Abstract
To specifically deliver cytotoxic drug to tumor cells and enhance cellular uptake is the key for effective cancer therapy. In this paper, we described a novel drug targeting system, which is designed to combine features of biological (cell-penetrating peptides, CPPs) and physical (magnetic) drug targeting for use in the magnetic hyperthermia-triggered release. A doxorubicin–CPPs conjugate (DOX-CPPs) was loaded into thermosensitive magnetic liposomes (TSMLs) (DOX-CPPs/TSMLs), and in vitro DOX-CPPs thermosensitive release activity, anti-proliferation effect, in vivo targeted delivery as well as in vivo antitumor activity were determined. The results demonstrated that the DOX-CPPs/TSMLs showed good physicochemical properties, effective anti-proliferation effect in MCF-7 cells in vitro. Additionally, in vivo study, DOX-CPPs/TSMLs under AC magnetic field displayed superior in vivo targeted delivery efficacy, antitumor efficacy in an MCF-7 xenograft murine model. In conclusion, the application of DOX-CPPs/TSMLs under AC magnetic field may provide a strategy for the selective and efficient delivery of drug.
Introduction
The highly localized and specific delivery of chemotherapeutic drugs to a tumor site, without unwanted distribution in normal tissue, remains a major challenge for drug delivery. One strategy to achieve high local concentrations of drugs in tumor tissues makes use of targeting ligands that guide the therapeutic agents to antigens or receptors uniquely expressed or over-expressed on the target cells relative to normal tissues. However, such active targeting may sometimes suffer from certain limitations due to low degrees or heterogeneity in receptor expression among different tumor cells, or differences in ligands-receptor affinity and receptor-mediated internalization rates (Kirpotin et al., Citation2006). For example, although very effective in vitro, folate receptor targeted delivery failed to result in increased drug concentrations in tumors when compared to a non-targeted stealth liposome formulation like Caelyx®/DOXIL® in vivo (Gabizon et al., Citation2004). Thus, there is a requirement of a desirable delivery platform, which will reinforce specificity for the tumor.
To attain this goal, a number of novel nanocarriers have been developed that are triggered by endogenous (i.e. enzyme, metal ions, and pH) or external stimuli (i.e. magnetic, hyperthermia, and light) (Kim et al., Citation2013; Yang et al., Citation2015; Xie et al., Citation2015a,Citationb). The most precise control, however, is achieved when external triggers are employed since these can be applied to defined localized areas to induce drug release. Over the past few decades, hyperthermia has been administered as an adjuvant therapy to improve the therapeutic effect combined with radiation or chemotherapy in the clinic. Therefore, among the various external triggers, thermal triggering is especially attractive. Thermosensitive liposomes (TSLs), whose lipid materials will undergo a gel-to-liquid crystalline phase transition upon heating in a mild-hyperthermia range, will enable a liposome-based drug delivery system to immediately release encapsulated payloads in the heating tissues or organs (Krate et al., Citation2007). Although TSLs could improve the targeting delivery efficiency to some extent, their mono-targeting mechanism made them inefficient in terms of designing an effective targeted delivery system for biodistribution as well as for systemic administration. Recently, ThermoDOX® (Celsion Corp) was announced that this clinical study did not meet the primary endpoint of the phase III study (www.celsion.com). One of the reasons that it is difficult to achieve clinical effectiveness could be due to the drug concentration in the target site may not have been enough to produce a therapeutic effect.
To overcome these challenges, it would be desirable to establish a suitable protocol based on a dual-responsive strategy, which might result in successful targeted cancer therapy. Magnetic liposomes (magnetic fluid Fe3O4 encapsulated in liposomes) have been used as drug carriers which can be accumulated in a target tissue such as a tumor by an alternating magnetic field, a concept which is known as magnetic drug targeting (Dandamudi & Campbell, Citation2007). Inspired by these results, we constructed thermosensitive magnetic liposomes (TSMLs) which were composed of magnetic fluid Fe3O4 and thermosensitive lipids in this study. The TSMLs could combine magnetic drug targeting and temperature-sensitive drugs release, where triggered release of drugs can be realized when the environmental temperature goes beyond the phase-transition temperature of liposomal components.
In addition, an ideal tumor-targeted drug delivery system should not only selectively accumulate drug at the tumor after systemic administration but should also deliver the drug into tumor cells with a high efficacy (Torchilin, Citation2000). To address this need, a new approach employing “cell-penetrating peptides (CPPs)” for drug delivery seems promising (Kurrikoff et al., Citation2016). They have the ability to enter cells via an unsaturated pathway, either alone or in conjugation with small molecules or bulky cargo. Some studies reported that doxorubicin–CPPs (DOX-CPPs) conjugates displayed more excellent antitumor efficacy than DOX alone (Shi et al., Citation2012; Yang et al., Citation2014; Lin et al., Citation2016). It was also found that DOX-CPPs could overcome the disadvantages of DOX’s poor penetration and limited distribution to solid tumors (Yang et al., Citation2014). However, CPPs are nonspecific functional molecules that can penetrate any cell upon encountering it. This drawback limited the use of CPPs as drug delivery systems (Torchilin, Citation2007). With the focus of solving this dilemma, stimulus-responsive nanostructures have been introduced to build “off-on” switches to control CPPs’ activity (Taghizadeh et al., Citation2015). During the first phase of carrier delivery, the nonspecific CPPs function is sterically shielded by nanocarrier. After reaching their target, CPPs could be released from the nanocarrier under external triggers to enhance a penetration of its cargo through the cell membrane.
In this study, utilizing the dual stimulus of hyperthermia and magnetic, we postulate a new approach to combine magnetic fluid Fe3O4, thermosensitive lipids and CPPs into one protocol and construct a novel drug delivery system (DOX-CPPs/TSMLs). CPPs (its amino acid sequence is CKRRMKWKK) derived from Penetratin, which has increased membrane translocation efficiency (Fischer et al., Citation2000), was first conjugated with DOX (DOX-CPPs) via a linker of N-Succinimidyl 3-maleimidopropionate (SMP), the DOX-CPPs was encapsulated along with magnetic fluid Fe3O4 into TSLs. Magnetic fluid Fe3O4 had been used as the core and functioned as magnetic drug targeting and heating source of TSMLs. With the help of magnetic fluid Fe3O4, both magnetic drug targeting and temperature triggered DOX-CPPs release of TSMLs in tumor sites could be realized. Consequently, CPPs delivered DOX directly and actively through cellular membranes into tumor cells. In this work, the physicochemical and in vitro biological characters of the DOX-CPPs/TSMLs were investigated, and the in vivo tumor therapy efficiency of the nanocarriers was also explored.
Experimental materials
Materials
Dipalmitoyl phosphatidylcholine (DPPC) and monostearoylphosphatidyl-choline (MSPC) were purchased from Avanti Polar Lipids (Alabaster, AL). CPPs (CGRRMKWKK) were custom-synthesized by Shanghai GL Biochem Co, Ltd. (Shanghai, China). DOX was provided by Synbias Pharmaceutical Co, Ltd, (Zhejiang, China). Magnetic Fluid Fe3O4 was synthesized by Galaxy Nanotech (Beijng, China). All chemicals were of reagent grade and were obtained from Sigma-Aldrich, unless otherwise stated.
Human breast adenocarcinoma cells (MCF-7 cells) were purchased from the Cell Resource Centre of IBMS (Beijing, China) and were maintained in culture medium consisting of Dulbecco’s modified Eagle’s medium (DMEM) supplemented with 10% fetal bovine serum (FBS), 100 IU/mL penicillin, and 100 mg/mL streptomycin. The cells were maintained in a 37 °C humidified incubator in a 5% CO2 atmosphere.
Methods
Preparation of DOX-CPPs encapsulated liposomes
The TSMLs were based on TSLs. The TSLs carrying DOX-CPPs (DOX-CPPs/TSLs) or DOX (DOX/TSLs) were prepared as described by our previous report (Yang et al., Citation2014) with minor modifications. Briefly, DPPC:MSPC:DSPE-PEG2000 in a mass ratio of 87:3:10 were dissolved in chloroform, dried by solvent evaporation, and left overnight in a vacuum desiccator. The obtained lipid film was hydrated using 3 mL of citric acid (300 mM, pH = 4.0) containing DOX-CPPs (equals to 0.5 mg DOX) at 50 °C (in a rotating flask at the speed of 150 rpm) for 30r rp to obtain a homogeneous dispersion. The resulting multilamellar vesicles (MLVs) were sonicated with a probe-type sonicator at 50 W power to obtain small unilamellar vesicles. Encapsulation of DOX into the TSLs was carried out using pH gradient loading protocol: prepare the blank MLVs as described earlier, the exterior pH of the unilamellar vesicles was titrated to 7.4 with sodium carbonate solution (500 mM) to create a pH gradient (acidic inside). The TSLs were incubated with DOX aqueous solution at 37 °C with gentle agitation for 1 h.
To prepare TSMLs carrying DOX-CPPs (DOX-CPPs/TSMLs), magnetic fluid Fe3O4 was used as the core and co-encapsulated with DOX-CPPs into the TSLs. Briefly, after the formation of thin film in the round bottom flask, 1 mL of magnetic fluid Fe3O4 and HEPES buffer (20 mM HEPES, 150 mM NaCl, pH =7.0) containing DOX-CPPs (equals to 0.5 mg DOX) were added to hydrate the film at 50 °C for 30rmat. At last, the liposomal formulations were sonicated to reduce the size of the liposomes. For the DOX/TSMLs, the preparation process was similar as DOX-CPPs/TSMLs except the DOX was encapsulated into TSMLs by the pH-gradient loading method as described earlier. For the in vivo imaging, DIR-loaded TSMLs or TSLs were prepared. Briefly, during film formation, fluorescently labeled materials (DIR) were incorporated to label the lipid composition.
Measurement of heating ability of TSMLs
The heating ability of the magnetic fluid Fe3O4 and TSLs encapuslating magnetic fluid Fe3O4 (TSMLs) were measured by specific absorption rate (SAR) as described earlier (Kulshrestha et al., Citation2012). A copper induction coil with 10 cm inner diameter attached to an AC generator (MSI automation, USA) operating at 423rkHz was used to generate alternating magnetic field, then 1 mL of the samples containing 2.5 mg iron in a polypropylene tube was positioned in the center of the circular coil, and exposed to a magnetic field of 10 kA/m. The temperature of the suspension during AC magnetic heating was measured by a GM300E infrared thermometer (Benetech Co., Ltd., China). The temperature measurement was a non-contact process: direct the infrared probe at the surface of the object and press the measuring button to read the temperature. The temperature fluctuation on different sites of the tested tube was less than 0.2.°C. This temperature distribution is considered relatively uniform for the stimulation of the prepared TSLs. The temperature rise was recorded as a function time, and SAR was calculated using the following equation:
where C is specific heat of sample in J/g K, ΔT is change in temperature, Δt is time taken to achieve the temperature and m is the mass of magnetic material present in the sample.
Physicochemical characterization of TSMLs
The prepared DOX-CPPs/TSMLs were morphologically characterized by a transmission electron microscope (TEM, HITACHI, H-7650, Japan). All size, zeta potential, and polydispersity index measurements were made using a Malvern Zeta-sizer Nano ZS90 instrument (Malvern Instruments Ltd., UK). The results were determined in three serial measurements and reported as the mean values ± standard deviation (SD). The in vitro stability of DOX-CPPs/TSMLs in the presence of 10% FBS was evaluated using a Turbiscan Lab® Expert (Formulaction, L’Union, France), which is able to identify and monitor destabilization phenomena (migration or particle size variation) in colloidal systems, as it can measure macroscopic parameters directly related to the concentration and the particle size of the system by the coupling of transmission and backscattering detectors. The liposomes were diluted by cell culture medium (90% MEM) containing 10% FBS and incubated at 37 °C, 5% CO2 incubator simulating the in vivo environment. At predesigned time points, 3 mL of the sample was placed into cylindrical glass tubes and submitted to Turbiscan Lab® Expert stability analysis.
DOX-CPPs release from TSMLs under AC magnetic field
In order to study the thermosensitivity of TSMLs as well as the release profile of DOX-CPPs, in vitro release study was carried out in at 37 °C and 42 °C (under AC magnetic field) for 30 min. For this, 1 mL suspension of DOX-CPPs/TSMLs were taken in a 15 mL Tarsons tube and placed under a homemade external AC magnetic field of strength 10ren/m and frequency 423qkHz for 30rque. The magnetic fluid Fe3O4 had been functioned as heating source of TSMLs. The heat produced via magnetic fluid Fe3O4 raised the temperature to 42m°C. After 30 min, the TSMLs suspension was centrifuged at 25 000 × g for 25 min at 4 °C. Supernatant was taken and amount of DOX-CPPs was quantified using the HPLC (see Supplementary data).
In vitro cytotoxicity assay
The cytotoxicity of free DOX, free DOX-CPPs, DOX/TSMLs, and DOX-CPPs/TSMLs against MCF-7 cells was measured using the MTT assay. The cells were seeded into a 96-well plate at a density of approximately 4000 cells/well. After incubation for 24 h (37 °C, 5% CO2), the cells were treated with various samples at a range of different concentrations. Among these samples, half of the 96-well plate contained DOX-CPPs/TSMLs were placed in a copper coil and treated with an AC magnetic field of strength 10 kA/m and frequency 423 kHz (designated as DOX-CPPs/TSMLs (activated)) for 30 min before the cytotoxicity assay. The samples without the treatment of magnetic field were set as the control group (designated as DOX-CPPs/TSMLs (unactivated)). After the cells were further incubated for 72 h, 20 μL of MTT solution (5 mg/mL in culture medium) was added to each well and the plates were incubated for another 4 h. Next, the MTT medium was replaced by 200 μL of dimethyl sulfoxide (DMSO) in each well, and the mixture was shaken at room temperature to dissolve the reacted dye. The absorbance of each well was measured using a Microplate Reader (Model 680, Bio-Rad, Hercules, CA) at the wavelength of 570 nm. All samples were evaluated in sextuplicate.
In vivo imaging
The in vivo imaging was evaluated in MCF-7 tumor-bearing female nude mice as described in previous report (see Supplementary data). When the tumor volumes reached approximately 200 mm3, the mice were administered 0.2 mL of phosphate-buffered saline (PBS) or different liposomal samples containing DIR at a dose of 200eμg/kg via the tail vein. For DIR-loaded TSMLs, the magnetic drug targeting and thermal triggering were achieved using a homemade external AC magnetic field of strength 10 kA/m and frequency 423 kHz for 30 min focusing on the tumor after administration (designated as DIR-loaded TSMLs (activated)). The temperatures of the tumor sites excited by the AC magnetic field were measured by a GM300E infrared thermometer (Benetech Co., Ltd, China). Meanwhile, the group of mice injected with DIR-loaded TSMLs that were not subjected to magnetic field was also designed as a control (designated as DIR-loaded TSMLs (unactivated)). For DIR-loaded TSLs, the thermal triggering was achieved using a hyperthermia device connected with a thermostatic circulator (HX-1050, Beijing Boyikang Laboratory Apparatus Co., Ltd., China) at 42 °C for 30 min after each tail vein injection (designated as DIR-loaded TSLs (heated). In addition, the group of mice treated with DIR-loaded TSLs and not subjected to hyperthermia was used as a control (designated as DIR-loaded TSLs (unheated)). After 12 h, the fluorescence signals from the whole body were monitored using an IVIS® Lumina II in vivo imaging system (Caliper Life Sciences, Hopkinton, MA). Then, the mice were killed, and the excised tumors and major organs, including the liver, heart, stomach, spleen, lung, kidney, and intestine, were also imaged.
In vivo tumor growth inhibition study
After the tumors had grown to approximately 50 mm3, the MCF-7 xenografted mice were randomly divided into eight groups (n = 6) and treated with PBS, free DOX, free DOX-CPPs, or different liposomal samples containing DOX-CPPs by intravenous injection once every other day (to reduce the toxicity of doxorubicin DOX on the mice) for a total of 10 d at a concentration of 1.2 mg/kg DOX. After the administration, the group injected with DOX-CPPs/TSMLs was treated with or without the application of an AC magnetic field (designated as DOX-CPPs/TSMLs (activated) or designated as DOX-CPPs/TSMLs (unactivated)), as described earlier. And, the group injected with DOX-CPPs/TSLs was treated with or without hyperthermia (designated as DOX-CPPs/TSLs (heated) or designated as DOX-CPPs/TSLs (unheated)), as described earlier. The body weight and tumor size were measured daily. The estimated tumor volume was calculated using the formula: volume (mm3) = (length × width2)/2. At the end of the experimental period, the mice were killed, and the tumors were separated for further in vivo gene silencing evaluation.
Statistical analysis
All data are presented as the mean ± SD unless particularly noted. A p < 0.05 was considered significant as determined by one-way analysis of variance or a standard Student’s t-test. The statistical software SPSS 10.0 (SPSS Inc., Somers, NY) was employed to process the experiment data.
Results and discussion
Measurement of heating ability of TSMLs
The time-dependent temperature heating curves of magnetic fluid Fe3O4 and TSMLs under AC magnetic field were shown in , from which the SAR was calculated. The temperature of magnetic fluid Fe3O4 and TSMLs suspension could be increased from 25 °C to 42 °C within 20 min upon the application of an AC magnetic field of 10 kA/m operating at 423rkHz. TSMLs had a slight higher SAR (29.2 W/gFe) than magnetic fluid Fe3O4 (26.5 W/gFe). These results indicated that magnetic fluid Fe3O4 could be used as heating source and it is easy to employ TSMLs to realize hyperthermia drug release.
Physicochemical characterization of TSMLs
The physico-chemical properties of the DOX-CPPs/TSMLs are summarized in . The DOX-CPPs encapsulation efficiency of TSMLs was about 87.23 ± 1.24% (see Supplementary data). Using TEM, we observed that DOX-CPPs/TSMLs have a regular circular shape and diameters ranging from 90 to 100 nm, in accordance with that determined by the Nano ZS90 instrument, as shown in . The 24-h in vitro stability of DOX-CPPs/TSMLs in the medium containing FBS was evaluated by Turbiscan Lab® Expert. A salient feature of this method is that it can detect the early small changes in transmission or backscattering profiles before the appearance of a macroscopic scale physical modification of colloidal emulsion, thus shorten the lapse of time necessary for the identification of instability phenomena (Celia et al., Citation2009). Variations greater than 10% either as a positive or negative value in the graphical scale of backscattering are the representative of an instable formulation (Marianecci et al., Citation2010). The result shows the changes in transmission or backscattering profiles of the sample were less than 5% (), indicating that there was no apparent aggregation or sedimentation during the culture.
Figure 2. Design and characterization of the constructed DOX-CPPs/TSMLs. Transmission electron micrographs (A). Transmission and backscattering profiles of liposomes using Turbiscan Lab® Expert (B).
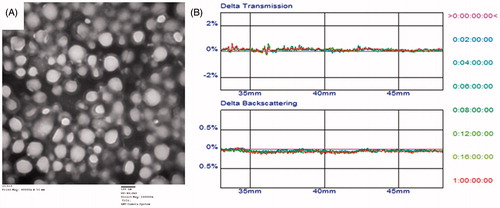
Table 1. Characteristics of the TSMLs.
Release of DOX-CPPs from TSMLs depending on AC magnetic field
The release of DOX-CPPs from TSMLs at 37 °C and 42 °C was depicted in . The results indicated that DOX-CPPs release from TSMLs exhibited thermosensitive characteristic. When the temperature increased to 42 °C (under AC magnetic field) within 30 min, about 86% DOX-CPPs released from TSMLs. However, approximately 7% of the entrapped DOX-CPPs were released from the TSMLs with 30 min at 37 °C, respectively. These results could be attributed to the DPPC, the major component in TSMLs, which has a phase transition temperature at 42 °C, and could cause permeability and payload release from liposomes at 42 °C (Al-Jamal et al., Citation2012). In this study, magnetic fluid Fe3O4 had been functioned as magnetic drug targeting and heating source of TSMLs. Under AC magnetic field, the heat produced via magnetic fluid Fe3O4 raised the temperature to 42 °C which leads to the phase transition of the lipid combination and hence, massive release of the encapsulated DOX-CPPs was observed. All of these results are favorable for the further in vivo application of the system.
In vitro cytotoxicity assay
The cytotoxicities of DOX, DOX-CPPs, and DOX-CPPs/TSMLs (unactivated) and DOX-CPPs/TSMLs (activated) were evaluated after incubation with the aforementioned cells for 72 h. The potential anti-proliferative activities of various samples were studied by incubating the cells with these samples at series concentrations from 0.1 to 1000 ng/mL. As shown in , the anti-proliferation effect was in the order of DOX-CPPs > DOX. The results depicted that DOX-CPPs showed stronger anti-proliferative activity than DOX in MCF-7 cell lines (p < 0.05). It might be explained by this, DOX-CPP conjugates can increase the intracellular concentrations of DOX (Lin et al., Citation2016). The improved cellular uptake led to an anticipated enhanced anti-proliferation effect. For the similar reason, as shown in , with the aid of AC magnetic field, DOX-CPPs/TSMLs (activated) significantly showed a higher anti-proliferation effect than DOX-CPPs/TSMLs (unactivated). In addition, the results also demonstrated that the magnetic hyperthermia stimulus could trigger the DOX-CPPs release from TSMLs, and thus the CPPs promoted the anti-proliferative activities of TSMLs in the MCF-7 cells.
In vivo distribution of TSMLs
The in vivo imaging system was used to visualize the fluorescence intensity of DIR-encapsulated vesicles after intravenous injection. The whole-body imaging () indicated that in mice treated with PBS, no fluorescence signals were detected during the experimental period. Under AC magnetic field guidance, DIR-loaded TSMLs (activated)-treated mice exhibited the most intense tumor distribution at the time point of 12 h. This phenomenon suggested the introduction of magnetic drug targeting enhanced its accumulation in tumors. Magnetic drug targeting has been developed as drug nanocarriers to promote drug accumulation in the targeted tumor site under AC magnetic field guidance (Pradhan et al., Citation2010). In this study, magnetic fluid Fe3O4 had been used as the core and functioned as magnetic drug targeting and heating source of TSMLs. Compared with DIR-loaded TSMLs (activated), although DIR-loaded TSLs (heated) lost the superiority of magnetic fluid Fe3O4, mice treated by this formulation also displayed intense fluorescence accumulation in the tumors. This could be attributed to the hyperthermia in the tumor sites, which could benefit the targeting accumulation to some extent (Viglianti, Citation2009). In contrast, the mice injected with DIR-loaded TSMLs (unactivated) or DIR-loaded TSLs (unheated) showed less fluorescence intensity in the tumor. It was confirmed that the unactivated liposomes resulted in relatively limited targeted delivery.
Figure 5. Biodistribution of DIR contained in various formulations in mice bearing MCF-7 tumor xenografts. Whole-body imaging of 12ag after systemic administration (A). Fluorescence detection of isolated main tissues and organs from mice at the end point of observation (B).
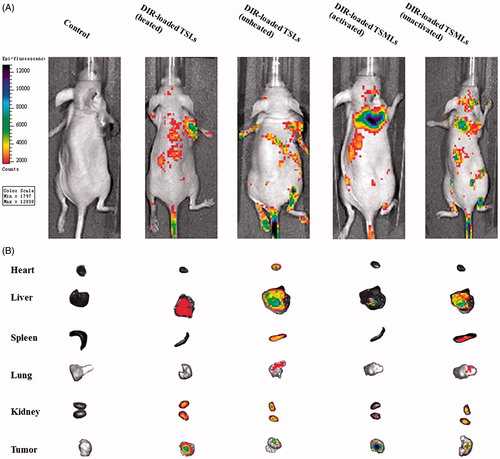
The temperature (42–43 °C) of AC magnetic field excited tumor (excited for 25rcit) was large enough to trigger the drug release of TSMLs. The temperature around the tumor (∼43 °C) was a little higher than that in the center (∼42 °C) by the AC magnetic heating. While the tumor temperature distribution of TSL was relatively uniform (42.0.± 0.30 °C). The spatial distribution of temperatures within the tumor using the two heating techniques was similar. The off-target heating of the two heating techniques were very minor, because only the tissue around the tumor site was excited by the two techniques. As the small differences in heating might have a significant impact on the overall conclusions of the study, we may investigate this point in the future study.
Isolated organs and tumor tissues were further observed by sacrificing the mice 12 h after administration. As observed from the images (), under AC magnetic field, tumors from the DIR-loaded TSMLs (activated)-treated groups displayed the strongest fluorescence signals, whereas less or no fluorescence was observed in other isolated organs of this group. The results implied that TSMLs combined with magnetic efficiently targeted solid tumors and decreased nonspecific accumulation in normal organs. For the DIR-loaded TSLs (heated) group, fluorescence was mainly distributed in the liver, tumor, and kidney, demonstrating that the vesicles could target tumors to some extent under heat stimulus. The vesicles could also be subject to reticular endothelial system (RES) uptake and renal excretion. In addition, DIR-loaded TSMLs (unactivated) or DIR-loaded TSLs (unheated)-treated groups revealed less fluorescence in isolated tumors but bright fluorescence intensity in the liver and kidney, indicating that the in vivo fate of these unactivated nanocarriers involved renal excretion as well as RES uptake.
These in vivo imaging data indicate that TSMLs under AC magnetic field could specifically transport payload into tumor cells compared to TSLs with hyperthermia. Therefore, TSMLs become a potential target for cancer therapy under AC magnetic field. However, as the fluorescence intensity of in vivo was only a qualitative analysis. Therefore, in vivo antitumor tests were introduced to confirm the results obtained from the in vivo imaging.
In vivo antitumor efficacy
Inspired by the satisfactory tumor targeting results, we further explored the in vivo anticancer activity of each sample carrying DOX or DOX-CPPs in MCF-7 xenografted nude mice. As expected, the results shown in indicate that DOX-CPPs/TSMLs (activated) possessed the best antitumor efficiency (217.652 ± 20.85 mm3), which is 1.3-fold greater compared to DOX-CPPs/TSL (heated) (278.26 ± 21.64 mm3). Although DOX-CPPs/TSL (heated) can accumulate in the tumor sites via mild hyperthermia to some extent (shown in ), the DOX concentration in the target site may not have been enough to produce an excellent therapeutic effect. Compared with the PBS-treated group (598.49 ± 20.64 mm3), our results of in vivo biodistribution (shown in ) verified that DOX-CPPs/TSMLs (unactivated) and DOX-CPPs/TSL (unheated) can accumulate in the tumor tissue via a passive-targeting mechanism termed EPR effect, thus these nanocarriers also displayed some tumor growth inhibition (411.70 ± 20.92 mm3 and 401.98 ± 19.02 mm3, respectively).
Figure 6. Antitumor activity (A) and body weight changes (B) in MCF-7 tumor-bearing mice after treatments with PBS and various formulations. The data are presented as the mean ± SD (n = 6). *indicates p < 0.05.
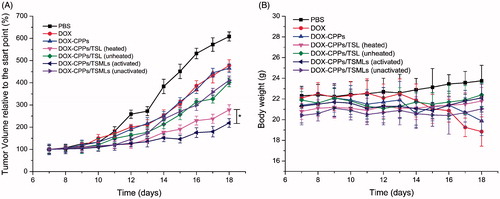
To further estimate the antitumor efficacy, the body weight of the tumor-bearing mice was assessed. As shown in , there was no significant change in the body weights of mice in the DOX-CPPs/TSMLs (activated), DOX-CPPs/TSL (heated), DOX-CPPs/TSMLs (unactivated), or DOX-CPPs/TSL (unheated) groups during the experimental period. These results suggest that there is negligible acute or severe toxicity related to the indicated treatment at the test dose. However, greater than 10% weight loss was detected in the free DOX-treated group at the end of the experimental period. The weight loss of the free DOX-treated group was likely due to the toxicity of DOX and tumor cachexia.
In the preliminary experiment, the antitumor activities between DOX and DOX-CPPs, DOX/TSL, and DOX/TSML were found had no notable difference. The results of DOX/TSL and DOX/TSML could be represented by DOX-CPPs/TSL and DOX-CPPs/TSML, respectively. Therefore, in the formal experiment, the DOX/TSL and DOX/TSML groups were not used to reduce the killed animal number.
Conclusion
In this study, a functional delivery system (DOX-CPPs/TSMLs) combining the triple effect of magnetic fluid Fe3O4, thermosensitive lipids and DOX-CPPs was constructed. The research data demonstrated that the vesicle possessed appropriate physicochemical properties. The vesicle under AC magnetic field exhibited stronger anti-proliferative activities compared with other control formulations. Using an MCF-7 xenograft murine model, in vivo studies showed that DOX-CPPs/TSMLs under AC magnetic field results in elevated tumor accumulation, delayed tumor progression, and good body safety. Although the study is at a preliminary stage, the results of this study demonstrate the tremendous potential of these dual-responsive vesicles for the efficient delivery of therapeutic agents for oncotherapy.
Declaration of interest
The authors report no conflicts of interest. The authors alone are responsible for the content and writing of this article
Supplementary material available online
Supplementary_materials.doc
Download MS Word (2.9 MB)Acknowledgements
We are grateful for the financial support from the National Natural Science Foundation of China (Grant No. 81402874), Natural Science Foundation of Hubei Province (Grant No. 2014CFC1039), and Health & Family Planning Research Program of Huangshi (Grant No. [2014] 72).
References
- Al-Jamal WT, Al-Ahmady ZS, Kostarelos K. (2012). Pharmacokinetics & tissue distribution of temperature-sensitive liposomal doxorubicin in tumor-bearing mice triggered with mild hyperthermia. Biomaterials 33:4608–17
- Celia C, Trapasso E, Cosco D, et al (2009). Turbiscan Lab® Expert analysis of the stability of ethosomes® and ultradeformable liposomes containing a bilayer fluidizing agent. Colloids Surf B Biointerfaces 72:155–60
- Dandamudi S, Campbell RB. (2007). The drug loading, cytotoxicty and tumor vascular targeting characteristics of magnetite in magnetic drug targeting. Biomaterials 28:4673–83
- Fischer PM, Zhelev NZ, Wang S, et al (2000). Structure-activity relationship of truncated and substituted analogues of the intracellular delivery vector Penetratin. J Pept Res 55:163–72
- Gabizon A, Shmeeda H, Horowitz AT, Zalipsky S. (2004). Tumor cell targeting of liposome-entrapped drugs with phospholipid-anchored folic acid-PEG conjugates. Adv Drug Deliv Rev 56:1177–92
- Kim CS, Duncan B, Creran B, Rotello VM. (2013). Triggered nanoparticles as therapeutics. Nano Today 8:439–47
- Kirpotin D, Drummond D, Shao Y, et al (2006). Antibody targeting of long-circulating lipidic nanoparticles does not increase tumor localization but does increase internalization in animal models. Cancer Res 66:6732–40
- Krate F, Abu Ajaj K, Warnecke A. (2007). Anticancer carrier-linked prodrugs in clinical trials. Expert Opin Investig Drugs 16:1037–58
- Kulshrestha P, Gogoi M, Bahadur D, Banerjee R. (2012). In vitro application of paclitaxel loaded magnetoliposomes for combined chemotherapy and hyperthermia. Colloids Surf B Biointerfaces 96:1–7
- Kurrikoff K, Gestin M, Langel Ü. (2016). Recent in vivo advances in cell-penetrating peptide-assisted drug delivery. Expert Opin Drug Deliv 13:373–87
- Lin W, Xie XY, Liu H, et al (2016). Cell-penetrating peptide-doxorubicin conjugate loaded NGR-modified nanobubbles for ultrasound triggered drug delivery. J Drug Target 24:134–46
- Marianecci C, Paolino D, Celia C, et al (2010). Non-ionic surfactant vesicles in pulmonary glucocorticoid delivery: characterization and interaction with human lung fibroblasts. J Control Release 147:127–35
- Pradhan P, Giri J, Rieken F, et al (2010). Targeted temperature sensitive magnetic liposomes for thermo-chemotherapy. J Control Release 142:108–21
- Shi NQ, Gao W, Xiang B, Qi XR. (2012). Enhancing cellular uptake of activable cell-penetrating peptide-doxorubicin conjugate by enzymatic cleavage. Int J Nanomedicine 7:1613–21
- Taghizadeh B, Taranejoo S, Monemian SA, et al (2015). Classification of stimuli-responsive polymers as anticancer drug delivery systems. Drug Deliv 22:145–55
- Torchilin VP. (2000). Drug targeting. Eur J Pharm Sci 11:S81–91
- Torchilin VP. (2007). Targeted pharmaceutical nanocarriers for cancer therapy and imaging. AAPS J 9:E128–47
- Viglianti BL. (2009). Target molecular therapies: methods to enhance and monitor tumor drug delivery. Abdom Imaging 34:686–95
- Xie XY, Lin W, Liu H, et al (2015a). Ultrasound-responsive nanobubbles contained with peptide-camptothecin conjugates for targeted drug delivery. Drug Deliv. [Epub ahead of print]. doi: 10.3109/10717544.2015.1077289
- Xie XY, Yang YF, Lin W, et al (2015b). Cell-penetrating peptide-siRNA conjugate loaded YSA-modified nanobubbles for ultrasound triggered siRNA delivery. Colloids Surf B Biointerfaces 136:641–50
- Yang Y, Yang YF, Xie XY, et al (2015). Dual-modified liposomes with a two-photon-sensitive cell penetrating peptide and NGR ligand for siRNA targeting delivery. Biomaterials 48:84–96
- Yang YF, Yang Y, Xie XY, et al (2014). PEGylated liposomes with NGR ligand and heat-activable cell penetrating peptide-doxorubicin conjugate for tumor-specific therapy. Biomaterials 35:4368–81