Abstract
Context: Transdermal local anesthesia is one of the most applied strategies to avoid systemic adverse effects; there is an appealing need for a prolonged local anesthetic that would provide better bioavailability and longer pain relief with a single administration.
Objective: Layer-by-layer (LBL) technique was used in this study to explore a nanosized drug delivery system for local anesthetic therapy.
Materials and methods: LBL-coated lidocaine-loaded nanostructured lipid nanoparticles (LBL-LA/NLCs) were prepared and characterized in terms of particle size (PS), zeta potential, drug encapsulation efficiency (EE), in vitro skin permeation and in vivo local anesthetic studies.
Results: Evaluation of the in vitro skin permeation and in vivo anesthesia effect illustrated that LBL-LA/NLCs can enhance and prolong the anesthetic effect of LA.
Discussion and conclusion: LBL-LA/NLCs could function as a promising drug delivery strategy for overcoming the barrier function of the skin and could deliver anesthetic through the skin with sustained release behavior for local anesthetic therapy.
Introduction
Transdermal local anesthesia is one of the most applied strategies to avoid systemic adverse effects; as well as minimize anxiety, pain and discomfort at the site of needle insertion prior to anesthesia during dermatologic surgical procedures (Musawi & Andersson, Citation2010; de Araújo et al., Citation2013; Pino et al., Citation2014). Some of the challenges for transdermal local anesthesia include low permeability of active ingredients, due to skin barrier such as the stratum corneum (Alexander et al., Citation2012). Moreover, the rapid redistribution of local anesthetics from the site of administration limits their duration of action. Therefore, there is an appealing need for a prolonged local anesthetic that would provide better bioavailability and longer pain relief with a single administration (Yuan & Acosta, Citation2009; Cohen et al., Citation2012).
Most commercially available topical anesthetic products use either lidocaine (LA) or prilocaine in lipid-soluble free-base form. It has been known that when these lipophilic drugs are encapsulated in lipid-based nanoparticles such as liposomes, solid lipid nanoparticles (SLNs), and nanostructured lipid nanoparticles (NLCs), their permeation through the skin is enhanced (Pathak & Nagarsenker, Citation2009; Shim et al., Citation2010; Puglia et al., Citation2011; de Araújo et al., Citation2013). NLCs are formed from a combination of solid and liquid lipids, which is still solid at body temperature (Müller et al., Citation2011). It is the second-generation lipid nanoparticles, with the following advantages: higher drug loading and storage stability; biocompatible, biodegradable and safe lipid composition; and controlled release. Moreover, the lipid nanocarrier adheres to the skin leads to the formation of thin film, and subsequently, to an occlusive effect with a decrease in the transepidermal water loss (Pardeike et al., Citation2009). Recently, Puglia and coauthors evaluated the encapsulation of benzocaine and LA in NLCs dispersions (Puglia et al., Citation2011). The NLCs dispersions comprised NLCs nanoparticles and hydrogels, which contained glycerol and xanthan gum. In vitro and in vivo tests of the NLCs hydrogel have indicated that the prolonged duration of action placing the NLCs hydrogel as a promising carrier for dermal delivery of LA (Puglia et al., Citation2011). Hydrogel formulations (chitosan, xanthan gum, hydroxyethylcellulose-4000, etc) containing NLCs were investigated regarding the physical stability of the lipid nanoparticles; results suggested that a good physical stability was obtained (Pardeike et al., Citation2009). Combined with the NLCs technology and hydrogel technology, we ulteriorly designed hyaluronic acid-coated and chitosan-coated NLCs using the layer-by-layer (LBL) technique.
LBL technique, first introduced at the beginning of the 1990s by Gero Decher, is a simple and versatile method used to assemble multilayer films with alternating the deposition of oppositely charged polyelectrolytes via electrostatic attraction (Decher, Citation1997). Coating of nanoparticles by LBL technology is an active area of research and currently considered a hot topic with many potential applications in drug delivery by the injection route and transdermal administration (Labouta & Schneider, Citation2010; Ramasamy et al., Citation2014; Jeon et al., Citation2015). Xavier et al. have proven that the slow release rate of aloin was achieved when it was encapsulated into liposomes in LBL films for transdermal drug delivery (Xavier et al., Citation2013). Jeon et al. have improved stability and skin permeability of sodium hyaluronate-chitosan multilayered liposomes by LBL electrostatic deposition for quercetin delivery (Jeon et al., Citation2015). However, there are no researches combing NLCs technology and LBL technique for slowing the release rate and improving skin permeability of local anesthetics.
Hyaluronic acid (HA), a negatively charged glycosaminoglycan, has versatile properties, including biocompatible, biodegradable, water absorption and water retention. It could be used in transdermal delivery (Choi et al., Citation2012; Jeon et al., Citation2015). Chitosan (CS), a cationic polysaccharide, is noncytotoxic, biocompatible, viscoelastic and degraded slowly over time into bioresorbable products, making them promising local anesthetic delivery vehicles (Yang et al., Citation2012). Researches have demonstrated that CS were able to improve drug diffusion to deeper skin due to its positively charged layers interacting with negative charges in the skin (Sapra et al., Citation2008; Li et al., Citation2011; El-Badry et al., Citation2014; Eroğlu et al., Citation2016). A HA–CS complex assembled using electrostatic attractions based on LBL liposomes has been investigated in transdermal delivery with the HA as the outermost layer, which showed negative charge (Jeon et al., Citation2015). In this study, we first designed CS and HA-coated NLCs for local anesthetic LA delivery system, in which CS was the outermost layer.
In the present study, we used the LBL technique to form the stable core-shell nanoparticles, thus allowing us to combine the advantages of NLCs with those of polyelectrolyte multilayer. To explore the suitability of the LBL-coated LA-loaded NLCs (LBL-LA/NLCs) delivery system, physicochemical parameters, in vitro release study, in vitro skin permeation and in vivo evaluation of local anesthetic effects were performed on animal models.
Materials and methods
Materials
LA, CS, glyceryl monostearate (GM) and cetyltrimethyl ammonium bromide (CTAB) were purchased from Sigma Aldrich (St. Louis, MO). Miglyol 812N was purchased from Peter Cremer GmbH (Hamburg, Germany). HA was provided by Shandong Freda Biochem Co., Ltd. (Shangdong, China). All other reagents and solvents were analytical or high-performance liquid chromatography (HPLC) grade.
Animals
Sprague-Dawley rats (SD rats) weighing 350–400 g (10–12 weeks old) were purchased from the Medical Animal Test Center of Shandong University. The rats were maintained under controlled conditions (temperature, 20 ± 1 °C; humidity, 60 ± 5%; 12/12 h light/dark cycle). All animal experiments complied with the Animal Management Rules of the Ministry of Health of the People’s Republic of China.
Fabrication and optimization of LBL-LA/NLCs
LA-loaded NLCs (LA/NLCs) were prepared by the melt-emulsification method (Puglia et al., Citation2011) (). For the screening of solid lipid, GM, stearic acid, compritol 888 ATO and Precirol ATO5 were used. Among all the lipids used, GM showed the highest drug portioning; hence, it was chosen for the formulation of NLCs. For the selection of liquid lipid, Miglyol 812N, polyoxyl castor oil, soybean oil and oleic acid was applied. Miglyol 812N showed the most uniform size distribution, so it was determined as oil phase. CTAB, dimethyl dioctadecylammonium bromide (DDAB), 1,2-dioleoyl-3-trimethylammoniumpropane (DOTAP), and N-[1-(2,3-Dioleyloxy)propyl]-N,N,N-trimethylammonium chloride (DOTMA) was used as surfactant to dissolve in the aqueous phase, among which CTAB achieved the best efficiency.
Briefly, 0.3 g GM as solid lipid was melted at 70 °C, and 0.2 g Miglyol 812N as oil phase and LA were added in a water bath at 70 °C. The desired amount of CTAB was dissolved in distilled water as aqueous phase, and heated to the same temperature. Then, the melted lipid phase was added into the aqueous phase drop wise under mechanical stirring at 500 rpm in a water bath at 70 °C for 20 min to form microemulsion. Finally, NLCs dispersions were obtained by solidifying under stirring at 500 rpm in an ice bath for 1 h. Blank NLCs without LA (NLCs) were prepared by the same method without adding LA.
To prepare the LBL-LA/NLCs, we coated cationic-charged LA/NLCs with an anionic HA solution at different volume ratios (Ramasamy et al., Citation2014). Then, the mixture was incubated for 30 min with stirring at 500 rpm. The subsequent layer was fabricated by adding CS solutions to the HA-coated LA/NLCs (HA-LA/NLCs) using the same process (). LBL-coated blank NLCs without LA (LBL-NLCs) were prepared by the same method using NLCs instead of LA/NLCs.
Box–Behnken design for the optimization of formulation
The Box–Behnken design is one of the response surface models used to hit the target, maximize or minimize a response. Based on the preliminary formulation screening, we used Box–Behnken experimental design for optimization of formulation with smaller particle size (PS) and a narrow particle size distribution (PDI). A 4-factor, 3-level Box–Behnken design was used for exploring quadratic response surfaces and constructing second-order polynomial models with Design Expert software V.8.0.5b (Stat-Ease Inc., Minneapolis, MN) (). A design matrix comprising 29 experimental runs was constructed. It was used to elucidate the main effects and interactions of lipid concentration (X1), surfactant concentration (X2), volume of HA solution (X3) and volume of CS solution (X4). Particle size (Y1) and polydispersity index (PDI) (Y2) were used as the indicators to determine the optimum formulation. Details of the design are listed in .
Table 1. Relationship between coded and actual values of the variables in Box–Behnken design for LBL-LA/NLCs formulation.
Characterization of the NLCs
Particle size, size distribution and zeta potential
The particle size, PDI and zeta potential of the NLCs were measured by dynamic laser scattering (DLS, Malvern Zetasizer 3000, Malvern Instruments, Worceshtire, UK). The dispersion of NLCs was diluted by ultrapure water according to the mass concentration, and were completely sonicated before measurement. The zeta potentials of the NLCs were measured based on electrophoretic mobility under an electric field (Jeon et al., Citation2015).
Surface morphology
The surface morphology of the LBL-LA/NLCs and LA/NLCs were observed by transmission electron microscopy (TEM, JEM-1200EX; JEOL Ltd, Tokyo, Japan). Samples were prepared respectively by placing a drop of NLCs suspensions onto a copper grid and air drying, followed by negative staining with one drop of 3% aqueous solution of sodium phosphotungstate for contrast enhancement. The air-dried samples were then directly examined under the transmission electron microscope (Li et al., Citation2012).
Drug encapsulation and loading efficiency
The drug encapsulation efficiency (EE) and drug loading efficiency (DL) of the LBL-LA/NLCs, HA-LA/NLCs, and LA/NLCs was measured by gel filtration method using Sephadex G50 Column (GE Healthcare Bio-Sciences, Pittsburgh, PA) (Vitorino et al., Citation2013). The free LA was separated from the NLCs and the content of free drug was measured using high-performance liquid chromatography (HPLC), performed on an Agilent 1100 series with a zorbax RP-select B column (Agilent Technologies, Santa Clara, CA) and the mobile phase was a mixture of 0.01 M sodium dihydrogen phosphate and methanol (25:75, v/v). The flow rate was 1 mL/min. The EE and DL were calculated using the following equations:
(1)
(2)
where the Wtotal LA is the total amount of LA determined in the NLCs system, the Wfree LA is the amount of free LA determined in the aqueous phase after separation of the NLCs and Wlipid is the weight of the lipid phase.
In vitro skin permeation experiments
In vitro skin permeation studies were performed using the Franz diffusion cells (Pathak & Nagarsenker, Citation2009; Puglia et al., Citation2011). SD rats were sacrificed by dislocation. The fur on the abdominal area of the rats was carefully removed by an electrical shaver to avoid damage to stratum corneum. The skin from the abdominal surface was excised and the adherent fat and subcutaneous tissue were removed. The in vitro skin permeation studies of LA from different formulations was carried out using the Franz glass diffusion cell maintained at 37 °C under nonocclusive conditions. The skin permeation study was performed using six diffusion cells and represented as an average of six cells. The skin samples were mounted on the Franz diffusion cell with a surface area of 0.75 cm2 and a receptor volume of 4.5 mL. Accurately weighed LA-containing formulations corresponding to 5 mg of LA was applied on the donor compartment side of the skin. The donor compartment was covered with an aluminum foil to avoid evaporation. The temperature was maintained at 37 ± 0.5 °C. Aliquots of 0.5 mL receptor medium was withdrawn at time intervals of 0, 0.5, 1, 2, 4, 6, 8, 10, 12, 15, 18 and 24 h and was replaced by the same volume of fresh buffer to maintain the sink condition. The samples were quantified by using HPLC analysis as described in the “Drug encapsulation and loading efficiency” section.
The flux of drug from each formulation was calculated (Junyaprasert et al., Citation2008). The cumulative drug permeation (Qt) was calculated as follows:
(3)
where Ct is the drug concentration of the receptor fluid at each sampling time; Ci is the drug concentration of the ith sample; Vr and Vs are the volumes of the receptor fluid.
Data were expressed as the cumulative drug permeation per unit of skin surface area, Qt/S (S = 0.75 cm2). The steady-state fluxes (JSS) were calculated by linear regression interpolation of the experimental data at steady state (△T) as shown in the following equation:
(4)
In vivo evaluation of local anesthetic effects
Local anesthetic effects of LA-containing formulations were evaluated by tail-flick latency (TFL) test using a tail-flick measuring device (Ugo Basile, Varese, italy) (Puglia et al., Citation2011; Ouchi et al., Citation2013; Caffarel-Salvador et al., Citation2015). A noxious heat stimulus was applied via a focused, radiant heat light source to the dorsal surface of the tail. Tail-flick test started 5 min after the local application of different preparations, and the test was conducted every 5 min for the first 30 min and then every 15 min for a total of 75 min. TFL was converted to represent the maximum possible effect (MPE) according to the following equation:
(5)
The baseline TFL was calculated as the mean of three different measurements taken at 10-min intervals. Baseline latencies were typically ranged from 2.5 to 3.0 s. A maximum cutoff latency of 10 s was set to avoid tissue damage in analgesic animals. Results are expressed as mean ± standard of eight rats per group.
Statistical analysis
All studies were repeated at least three times. Results were reported as means ± standard deviation. Statistical significance was tested by two-tailed Student’s t-test. Differences between experimental groups were considered significant when the p value was less than 0.05 (p < 0.05).
Results
Optimization of LBL-LA/NLCs formulation
A 4-factor, 3-level Box–Behnken design was applied to precisely understand the effect of amount of lipid (X1), amount of surfactant (X2), volume of HA solution (X3) and volume of CS solution (X4) on PS and PDI of LBL-LA/NLCs. The respective observed responses are given in .
Table 2. The Box–Behnken experiment design of LBL-LA/NLCs and evaluated response parameters (n = 3).
Quadratic equations establishing main effects and interaction factors were determined. Statistical validation of quadratic equations was confirmed by ANOVA. The Model F value of PS and PDI were 1854.55 and 2564.7, respectively, which indicate the model was significant.
Response surface analyses plotted in three-dimensional model graphs for depicting the effects of the predetermined factors on the PS or PDI are shown in .
Figure 2. Three-dimensional model graphs for depicting the effects of the predetermined factors on the PS or PDI.
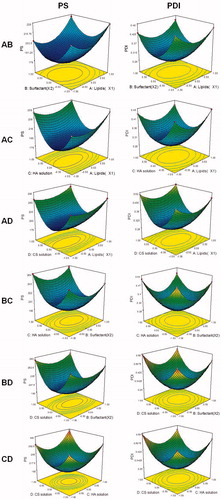
The optimum formulation was based on the set criteria of smaller PS, and minimum PDI. The composition of optimized formulation was as follows: lipid was 29.7%, the amount of surfactant was 2.06%, the volume of HA solution was 40.2 μL and the volume of CS solution was 39.6 μL. A new batch of NLCs with the predicted levels was prepared. PS and PDI of optimized formulation were 181.2 and 0.264, respectively, which were in good agreement with the predicted values.
Surface morphology
shows the TEM images of the structural morphology of the LBL-LA/NLCs and LA/NLCs. The images of the LA/NLCs revealed nanosized, spherical shape without hierarchy (). The image of LBL-LA/NLCs had obviously two layers on the surface (): a darker sub-coat was one layer, which was further coated by a slightly gray coat on the surface of the particle. According to the 100 nm bar in the images, the LBL-LA/NLCs were larger in size compared to the uncoated LA/NLCs.
Zeta potential, EE and DL
The zeta potentials of LBL-LA/NLCs and other formulations are summarized in . The zeta potential of blank NLCs was +33.5 mV, with the loading of negatively charged LA, the zeta potential of LA/NLCs decreased to +13.9 mV. HA is a negatively charged glycosaminoglycan, after coated with HA, the surface charge of LA/NLCs was reversed, HA-LA/NLCs has a zeta potential of −21.5 mV. CS is a cationic polysaccharide, the coating of the outer layer of CS increased the zeta potential of LBL-LA/NLCs to +37.6 mV. EE of all LA-loaded NLCs was over 85%. DL of LA/NLCs, HA-LA/NLCs and LBL-LA/NLCs was 11.6%, 5.7% and 2.1%, respectively.
Table 3. Characterization of different NLCs formulations.
In vitro skin permeation
In vitro permeation profiles of LA from different formulations were calculated and are shown in . summarizes the steady-state fluxes (JSS) with different formulations. Data show that the permeation of LA in LA/NLCs was much more sufficient than LA solutions (p < 0.05). The results illustrated that NLCs formulas have better skin permeation capacity. The permeation of LBL-LA/NLCs was more sufficient than HA-LA/NLCs (p < 0.05); and permeation of HA-LA/NLCs was more sufficient than LA/NLCs (p < 0.05). The results indicated that LBL-LA/NLCs have the potential to carry LA across the skin layers more freely than other systems.
Table 4. Skin permeation steady-state fluxes (JSS) of the LA from different formulations.
In vivo local anesthetic effects
Local anesthetic effects of LA-containing formulations were evaluated by TFL test. As reported in , all the LA-containing formulations increased the TFL significantly. There were no significant differences in baseline TFL between the saline control group and other formulations. The time MPE in the saline group did not change during the experiment. LA solution formulation increased t showed a rapid but short-lasting anesthetic effect, with a peak effect seen within 10 min from the application. While the LA-loaded NLCs formulations have longer effect than the solution formulation, among which LBL-LA/NLCs were able to induce a pronounced anesthetic effect that persisted for 60 min after the application. We can tell from the results that the anesthetic activity of LBL-LA/NLCs revealed a more interesting rapid anesthetic effect in the first few minutes and also displayed sustained activity compared with the other formulations.
Discussion
The present study aimed to use the LBL technique to form the stable core-shell nanoparticles, thus allowing us to combine the advantages of NLCs with those of polyelectrolyte multilayer (Mandapalli et al., Citation2014). LBL-LA/NLCs delivery system was designed to overcome the barrier function of the skin and could deliver anesthetic through the skin with sustained release behavior for local anesthetic therapy.
At the beginning of this study, the LBL-LA/NLCs formulation was optimized by Box–Behnken design. A 4-factor, 3-level Box–Behnken design was applied to precisely understand the effect of amount of lipid (X1), amount of surfactant (X2), volume of HA solution (X3) and volume of CS solution (X4) on PS and PDI of LBL-LA/NLCs. The respective observed responses are given in . Quadratic equations establishing main effects and interaction factors were determined. Statistical validation of quadratic equations was confirmed by ANOVA. The Model F value of PS and PDI were 1854.55 and 2564.7, respectively, which indicate the model was significant. Values of “Prob > F” less than 0.05 demonstrated model terms such as X1, X2, X3, X4, X1X2, X1X4, X3X4, etc were significant model terms. Nonsignificant lack of fit for PS and PDI imply that models were well fitted. The effect could be explained by the following quadratic equations:
Response surface analyses plotted in three-dimensional model graphs for depicting the effects of the predetermined factors on the PS or PDI are shown in . The optimum formulation was based on the set criteria of smaller PS, and minimum PDI. The composition of optimized formulation was as follows: lipid was 29.7%, the amount of surfactant was 2.06%, the volume of HA solution was 40.2 μL and the volume of CS solution was 39.6 μL. A new batch of NLCs with the predicted levels was prepared. PS and PDI of optimized formulation were 181.2, and 0.264, respectively, which were in good agreement with the predicted values. PS and PDI are important factors for the nanocarriers, which can influence the biodistribution of carriers (Zhang et al., Citation2016). PS lower than 200 nm could decrease uptake by the liver, prolongs circulation time in the blood and improves bioavailability. The small PS of the delivery system may enhance the bioavailability of bioactive compounds, adhesion efficiency with biological cells and cellular uptake ability of the drug delivery system (Chinaeke et al., Citation2015). Decreasing the PS results in an occlusive effect on the skin layer and could lead to a long duration of action of the drug (Pathak & Nagarsenker, Citation2009).
Surface morphology of LBL-LA/NLCs and LA/NLCs was determined by the TEM images (). The images of the LA/NLCs revealed nanosized, spherical shape without hierarchy. The image of LBL-LA/NLCs had obviously two layers on the surface: a darker sub-coat was one layer, which was further coated by a slightly gray coat on the surface of the particle. According to the 100 nm bar in the images, the LBL-LA/NLCs were larger in size compared to the uncoated LA/NLCs. Surface charge is an important indication for the structure and stability of nanoparticle system in medium (Wang et al., Citation2013). High surface charge may lead to stronger repellent interactions among nanoparticles of dispersion, resulting in an enhanced stability. The zeta potential of blank NLCs was +33.5 mV, with the loading of negatively charged LA, the zeta potential of LA/NLCs decreased to +13.9 mV (). HA is a negatively charged glycosaminoglycan, after coated with HA, the surface charge of LA/NLCs was reversed, HA-LA/NLCs has a zeta potential of −21.5 mV. CS is a cationic polysaccharide: biodegradability and the absence of toxicity increase the use of CS and derivatives in the pharmaceutical technology (Pignatello et al., Citation2009). Moreover, it can form hydrogels and films, is hydrophilic, and can be sterilized at high temperatures. The coating of the outer layer of CS increased the zeta potential of LBL-LA/NLCs to +37.6 mV. Cationic surface charge may augment in absorption across the skin barrier which could enhance the potential of nano-sized delivery system (Yang et al., Citation2015). The positively charged surface of LBL-LA/NLCs could make the carriers easily bind toward the negatively charged cell surfaces. The EE is a key effect of the drug therapeutic effect (Ghanbarzadeh et al., Citation2015). EE of all LA-loaded NLCs was over 85%. DL of LA/NLCs, HA-LA/NLCs and LBL-LA/NLCs was 11.6%, 5.7% and 2.1%, respectively.
In vitro permeation profiles of LA from different formulations were calculated (), and the JSS with different formulations were summarized (). Data show that the permeation of LA in LA/NLCs was much more sufficient than LA solutions (p < 0.05). The results illustrated that NLCs formulas have better skin permeation capacity, which may be due to their similar property to skin lipids, the high specific surface area for drug absorption because of their smaller size, the existence of a solid matrix and the biocompatibility make them suitable for skin administration (Vitorino et al., Citation2013; Alam et al., Citation2014). The permeation of LBL-LA/NLCs was more sufficient than HA-LA/NLCs (p < 0.05); and permeation of HA-LA/NLCs was more sufficient than LA/NLCs (p < 0.05). HA, which is a biocompatible and biodegradable polymer that dissolves rapidly in the biological milieu, could be used in transdermal delivery (Choi et al., Citation2012; Jeon et al., Citation2015). CS gels are noncytotoxic, biocompatible, viscoelasticity and degrade slowly over time into bioresorbable products, making them promising local anesthetic delivery vehicles (Yang et al., Citation2012). Thus, HA and CS could be assembled over the out layer of NLC by the LBL technology in this study and the results indicated that LBL-LA/NLCs has the potential to carry LA across the skin layers more freely than other systems.
The TFL test is the most frequently used method to measure pain levels and assess the effects of anesthesia (Ouchi et al., Citation2013). It uses radiant heat to both measure the latency of the response to thermal noxious stimuli and assess the pain threshold and effect of anesthesia. Radiant heat is a type of constant infringement stimulation that does not damage the skin when a proper cutoff time is used (Jackson et al., Citation2010). Local anesthetic effects of LA-containing formulations were evaluated by TFL test. As reported in , all the LA-containing formulations increased the TFL significantly. There were no significant differences in baseline TFL between the saline control group and other formulations. The time MPE in the saline group did not change during the experiment. LA solution formulation increased t showed a rapid but short-lasting anesthetic effect, with a peak effect seen within 10 min from the application. While the LA-loaded NLCs formulations have longer effect than the solution formulation, among which LBL-LA/NLCs were able to induce a pronounced anesthetic effect that persisted for 60 min after the application. We can tell from the results that the anesthetic activity of LBL-LA/NLCs revealed a more interesting rapid anesthetic effect in the first few minutes and also displayed sustained activity compared with the other formulations. The sustained anesthetic effect of the LBL lipid carriers could bring about the long-lasting local anesthetic therapy.
Conclusions
LBL technique was used in this study to form the LA-loaded core-shell NLCs. LBL-LA/NLCs were applied for the transporting of LA through the skin to achieve topical anesthesia. In vitro skin permeation studies and in vivo anesthesia effect evaluation illustrated LBL-LA/NLCs can enhance and prolong the anesthetic effect of LA. LBL-LA/NLCs could function as a promising drug delivery strategy for overcoming the barrier function of the skin and could deliver anesthetic through the skin with sustained release behavior.
Declaration of interest
The authors report no conflicts of interest.
References
- Alam S, Aslam M, Khan A, et al (2014). Nanostructured lipid carriers of pioglitazone for transdermal application: from experimental design to bioactivity detail. Drug Deliv 23:601–9
- Alexander A, Dwivedi S. Ajazuddin, et al (2012). Approaches for breaking the barriers of drug permeation through transdermal drug delivery. J Control Release 164:26–40
- Caffarel-Salvador E, Tuan-Mahmood TM, McElnay JC, et al (2015). Potential of hydrogel-forming and dissolving microneedles for use in paediatric populations. Int J Pharm 489:158–69
- Chinaeke EE, Chime SA, Onyishi VI, et al (2015). Formulation development and evaluation of the anti-malaria properties of sustained release artesunate-loaded solid lipid microparticles based on phytolipids. Drug Deliv 22:652–65
- Choi WI, Lee JH, Kim JY, et al (2012). Efficient skin permeation of soluble proteins via flexible and functional nano-carrier. J Control Release 157:272–8
- Cohen R, Kanaan H, Grant GJ, et al (2012). Prolonged analgesia from bupisome and bupigel formulations: from design and fabrication to improved stability. J Control Release 160:346–52
- de Araújo DR, da Silva DC, Barbosa RM, et al (2013). Strategies for delivering local anesthetics to the skin: focus on liposomes, solid lipid nanoparticles, hydrogels and patches. Expert Opin Drug Deliv 10:1551–63
- Decher G. (1997). Fuzzy nanoassemblies: toward layered polymeric multicomposites. Science 277:1232–7
- El-Badry M, Fetih G, Shakeel F. (2014). Comparative topical delivery of antifungal drug croconazole using liposome and micro-emulsion-based gel formulations. Drug Deliv 21:34–43
- Eroğlu İ, Azizoğlu E, Özyazıcı M, et al (2016). Effective topical delivery systems for corticosteroids: dermatological and histological evaluations. Drug Deliv 23:1502–13
- Ghanbarzadeh S, Khorrami A, Arami S. (2015). Nonionic surfactant-based vesicular system for transdermal drug delivery. Drug Deliv 22:1071–7
- Jackson KJ, Carroll FI, Negus SS, et al (2010). Effect of the selective kappa-opioid receptor antagonist JDTic on nicotine antinociception, reward, and withdrawal in the mouse. Psychopharmacology (Berl) 210:285–94
- Jeon S, Yoo CY, Park SN, et al (2015). Improved stability and skin permeability of sodium hyaluronate-chitosan multilayered liposomes by layer-by-layer electrostatic deposition for quercetin delivery. Colloids Surf B Biointerfaces 129:7–14
- Junyaprasert VB, Boonme P, Wurster DE, et al (2008). Aerosol OT microemulsions as carriers for transdermal delivery of hydrophobic and hydrophilic local anesthetics. Drug Deliv 15:323–30
- Labouta HI, Schneider M. (2010). Tailor-made biofunctionalized nanoparticles using layer-by-layer technology. Int J Pharm 395:236–42
- Li L, Zhang Y, Han S, et al (2011). Penetration enhancement of lidocaine hydrochlorid by a novel chitosan coated elastic liposome for transdermal drug delivery. J Biomed Nanotechnol 7:704–13
- Li P, Liu D, Miao L. (2012). A pH-sensitive multifunctional gene carrier assembled via layer-by-layer technique for efficient gene delivery. Int J Nanomedicine 7:925–39
- Mandapalli PK, Labala S, Vanamala D, et al (2014). Influence of charge on encapsulation and release behavior of small molecules in self-assembled layer-by-layer microcapsules. Drug Deliv 21:605–14
- Müller RH, Shegokar R, Keck CM, et al (2011). 20 years of lipid nanoparticles (SLN and NLC): present state of development and industrial applications. Curr Drug Discov Technol 8:207–27
- Musawi AA, Andersson L. (2010). Use of topical as only anesthetic for suturing a traumatic facial laceration. Dent Traumatol 26:292–3
- Ouchi K, Sekine J, Koga Y, et al (2013). Establishment of an animal model of sedation using epidural anesthesia that uses the tail-flick test for evaluating local anesthetic effects in rats. Exp Anim 62:137–44
- Pardeike J, Hommoss A, Müller RH, et al (2009). Lipid nanoparticles (SLN, NLC) in cosmetic and pharmaceutical dermal products. Int J Pharm 366:170–84
- Pathak P, Nagarsenker M. (2009). Formulation and evaluation of lidocaine lipid nanosystems for dermal delivery. AAPS PharmSciTech 10:985–92
- Pignatello R, Basile L, Puglisi G. (2009). Chitosan glutamate hydrogels with local anesthetic activity for buccal application. Drug Deliv 16:176–81
- Pino CJ, Scherer MA, Shastri VP, et al (2014). Investigation of the transdermal transport of charged local anesthetics in the presence of triterpene saponin glycosides. Drug Deliv Transl Res 4:131–8
- Puglia C, Sarpietro MG, Bonina F, et al (2011). Development, characterization, and in vitro and in vivo evaluation of benzocaine- and lidocaine-loaded nanostructrured lipid carriers. J Pharm Sci 100:1892–9
- Ramasamy T, Tran TH, Choi JY, et al (2014). Layer-by-layer coated lipid-polymer hybrid nanoparticles designed for use in anticancer drug delivery. Carbohydr Polym 102:653–61
- Sapra B, Jain S, Tiwary AK. (2008). Transdermal delivery of carvedilol containing glycyrrhizin and chitosan as permeation enhancers: biochemical, biophysical, microscopic and pharmacodynamic evaluation. Drug Deliv 15:443–54
- Shim J, Kim MJ, Kim HK, et al (2010). Morphological effect of lipid carriers on permeation of lidocaine hydrochloride through lipid membranes. Int J Pharm 388:251–6
- Vitorino C, Almeida J, Gonçalves LM, et al (2013). Co-encapsulating nanostructured lipid carriers for transdermal application: from experimental design to the molecular detail. J Control Release 167:301–14
- Wang Y, Su W, Li Q, et al (2013). Preparation and evaluation of lidocaine hydrochloride-loaded TAT-conjugated polymeric liposomes for transdermal delivery. Int J Pharm 441:748–56
- Xavier AC, de Moraes ML, Ferreira M. (2013). Immobilization of aloin encapsulated into liposomes in layer-by-layer films for transdermal drug delivery. Mater Sci Eng C Mater Biol Appl 33:1193–6
- Yang C, Shen Y, Wang J, et al (2015). Cationic polymer-based micro-emulgel with self-preserving ability for transdermal delivery of diclofenac sodium. Drug Deliv 22:814–22
- Yang JA, Kim ES, Kwon JH, et al (2012). Transdermal delivery of hyaluronic acid: human growth hormone conjugate. Biomaterials 33:5947–54
- Yuan JS, Acosta EJ. (2009). Extended release of lidocaine from linker-based lecithin microemulsions. Int J Pharm 368:63–71
- Zhang G, Liu F, Jia E, et al (2016). Folate-modified, cisplatin-loaded lipid carriers for cervical cancer chemotherapy. Drug Deliv 23:1393–7