest enzyme activity measured among the liposomal samples. Relative activity (%) = activity of SL-Rh with a given lipid composition related to the highest activity achieved with the ‘optimal lipid composition’ multiplied by 100. Therefore, the relative activity of the SL-Rh with the ‘optimal lipid composition’ = 100. The maximum encapsulation efficiency was achieved when the liposomes were prepared with the lipid composition of POPC (57%), CHOL (38%) and PEG-PE-2000 (5%) with a hydrating buffer of HEPES at pH = 7.4. With LEC- or DPPC-containing liposomes, relatively low Rh activity was detected.
The rate of SCN formation as a function of the assay volume of SL-Rh when TS was employed as sulfur donor is shown in . The rate of SCN formation was directly proportional to the amount of encapsulated Rh in the system. SL without Rh did not enhance the formation of SCN from CN and TS.
Figure 2. Formation of SCN as a function of increasing amounts of SL-Rh. The rate of SCN formation was directly proportional to the amount of encapsulated enzyme in the system. Blank contained the same concentration of cyanide and thiosulfate without Rh or SL. Each data point is the average of triplicate assays; in all cases the experimental error was less than 10%. SL alone did not generate product formation.
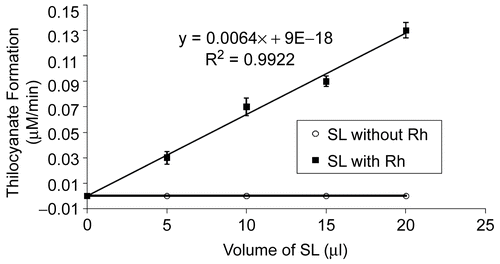
For all routes of administration, a pH-range of 4.5–8.0 is acceptable; and the normal physiologic osmolarity range is 280–310 mosmol/l. According to the measurements, the test preparations fulfilled the criteria needed for successful i.v. administration. The viscosity of the SUV samples varied in the range from 0.8011–1.2292 mPa.s for the 10.0 mg/ml lipid concentration, depending on temperature (20°C and 37°C) and on the presence of Rh (0.5 mg/ml) ().
Table 1. Viscosity of empty (control) and Rh-containing SUVs at two different temperatures in HEPES with the optimal lipid composition. Rh and lipid concentration were 0.5 mg/ml and 10.0 mg/ml, respectively.
To investigate possible molecular interactions between Rh and the lipid molecules in the region of polar lipid head-groups, the SL-SPPC-5 spin probe was used. Using EPR, the outer-peak separation of the spectra, 2Azz, was determined (). Neither the control nor the enzyme containing liposomes exhibited an abrupt change in their temperature— 2Azz curves. Regarding the experimental uncertainty (± 0.3 Gauss), the SL-SPPC-5 spin probe reflects a slight increase in the membrane rigidity in the presence of Rh in 0.5 mg/ml concentration across the entire temperature range examined ().
Figure 3. EPR spectra of liposomes in various hydrating buffers using SL-SPPC-5 spin label (pH = 7.4). Rh in a concentration of 0.5 mg/ml has only a slight rigidizing effect in case of the optimal liposomal composition (in both hydrating buffers).
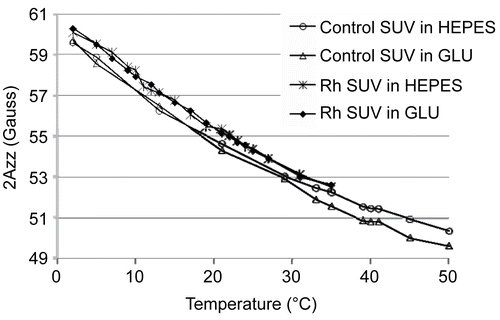
The mean diameter, size-distribution, and polydispersity index of the liposomal preparations were monitored by dynamic light scattering ( and ). Liposomes prepared with GLU hydration possessed a greater diameter (∼ 10–15 nm; data not shown) than the liposomes prepared with HEPES buffer of both the control and the Rh-containing liposomes. Comparing the size of liposomes prepared with 58% DOPC or 58% POPC as main lipid components, the DOPC-containing liposomes were found to be larger by ∼ 80 nm; however, the presence of Rh in a concentration of 0.1 and 0.3 mg/ml did not have a significant impact on the liposomal vesicle size ( and ). The PDI values were less than 0.1 for both the control and the Rh-containing liposomes.
Table 2. Average size (zave) and polydispersity index (PDI) of DOPC comprised liposomes passed through filters with various pore sizes and containing varying Rh concentrations in HEPES at pH = 7.4.
Table 3. Average size (zave) and polydispersity index (PDI) of POPC comprised liposomes passed through filters with various pore sizes and containing varying Rh concentrations in HEPES at pH = 7.4.
In GLU, the surface potential of the sterically stabilized control liposomes (optimal composition) was negative (from pH 4.2 to 8.0), while they were slightly positively charged in HEPES buffer in the pH range from 7.4 to 7.8 (). Rh did not have a significant impact on the surface charge of liposomes at the tested enzyme concentrations (0.1 and 0.3 mg/ml) in HEPES buffer ().
Table 4. The zeta-potential of sterically stabilized control (empty) liposomes in two different hydrating solutions (HEPES or GLU) at various pH values.
Table 5. The effect of Rh on the zeta-potential of sterically stabilized liposomes at two various Rh concentrations (0.1 and 0.3 mg/ml) at pH = 7.4 in HEPES.
Discussion
Physicochemical characterization of liposomal drug delivery systems is essential in order to gain information on their in vitro stability and in vivo fate. Our antidotal approach is based on the idea of destroying (or inactivating) the toxicant (e.g. CN) before it reaches the target organs. Application of this approach would result in a more effective therapy with less toxicity and could yield fewer side-effects than the most commonly used antidotal systems. Minimizing the immunologic reactions, e.g. encapsulation of the metabolizing enzyme, is crucial for making this approach efficient in vivo. Originally this approach has been planned for prophylactic applications, e.g. protecting troops in the field, or agricultural workers who can be exposed to various OP agents (CitationPei, Petrikovics, & Way, 1995; CitationPetrikovics et al., 1995). However, externally administered metabolizing (detoxifying) enzymes can also serve as efficient post-exposure treatments, since they are readily available in the circulation (CitationPetrikovics et al., 2004). In case of CN intoxication, if the sulfur donor is co-encapsulated with Rh, a controlled dose of sulfur donor/Rh can be provided to react with CN in the circulation right after the CN exposure. In vivo results with liposomal encapsulated Rh on therapeutic and phophylactic mice models will be published later. Present studies were focused on the preparation and physicochemical characterization of the sterically stabilized (stealth) liposomal carrier systems with previously optimized lipid composition. In the stealth liposomal system, the PEG-chains act as a steric repulsive force against opsonization by plasma proteins resulting in protection of the liposomes from recognition by the reticuloendothelial system (CitationOku & Namba, 1994). This steric hindrance is considered the main contributor to the extended liposome circulatory life. In addition, it is well accepted that the flexibility of the polymer chains on the surface of liposomes, as well as the surface charge and the hydrophilicity of the liposomes, are also important to ensure the stability of the liposomes (CitationSenior et al., 1991; CitationTakeuchi et al., 2001). The permeability properties of the liposomal bilayers for the co-administered sulfur donors play an important role in the detoxification of CN; therefore, sulfur donors with low cell penetration capability (e.g. TS) must be co-encapsulated with Rh. On the basis of the pKa of CN (pKa = 9.2) under physiological conditions (pH = 7.4), HCN has the ability to pass through any membranes, including liposomal bilayers, and after achieving an equilibrium between all the membranes in the body, it can rapidly be distributed to the various compartments. However, for a number of the sulfur donors, the varied liposomal compositions possessed different permeabilities.
In our current study, the optimal liposomal composition (highest encapsulation efficiency for Rh) was determined by applying various combinations of lipids possessing saturated and/or unsaturated fatty acid chains (). The natural LEC contains a mixed variety of saturated and unsaturated fatty acids with carbon chain lengths of ∼ 14–22 carbon atoms, thus providing a fluid membrane with a low phase transition temperature (∼ 2°C). On the contrary, DPPC is comprised of saturated fatty acids, giving it a rigid membrane and a higher phase transition temperature (41.5°C) (CitationBudai et al., 2004; CitationBéni et al., 2006). The POPC with its palmitoylic and oleoylic chains represents a midpoint between DPPC and LEC, because of its relatively high phase transition temperature (∼ 12°C) and consequently fluid membrane. Addition of CHOL, as an auxiliary material, decreases the rigidity of membranes below their phase transition temperature (e.g. for DPPC) and decreases the fluidity above the phase transition temperature (e.g. for LEC and POPC) (CitationZhao et al., 2007). Significant increases in the CN conversion were detected only for POPC-containing vesicles.
The encapsulated Rh did not have a significant impact on most of the examined physico-chemical parameters (size, surface potential) of the liposomes prepared. An increase in the 2Azz value in the presence of Rh indicates a slight decrease of membrane fluidity (CitationMarsh, 1981). For both control and Rh-loaded liposomal samples, it was clear that with increasing temperature, the fluidity of the bilayer increased (). The fact that neither the control nor the enzyme encapsulating liposomes exhibited an abrupt change in their temperature—2Azz curves indicates the absence of a gel-fluid phase transition in the temperature range studied. This effect may have been due to the presence of cholesterol in a relatively high concentration (38%), which was used to form the liposomes (CitationBudai et al., 2003; CitationBudai et al., 2004). The EPR results—on the basis of the increased 2Azz values in the presence of the enzyme—suggest that Rh can be localized not only in the aqueous interior of the liposomes, but can be immersed in the liposomal bilayer, thus leading to a membrane rigidity increase at the depth of the 5th carbon atom along the fatty acid chains.
The type of hydrating solution (HEPES or GLU) used to prepare the liposomes did not have a significant impact on the membrane fluidity.
The size analysis monitored by dynamic light scattering shows that the presence of Rh did not have a significant impact on the liposomal size ( and ). However, the hydrating solutions interfered with the size of both the control and the Rh-containing liposomes. In GLU the average hydrodynamic diameter of vesicles was slightly higher than the appropriate size of the same liposomal compositions in HEPES buffer at the same pH. In GLU the PEG chains seemed to be more ‘relaxed’ and lengthened, thus accounting for the higher hydrodynamic diameter. For i.v. administration, the smaller size would be more suitable; therefore, the use of HEPES is recommended. Furthermore, one must consider some more disadvantages of GLU: it is more complicated to maintain the pH-, and microbiological stability with GLU-containing liposomal systems than with HEPES buffer-containing systems.
Comparing the size of liposomes prepared with DOPC or POPC as the main lipid component, the DOPC-containing liposomes seemed to be more flexible, due to the high number of double bonds ( and ); However, liposomes prepared from POPC with less unsaturated fatty acid residues have more rigid, less deformable structures. The POPC-containing liposomes are smaller in size after pressing them through polycarbonate filters with an appropriate pore size ( and ). The smaller size provided by POPC- containing liposomes is more suitable to our present purpose, considering the special needs of intravenous administration where the recommended maximum vesicle size is ∼ 200 nm.
The observed difference in the surface potential for liposomes prepared with HEPES and GLU can be explained with the presence of charged ions in the HEPES buffer that can ‘cover’ the original surface charge (). Liposomes in HEPES possess a slightly positive charge, while in GLU they have pronounced negative charge. The ions of the HEPES buffer and the non-dissociated molecules of the non-ionic hydrating solution GLU behave in different ways in the environment of liposomal membranes. While the charged ions can ‘cover’ and ‘mask’ the original charge/surface potential of liposomal membranes, the GLU molecules do not have a significant impact. The presence of Rh was found to have no significant impact on the surface potential of the liposomes in HEPES buffer, indicating that the surface charge of Rh-SL does not play a pronounced role in preventing fusion and/or aggregation of the liposomal vesicles (). It is important to note that EPR and surface potential data are consistent with each other. The presence of Rh at the region of lipid head groups should not have to cause a significant change in the surface potential. The positive and negative ions of HEPES and NaCl partially can ‘cover’/‘mask’ the original surface charge of the liposomes, much like Rh.
Figure 4. The role of hydrating solution in the zeta-potential of liposomes. Liposomes in HEPES possess slightly positive charge, while in GLU they have pronounced negative charge.
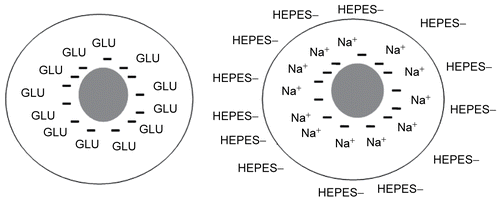
These findings support the in vivo suitability of the liposomal preparation encapsulating Rh for protecting against CN intoxication. Based on these results, the optimal liposomal composition for the encapsulation of Rh is POPC/CHOL/PEG-PE-2000 at a molar ratio of 57:38:5. The use of POPC vs DOPC in the optimal composition can be explained not only by the high encapsulation efficiency reached with the POPC-containing composition, but also with optimal size distribution provided by the POPC. The molar ratio of PEG-PE-2000 (5%) in the Rh-SL liposomal system is a sufficient amount for complete protection of the liposome surface, to prevent aggregation and/or fusion (CitationTorchilin & Papisov, 1994; CitationTorchilin et al., 1994). For future in vivo studies, co-encapsulation of TS with Rh is recommended to eliminate any permeability issues associated with TS.
Acknowledgments
This study was supported by NIH: NIAID/USAMRICD Interagency Agreements (Y1-A1-6176-01/02 and A120-B.P2006/7-01), and the ARMY MEDICAL RESEARCH INSTITUTE OF CHEMICAL DEFENSE (Dr Gary Rockwood) under the auspices of the US Army Research Office Scientific Services Program administered by Battelle (Delivery Order 0878, Contract No. DAAD19-02-D-0001, TCN 06-170 and 08284), and the Robert A. Welch Foundation (x-0011) at Sam Houston State University, Huntsville, TX.
Declaration of interest: The authors report no conflicts of interest. The authors alone are responsible for the content and writing of the paper.
References
- Allen, T.M. (1994). Long circulating (sterically stabilized) liposomes for targeted drug delivery. Adv Drug Del Rev, 13, 285–309.
- Atkinson, A., Rutter, D.A., Sargeant, K. (1974). Enzyme antidote for experimental cyanide poisoning. Lancet, 2, 1446.
- Baskin, S.I., Porter, D.W., Rockwood, G.A., Romano, J.A., Patel, H.C., Kiser, R.C., Cook, C.M., Tenay, A.L. (1999). In vitro and in vivo comparison of sulfur donors as antidotes to acute cyanide intoxication. J Appl Toxicol, 19, 173–83.
- Béni, S.Z., Budai, M., Noszál, B., Gróf, P. (2006). Molecular interactions in imatinib-DPPC liposomes. Eur J Pharm Sci, 27, 205–11.
- Beutler, E., Dale, G., Guinto, E., Kuhl, W. (1977). Enzyme replacement therapy in Goucher’s disease: Preliminary clinical trial of a new enzyme preparation. Proc Natl Acad Sci USA, 74, 4620–3.
- Budai, M., Szabó, Z.S., Szőgyi, M., Gróf, P. (2003). Molecular interactions between DPPC and morphine derivatives: a DSC and EPR study. Int J Pharm, 250, 239–50.
- Budai, M., Szabo, Z.S., Zimmer, A., Szogyi, M., Grof, P. (2004). Studies on molecular interactions between nalidixic acid and liposomes. Int J Pharm, 279, 67–79.
- Cho, Y.I., Hartnett, J.P., Lee, W.Y. (1984). Non-Newtonian viscosity measurements in the intermediate shear rate range with the falling-ball viscometer. J Non-Newtonian Fluid Mech, 15, 61–74.
- Chow, S.F., Horowitz, P.M., Westley, J., Jarabak, R. (1985). Evidence for dynamically determined conformational states in rhodanese catalysis. J Biol Chem, 260, 2763–70.
- Clemedson, C.J., Hultman, H.I., Sorbo, B. (1954). The antidotal effects of some sulfur compounds and rhodanese in experimental cyanide poisoning. Acta Physiol Scand, 32, 245–51.
- Frankenberg, L. (1980). Enzyme therapy in cyanide poisoning: effect of rhodanese and sulfur donor compounds. Arch Toxicol, 45, 315–23.
- Himwich, W.A., Saunders, J.P. (1948). Enzymatic conversion of cyanide to thiocyanate. Am J Physiol, 153, 348–54.
- Hol, W.G.J., Lijk, L.J., Kalk, K.H. (1983). The high-resolution three-dimensional structure of bovine liver rhodanese. Fundam Appl Toxicol, 3, 370–6.
- Ihler, G.M., Glew, R.H., Schnure, F.M. (1973). Enzyme loading erythrocytes. Proc Natl Acad Sci USA, 70, 2663–6.
- Leung, P., Cannon, E.P., Petrikovics, I., Way, J.L. (1991). In vivo studies on rhodanese encapsulation in mouse carrier erythrocytes. Toxicol Appl Pharmacol, 110, 268–74.
- Marsh, D. (1981). Electron spin resonance: spin labels. In Grell, E. (ed.), Molecular Biology Biochemistry and Biophysics, Membrane Spectroscopy. Berlin: Springer, pp. 51–142.
- Oku, N., Namba, Y. (1994). Long circulating liposomes. Crit Rev Therap Drug Carrier Syst, 11, 231–70.
- Papahadjapoulos, D., Allen, T.M., Gabizon, A., Mayhew, K., Matthay, K., Huang, S.K., Lee, K.D., Woodle, M.C., Lasic, D.D., Redemann, C., Martin, F.J. 1991. Sterically stabilized liposomes: improvements in pharmacokinetics and antitumor therapeutic efficacy. Proc Natl Acad Sci USA, 88, 11460–4.
- Pei, L., Omburo, G., McGuinn, W.D., Petrikovics, I., Dave, K., Raushel, F.M., Wild, J.R., DeLoach, G.R., Way, J.L. (1994). Encapsulation of phosphotriesterase within murine erythrocytes. Toxicol Appl Pharmacol, 124, 296–301.
- Pei, L., Petrikovics, I., Way, J.L. (1995). Antagonism of the lethal effect of paraoxon by carrier erythrocytes containing organophosphorous acid anhydrase. Fundam Appl Toxicol, 28, 209–14.
- Petrikovics, I., McGuinn, W.D., Cannon, E.P., Pei, L., Way, J.L. (1994). Encapsulation of rhodanese and organic thiosulfonates by mouse erythrocytes. Fundam Appl Toxicol, 23, 70–5.
- Petrikovics, I., Cannon, E.P., McGuinn, W.D., Pei, L., Way, J.L. (1995). Cyanide antagonism with organic thiosulfonates and carrier red blood cells containing rhodanese. Fundam Appl Toxicol, 24, 1–8.
- Petrikovics, I., Hong, K., Omburo, G., Hu, Q., Pei, L., McGuinn, W.D., Sylvester, D., Tamulinas, C., Papahadjopoulos, D., Jaszberenyi, J.C., Way, J.L. (1999). Antagonism of paraoxon intoxication by recombinant phosphotriesterase encapsulated within sterically stabilized liposomes. Toxicol Appl Pharmacol, 156, 56–63.
- Petrikovics, I., Hong, K., Papahadjopoulos, D., Yuzapavik, P., Jiang, J., Yin, R., Cheng, T.C., DeFrank, J.J., McGuinn, W.D., Sylvester, D., Way, J.L. (2000). In vitro studies on sterically stabilized liposomes (SL) as enzyme carriers in organophosphorus (OP) antagonism. Drug Deliv, 7, 83–9.
- Petrikovics, I., Papahadjapoulos, D., Hong, K., Cheng, T.C., Baskin, S.I., Jiang, J., McGuinn, W.D., Jaszberenyi, J.C., Szilasi, M., Way, J.L. (2004). Comparing prophylactic and therapeutic protection against the lethal effects of paraoxon. Toxicol Sci, 77, 258–62.
- Russell, J., Weng, L., Keim, P.S., Heinrikson, R.L. (1978). The covalent structure of bovine liver rhodanese. J Biol Chem, 253, 8102–8.
- Senior, J., Delgado, D., Fisher, D., Tilcock, D., Gregoriadis, G. (1991). Influence of surface hydrophilicity of liposomes on their interaction with plasma protein and clearance from the circulation: studies with poly(ethyleneglycol)-coated vesicles. Biochem Biophys Acta, 1062, 77–82.
- Silbert, J.A. (1983). The storage of washed human erythrocytes in a protein free medium. Transfusion, 23, 8–10.
- Sörbo, B.H. (1953). On the substrate specificity of rhodanese. Acta Chem Scand, 7, 1137–45.
- Szoka, F., Papahadjopoulos, D. (1980). Comparative properties and methods of preparation of lipid vesicles (liposomes). Ann Rev Biophys Bioeng, 9, 467–508.
- Takeuchi, H., Kojima, H., Yamamoto, H., Kawashima, Y. (2001). Evaluation of circulation profiles of liposomes coated with hydrophilic polymers having different molecular weights in rats. J Contr Rel, 75, 83–91.
- Torchilin, V.P., Papisov, M.L. (1994). Why do polyethylene glycol coated liposomes circulate so long? J Liposome Res, 4, 725–39.
- Torchilin, V.P., Trubetskoy, V.S., Milshteyn, A.M., Canillo, J., Wolf, G.L., Papisov, M.I., Bogdanov, A., Narula, J., Khaw, B.A., Omelyanenko, V.G. (1994). Targeted delivery of diagnostic agents by surface- modified liposomes. J Contr Rel, 28, 45–58.
- Updike, S., Wakamiya, R. (1983). Infusion of red blood cell-loaded asparaginase in monkey. Immunologic, metabolic and toxiologic consequences. J Lab Clin Med, 101, 679–91.
- Volini, M., Westley, J. 1966. The mechanism of the rhodanese-catalyzed thiosulfate–lipoate reaction: kinetic analysis. J Biol Chem, 241, 5168–76.
- Way, J.L., Leung, P., Ray, L., Saunder, C. (1985). Erythrocyte encapsulated thiosulfate sulfurtransferase. Biol Haematol, 51, 75–81.
- Weber, D., Friedberg, K.D., Lendle, L. (1962). Beurteilung therapeutisher Massnahmen bei der Blausaurevergiftung unter konstanter Cyanide-infusion. Naunyn-Schmiedebergs. Arch Exp Pathol Pharmakol, 224, 1–16.
- Westley, J. (1981). Thiosulfate: cyanide sulfurtransferase (rhodanese). Methods Enzymol, 77, 285–91.
- Woodle, M.C. (1993). Surface-modified liposomes: assessment and characterization for increased stability and prolonged blood circulation. Chem Phys Lipids, 64, 249–62.
- Woodle, M.C., Lasic, D.D. (1992). Sterically stabilized liposomes. Biochem Biophys Acta, 1113, 171–99.
- Zhao, L., Feng, S.S., Kocherginsky, N., Kostetski, I. (2007). DSC and EPR investigations on effects of cholesterol component on molecular interactions between paclitaxel and phospholipid within lipid bilayer membrane. Int J Pharm, 338, 258–66.
- Zomber, G., Bogin, E., Barenholz, Y. (1996). Effects of i.v. injection of small unilamellar liposomes of egg phophatidylcholine on cholesterol in plasma and erythrocytes, serum enzymes and liver function in dogs. J Liposome Res, 6, 455–77.