Abstract
Objective: The objective of the present study was to develop novel PMV [poly (methacrylic acid-co-vinyl triethoxylsilane)]-coated mesoporous silica nanoparticles (MSN) with improved hypoglycemic effect for oral insulin (INS) delivery.
Methods: MSN was synthesized under acidic condition using Pluronic® P 123 and Tetra ethoxy orthosilane. Surfactant was removed by calcination. Calcined MSN was coated with pH sensitive polymer PMV. Cytotoxicity of this coated MSN was evaluated by MTT assay using CHO-K1 cell line. Different MSN samples were characterized with BET surface area analyzer, FESEM, TEM, FT-IR, XRD, TG-DTA. In vivo study was performed using male rats. Pharmacokinetic study was conducted using HPLC.
Results and discussion: Highest surface area (304.3921 m2/g) was observed in case of calcined sample. Adsorption pore width of final coated sample was highest (64.7844 nm) compared with others. No noticeable cytotoxicity was observed for this coated support. The entrapment efficiency of insulin was found to be 39.39%. In vitro studies were done at different pH using Franz-diffusion cell. Results showed significant release at pH 7.4. Cumulative drug release over a period of 6 h was more than 48% at this systemic pH. Effect of this MSN-PMV-INS on blood glucose level was retained for 16 h. This novel formulation has shown 73.10% relative bioavailability of insulin.
Conclusion: A novel-coated mesoporous silica support was successfully developed for delivery of insulin through oral route.
Introduction
Oral delivery of protein and peptide drugs still remains a fascinating challenge to parenteral delivery due to its significant challenge to the biomedical researchers worldwide. Enzymatic degradation, low permeability, acidic environment of stomach, rapid clearance of GI tract are major glitches in this context (Mabato et al., Citation2003; Shen, Citation2003; Thompson et al., Citation2010). Insulin, a dual chain therapeutic peptide, is the drug of choice for treatment of patients with type-I diabetes. On the other hand, when it is administered orally less than 0.1% reaches the systemic circulation due to its enzymatic degradation during the passage in the GI tract (Lowman et al., Citation1999). Several scientific investigations have been carried out to increase the bioavailability through oral route as it is still the most convenient and safe route of administration. Currently, subcutaneous route is the only option available for insulin dependent diabetic patients. Many smart attempts have been made by researchers for improving insulin absorption after oral administration. To date, many structurally stable Drug Delivery System (DDS), such as dendrimer (Shen et al., Citation2010), liposome (Hosta-Rigau et al., Citation2010), inorganic nanomaterials (Urbina et al., Citation2008), hydrogel microparticles (Raghavendra et al., Citation2011a) etc. have proved great potential in this field. Among them mesoporous silica nanoparticle (MSN) has showed promising result for immobilization of therapeutic biomolecules (Wong et al., Citation2004; Lee et al., Citation2005). This novel DDS has attracted lot of interest due to its high surface area, pore volume, stability and excellent biocompatibility (Stein et al., Citation2000; Vallet-Regí & Balas, Citation2006).
Earlier MSN-based DDS was designed according to morphological and physical properties of porous support. However, in these systems physically adsorbed guest molecule would release instantaneously after administration, which would produce undesirable adverse effects to normal cells. Therefore, it is highly desirable to design a “smart” DDS, which can release the entrapped cargo at proper site in a controlled fashion upon exposure to certain stimuli. Many successful attempts including gate keeping strategy have been applied to produce stimuli responsive MSN-based DDS. From dendrimer to quantum dot (Lai et al., Citation2003; Radu et al., Citation2004), many gatekeepers have explored to cap the pore of MSN for preventing undesirable leaching of cargo from the porous inorganic support. However, most of these systems are intricate to synthesize, and the detached caps might bring toxic reaction to normal cells and organs. Besides gate-keeping concept, stimuli responsive such as pH responsive MSN-based DDS have attracted lot of attention among researchers. Smart polymers like poly (dimethyldiallylammonium chloride) (Yang, Citation2005), poly (2-diethylaminoethyl methacrylate) (Sun et al., Citation2010), poly (acrylic acid) (Yuan et al., Citation2011) etc. have been used by researchers to generate polymer-MSN hybrids. To the best of our knowledge, most reported polymer-MSN hybrids have been prepared by three methods – layer-by-layer technique (Wang et al., Citation2005), graft-to (Carniato et al., Citation2010) and graft-from technique (Liu et al., Citation2011). Defiance of their novelty these strategies still possess some pitfalls such as tedious step synthesis, very low surface graftings efficiency etc. (Fleming et al., Citation2001). It is still challenging for the researchers to explore a facile and efficient method for producing a hybrid that can controllably deliver entrapped guest molecule.
Poly (methacrylic acid-co-vinyl triethoxylsilane) (PMV), a pH-sensitive polymer was first described by Gao et al. (Citation2009). Methacrylic acid (MAA) and vinyl triethoxylsilane (VTES) were employed to synthesize this smart polymer.
It is well known that nanoparticles are capable of protecting guest molecule from acid catalyzed degradation. To stop further leaching, MSN was coated with PMV, a biodegradable pH responsive polymer. Few researchers have prepared coated MSN for drug and protein delivery. Moreover several scientific investigations were carried out by researchers on pH responsive polymer-based devices like hydrogels (Kiran et al., Citation2013), microspheres (Raghavendra et al., Citation2011b) etc. for delivery of insulin orally (Babu et al., Citation2008). However, no such report has been documented on PMV-coated MSN for oral delivery of insulin. Herein, we have synthesized a novel PMV-coated insulin-loaded bean shaped MSN (MSN-PMV-INS) for controlled delivery of therapeutic cargo i.e. insulin through oral route. Bioavailability has been increased compared to other scientific investigation.
MSN was synthesized in acidic condition following S0H + X− I+ pathway (S0-non-ionic surfactant; X− – anionic counter ion: I+ – silicate species) using non-ionic tri-block copolymer (P123) as structure directing agent and tetra ethoxy silane (TEOS) as silica precursor (Zhao, Citation1998; Zhao et al., Citation1998).
Insulin with smaller dimension had easy access to the larger pore (64.78 nm) of MSN. Insulin was loaded onto MSN following physical adsorption strategy, which remains simplest approach for immobilization of biomolecule. In addition, this process is usually not directly affecting the active sites. As weak interactions involves in this scheme, we prepared coated MSN which ensured “zero” premature release.
Materials and methods
Materials
Insulin human solution, Tetra ethoxy silane (TEOS) and 1, 3, 5 tri methyl benzene (TMB), Pluronic® P123, Azodiisobutyronitrile were procured from Sigma Aldrich (St. Louis, MO). Analytical grade chemicals like water, hydrochloric acid, ethanol, etc. were purchased from Merck Ltd., Mumbai, India.
Synthesis of MSN
In a typical preparation (), 2 g of Pluronic® P123 was dissolved in 52 g water and 20 ml (37%) HCl with stirring at 35 °C. Then, 4.28 g of TEOS was added into the solution with vigorous stirring at 35 °C for 24 h (Zhao, Citation1998). The mixture was aged at 100 °C overnight without stirring. Trimethylbenzene (TMB) was added during synthesis in a 1:1 ratio with the surfactant. The solid product was filtered and washed repeatedly with water and ethanol. The product was dried in an oven at 90 °C for 20 h.
Calcination
The polymeric template was removed from MSN through thermal treatment. Isothermal annealing of the silica sample was done at 550 °C for 6 h in a PID controlled Muffle furnace (Grieken et al., Citation2003).
Preparation of PMV and coating of MSN
PMV [Poly(methacrylic acid co-venyl triethoxy silane)] was synthesized by the radical mediated polymerization of the monomers, methacrylic acid (MAA) and vinyl triethoxy silane (VTES) using azodiisobutyronitrile under nitrogen atmosphere (Gao et al., Citation2009).
For preparation of PMV-coated MSN two separate ethanolic solution were prepared by dissolving 100 mg of each PMV and MSN in 5 ml ethanol. The solutions were mixed in a reaction vessel under constant in nitrogen atmosphere overnight. The mesoporous nanoparticles were collected by filtration, washed with deionized water and freeze dried. The dried product was stored in refrigerator prior to further studies.
Cytotoxicity assay (MTT)
Cytotoxicity evaluation of MSN-PMV (test) and doxorubicin (standard) was performed using MTT assay. Approximately 1 × 105 cells/ml (CHO-K1) in their exponential growth phase were seeded in a flat-bottomed 96-well polystyrene-coated plate and were incubated for 24 h at 37 °C in a CO2 incubator. Series of dilution (0.13, 0.41, 1.23, 3.70, 11.11, 33.33, 100 μg/ml) of MSN-PMV (coated MSN) and doxorubicin (10, 5, 2.5, 1.25, 0.62, 0.31, 0.15 μM) in the medium was added. After 24 h of incubation, 10 μl of MTT reagent was added to each well and was further incubated for 4 h. After 4 h, formazan crystals formed in each well were dissolved in 150 μl of DMSO and the plates were read instantly in a microplate reader (Spectramax M5, Sunnyvale, CA) at 570 nm. All experiments were conducted in triplicate. Wells with complete medium, coated nanoparticles (MSN-PMV) and MTT reagent, without cells were used as blanks for cell viability determination. Untreated cells exposed to the medium were used as solvent control (Vijayakumar & Ganesan, Citation2012).
Drug loading
50 mg coated MSN was added in 5 ml of phosphate buffer pH 7.8, vortex mixed for 10 min and kept under constant stirring at 4 °C. 7.5 mg insulin (30 units) was added to this dispersion dropwise. The stirring was continued for 10 hrs. Few drops of 0.1 N HCl solution was then added to this mixture to reduce the pH to 4.0 so that PMV coating shrinks to prevent undesired release of loaded insulin from the pores of MSN. The mixture was removed from the stirrer and the nanoparticles were collected by filtration and freeze dried.
Characterization
Nitrogen sorption isotherms at 77 K
Different MSN samples were characterized by nitrogen gas desorption/adsorption isotherm using Surface area analyzer (Micromeritics Gemini VII-2390t, Norcross, GA). It was then measured at −196 °C whereby the samples were degassed at 150 °C for 4 h in the degassing port of the adsorption apparatus. Total surface was determined using Brauner-Emmett-Teller (BET) model in the relative pressure range between 0.05 and 0.3.The pore volume and pore size distribution were derived from the adsorption branches of the isotherms using Barrett-Joyner-Halenda (BJH) method. Total pore volume was estimated from the amount adsorbed at a relative pressure of 0.99. Micropore volume and micropore areas were measured from t-plot. t values were calculated as a function of relative pressure using Harkins and Jura equation.
TG-DTA
Thermal decomposition of the (Pluronic® P123 template) sample was determined by thermogravimetry (TG) and differential scanning calorimetric analysis (DSC) using a Pyris Diamond TG/DTA (Perkin Elmer, Waltham, MA) instrument.
XRD
X-ray diffraction (XRD) was used to identify the crystal phases of the MSN materials. XRD data was collected at room temperature using Cu-Kα radiation of wavelength 1.5404 Å on a Rigaku UltimaIII (Tokyo, Japan) X-ray diffractometer (Bragg–Brentano geometry) at 40 kV and 36 mA.
FTIR
Room temperature Infrared (IR) spectra of all the samples were taken on IR-Prestige, Shimadzu spectrometer using DRS (Diffuse Reflectance Spectroscopy) technique in the range between 400 and 4000 cm−1.
FESEM and TEM
The shape and surface morphology of different MSN samples were investigated using a field emission scanning electron microscope (FESEM, Hitachi S-4800, Tokyo, Japan)
TEM images were recorded on a TEM system (JEOL, Tokyo, Japan) with an acceleration voltage 200 kV. Finely ground samples are dispersed in ethanol and placed in an ultrasonic bath and the suspension was then dropped on carbon-coated copper grids.
Drug entrapment efficiency
Entrapment efficiency of PMV-coated insulin-loaded MSNs were determined by suspending in phosphate buffer (pH 7.8) under magnetic stirring for 10 h at 4 °C. Upon completion of stirring, the solution was filtered through Whatman filter paper (grade 41). The amount of entrapped insulin in the filtrate was studied using UV-Visible spectrophotometer at 275 nm wavelength. The drug entrapment efficiency was calculated by the following equation (Yuan et al., Citation2011).
In vitro release
The release profiles of MSN-PMV-INS were performed in distinct dissolution media of pH 1.2 and pH 7.4 at 37 °C under agitation in a franz-diffusion cell of 100 ml capacity. At particular time intervals, 1 ml of sample was withdrawn and instantly replaced with an equal volume of dissolution medium to maintain the sink condition. The collected samples were filtered using Whatman (grade 41) filter paper and analyzed at 275 nm using UV-Vis Spectrometer (JASCO V-550 Double Beam UV, Tokyo, Japan) to find out the insulin content.
Pharmacodynamic studies
Animals
Adult albino male rats weighting 200–220 g were used for pharmacodynamic studies. Before initiation of this experiment, the rats were acclimatized for a period of 14 days. Standard environmental conditions such as temperature (25 ± 2 °C), relative humidity (45–55%), and 12-h day–night cycles were perpetuated during experiment. Rats were rendered diabetic prior to the study by intraperitoneal injection of 60 mg/kg streptozotocin in 0.1M citrate buffer (pH = 4.5). Rats having blood glucose level more than 250 mg/dL were considered diabetic. All of the animal experiments adhered to the principles of care and use of laboratory animals and approved by the Institutional Animal ethical Committee of Jadavpur University, Kolkata.
Oral administration approach
Twenty four diabetic rats were randomly divided into four groups (six animals for each group). All animals were fasted for 12 h before the experiment, though they had free access to water throughout the experiment. The rats in group 1 were given 1 ml of saline solution orally as normal controls. The rats in group 2 were subcutaneously injected with insulin solution in a dose of 0.036 mg/kg (equivalent to 1 IU/kg) as a positive control. The rats in groups 3 were given insulin solution orally. Group 4 animals were given MSN-PMV-INS orally. Insulin doses were 0.54 mg/kg (equivalent to 15 IU/kg) for group 3 and group 4 animals. All above formulations were in the medium of physiological saline. The oral administration volume of solution was in the range of 1 ml/100 g.
Blood samples were collected from the retroorbital plexus of each rat at different time interval. Blood glucose was determined in the plasma sample by the glucose-oxidase method (Glucose test kit-GOD-POD method, Span Diagonistic Ltd., Gujarat, India)
Pharmacokinetic studies
Animals
In-vivo studies were performed on six New Zealand male albino rabbits weighing between 1.2 and 1.5 kg in accordance with a protocol by the institutional ethical committee, Jadavpur University. Animals were kept in individual cage. Standard diet and water ad libitum were given supplied to the animals throughout the experiment. Rabbits were divided into two groups containing six rabbits each. First group was administered subcutaneous injection of insulin at the dose of 1 IU/kg and 20 mg MSN-PMV-INS (equivalent to 15 IU/kg) was administered orally in second group.
Instrumentation and chromatographic condition
A high performance liquid chromatography (Shimadzu, Kyoto, Japan) was composed of a LC-20AD Prominence solvent delivery module, a manual Rheodyne injector with a 20 μl fixed loop and a SPD-M20A Prominence Diode array detector. Separation was performed on a Phenomenex C18 column (particle size 5 micrometer; 250 mm∗4.6 mm i.d., Phenomenex, Torrance, CA) at ambient temperature. Analysis was done at 1.0 ml/min flow rate with ACN: 0.2 M sodium sulfate buffer (adjusted to pH 2.8) (30:70, v/v) as mobile phase. The eluted peak was detected at 214 nm.
Blood samples were collected from marginal ear vein of the rabbit into heparinized micro-centrifuge tube (ependorff) and the plasma was separated by centrifugation at 2000 rpm for 10 mins. 100 μl of blank plasma was further transferred to ependorff tube and spiked with 0.5 ml of each known concentration of insulin solution prepared in phosphate buffer pH 7.4. Sample was extracted with 0.7 ml dichloromethane (DCM) by rotating for 10 min. The tube was then centrifuged at 5000 rpm for 10 min to separate layer. Then the tubes were immersed in dry ice bath. Supernatant layer was decanted and the organic layer was collected and kept in the refrigerator overnight for complete evaporation of DCM. The dried precipitate was reconstituted with 0.2 ml of 0.01N HCl. 0.2 ml of 5 μg/ml of ortho-cresol was added to this reconstituted solution. The samples were then filtered through a 0.2 μm membrane filter using syringe filter holder and 20 μl was injected into the column. The chromatogram was recorded at 215 nm. The developed method was validated for linearity, accuracy, precision, selectivity, and sensitivity in accordance with US FDA method validation guidelines (US Department of Health and Human Services, Citation2001).
Pharmacokinetic analysis
In all, 0.5 ml of blood samples were collected at the interval of 5 min, 10 min, 30 min, 45 min, 60 min, 90 min, 120 min, 180 min for Gr-I and 1 h interval up to 24 h for Gr-II in heparinized ependorff tube after administration. These samples were centrifuged immediately at 2000 rpm at 4 °C for 10 min. Plasma samples were stored in refrigerator prior to assay. Pharmacokinetic data were analyzed using Kinetica 5.1 (Thermo Fisher Scientific, Waltham, MA).
Results and discussion
Cytotoxicity assay
Cells which are viable after 24 h exposure to the sample were competent of metabolizing a dye (3-(4,5-dimethylthiozol-2-yl)-2,5-diphenyl tetrazolium bromide) proficiently and the purple colored precipitate which is dissolved in DMSO was studied spectrophotometrically. After 24 h of post treatment, CHO-K1 cells showed excellent viability even up to the concentration of 100 μg/ml of coated nanoparticles as shown in . Doxorubicin was used as a standard cytotoxic drug and its observed cytotoxicity (IC50= 0.265) standardized the assay. These results clearly demonstrate that the PMV-coated MSN has no cytotoxicity which justify safety aspect of this novel composite. The lack of any noticeable toxicity of these smart nanoparticles may explore a newer avenue in the field of drug delivery.
Characterization
Bet analysis
BET surface area plot, N2 desorption adsorption isotherm and pore size distribution analysis were carried out for all the MSN sample and the results are shown in the and . A huge increase in surface area of the heat treated MSN sample (), 304.3921 m2/g was observed compared to the as synthesized MSN sample (), 42.7893 m2/g, which ensures the effective loading of the biomolecule within the mesopores of the MSN. This increase in surface area was due to the removal of surface bound Si-OH (silanol) groups. All the isotherms () for as synthesized, calcined and PMV-coated MSN respectively) obtained were of type IV according to IUPAC indicating the mesoporous nature of the silica sample. H1-type Hysteresis loops () with sharp adsorption and desorption branches were observed for as synthesized, calcined, PMV-coated MSN samples respectively which signifies capillary condensation taking place in the mesopore of MSNs. A distribution in pore width with average of 6.16 nm was observed for the as synthesized MSN sample (inset of ) which was due to the presence of both meso- and micro-pores within the sample. According to literature (Bérubé & Kaliaguine, Citation2008), swelling agent upto a certain ratio can expand pore size of the final product. Herein, we have added 1, 3, 5 trimethylbenzene to increase the pore size. It produces positive result (size 6.16 nm) compared to those without TMB. However, after calcination, the pore width reduces sufficiently (inset of ). This reduction in pore width can be assigned to the shrinkage of the hexagonal structure and closing of the mesopore (Gao et al., Citation2009). The highest pore width of the coated sample (64.78 nm) has ensured loading of insulin with smaller dimension. The observed changes in surface area and pore width in the final sample () were obviously due to the effect of the coating of the PMV, which cover the surface and pores of the MSN sample.
FTIR analysis
FTIR spectra of different samples were shown in . A comparison of the FTIR spectra of as synthesized, calcined, MSN-PMV, pure insulin and MSN-PMV-INS samples were shown in . Samples other than insulin showed the characteristic peaks of silica (Si-O-Si) network and the surface silanol (Si-OH) group at 1300–400 cm−1and 1630 cm−1 respectively (Tian et al., Citation2002). The characteristic C–O–C linkage at 1730 cm−1 and the Si-C bond at 1340-1460 cm−1 confirmed the presence of Pluronic® P123 in the as synthesized silica sample (McDonald, Citation1958). Pluronic® P123 or TMB molecule showed three small peaks at 2970, 2934 and 2880 cm−1 due to the C(sp3)-H stretching vibration (Mishler, Citation2009). The broad absorption peak for all the samples in the range of 3700–3000 cm−1 was due to the presence of different kind of water molecules associated with the MSN structure (Etzkorn, Citation1999). Insulin-loaded PMV-MSN nanoparticles showed two characteristic peaks of protein at 1650 cm−1 and 1540 cm−1 for amide I and amide II, respectively. The monomers of insulin consists of many ionizable groups like six amino acid residues which are capable of attaining a positive charge and 10 amino acid residues are capable of attaining a negative charge (Brange, Citation1987). These properties of insulin have profound effect on insulin loading into PMV-coated MSN nanoparticles.
TG-DTA and XRD analysis
Thermo-gravimetric (TG) and differential thermal analysis (DTA) of the as synthesized MSN sample was shown in the . The result suggests that the decomposition of the pluronic triblock co-polymer template (Pluronic® P123) was accomplished at 600 °C. However, the silica maintains its integrity at this temperature or even above it. Thus a heat treatment temperature of 600 °C for 6 h would be adequate for the efficient removal of the template. During the shrinkage of the hexagonal structure, the mesopore volume is generally decreased but the micropore volume remains almost constant (Tian et al., Citation2002; Bérubé & Kaliaguine, Citation2008). Wide angle x-ray diffraction patterns of different MSN samples were shown in the . All the diffraction patterns signify the typical amorphous nature of the silica matrix.
FESEM and TEM analysis
Surface morphology of the as synthesized, calcined and the coated samples analyzed by FESEM were shown in the , respectively. As the image shows (), the as synthesized silica sample contains cylindrical shaped particles with typical aspect ratio (p = L/d) of 2. The particles also shows tendency to form linear type chain by overlapping head-to-tail. In some portion, we also observed some irregular shaped aggregates of the particles as shown in the inset of the . After calcined at 550 °C, the shape and size of the particles remain intact (see ) signifying the excellent thermal stability of the silica sample. However, after coating with PMV, individual particles agglomerated and give rise to bigger assembly (see ) which includes some hollow-sphere like structure as shown in the inset of . shows high resolution TEM image of the as-synthesized sample. Pores with diameter in the range of 15–20 nm are clearly visible, which strongly support the mesoporous characteristic of the sample. A representative low magnification TEM image also shown in the inset of .
Drug loading
Protein was loaded onto Mesoporous support strategically. Insulin was loaded in phosphate buffer at pH 7.4 as PMV dissolves at this systemic pH. 39% entrapment efficiency was obtained. After hours, pH was reduced to 4 to block pore opening of MSN as polymer coating shrinks at low pH. Thus, insulin-loaded PMV-coated MSN was prepared.
In vitro release
In vitro insulin release from coated MSN (MSN-PMV-INS) was performed at pH 1.2 and 7.4. Only 13% release of insulin was observed within first 2 h at pH 1.2. No further release was perceived at this pH. This 13% release may be due to the insulin adhered to the surface of coated MSN (MSN-PMV). As PMV coat dissolves at pH 7.4, no additional release was noticed after 2 h at pH 1.2. illustrates the release profile of insulin from MSN-PMV-INS at various pH. Results depict that the cumulative insulin release from the coated mesoporous support was more than 48% within 6 hr. These results refer pH responsive behavior of PMV, which govern controlled release of entrapped cargo. Moreover this PMV coating prevents undesirable leaching of biomolecule and thus it protect insulin from proteolytic breakdown in strong acidic gastric environment. Through mesenteric lymph-loaded and -coated MSN reaches systemic circulation where release of entrapped insulin takes place.
Pharmacodynamic study
PMV-coated MSN (PMV-MSN-INS) as oral insulin delivery system was assessed by measuring blood glucose levels in rats with streptozotocin-induced diabetes. The hypoglycemic profiles of the different groups of diabetic rats receiving subcutaneous injection, oral PMV-MSN-INS, oral insulin solution and normal saline were shown in . SC injection of 1 IU/kg insulin solution reduced blood glucose level very fast, almost 60% within 2 h and returned to the basal value after 12 h of administration. Oral insulin solution of 15 IU/kg showed minimum effect on blood glucose level and the differences from the group treated as blank was insignificant. Degradation of oral insulin solution by the protease enzyme present in gastric barrier may be the reason of this negligible effect. The diabetic rat group received oral PMV-MSN-INS containing 15 IU/kg insulin showed a small reduction in blood glucose level in first 2 h, followed by a sharp fall upto next 6 h which was maintained for almost 16 h. These results demonstrated that the PMV-MSN-INS possessed significantly stronger oral antidiabetic activities than oral insulin solution. PMV-coated MSN suspension quickly uptaken by m cells of Peyer’s patch and through mesenteric lymph it directly reaches to systemic circulation which has promoted the rapid reduction of blood glucose in diabetic rats. pH sensitive coating of PMV prevented premature leaching of insulin in stomach and protected it from getting degraded by the enzymes in the gastric region.
Pharmacokinetic studies
Mean plasma concentration–time curves of insulin after administration of SC injection and oral MSN-PMV-INS formulation in rabbits were shown in . The pharmacokinetic parameters including maximum peak concentration of the drug in plasma (Cmax), the time to reach maximum concentration (tmax), half life (t1/2), area under the curve (AUC) and minimum residential time (MRT) were shown in . After oral administration of MSN, plasma insulin concentration increased slowly and attained maximum concentration after 615 min. Then a steady fall in the concentration was noted for 1440 min. The formulation showed a good relative bioavailability of insulin, ∼73.10%. These results suggest that the uptake of intact gastrointestinal MSN particles occurred by the mechanisms involving M-cells in Peyer’s patches of the gastrointestinal lymphoid tissue (Jani et al., Citation1990; Clark et al., Citation2001; Florence & Hussain, Citation2001). Oral delivery of insulin presents a challenge because of its poor oral absorption. The main hurdle faced by insulin in the GI tract is degradation by proteolytic enzymes and lack of transport across the intestinal epithelium. Encapsulation of insulin in PMV-coated MSN particle promoted insulin paracellular or transcellular transport across the intestinal mucosa. The PMV coat degraded at pH 7.4 in the blood and insulin was released from MSN in a sustained fashion. The evidence of intact uptake of mesoporous silica nanoparticles (30–90 nm) was found in previous studies (Zhang, Citation2012).
Figure 9. Pharmacokinetic release profiles of insulin after oral administration of MSN-PMV-INS and S.C. administration of insulin solution in rabbit model.
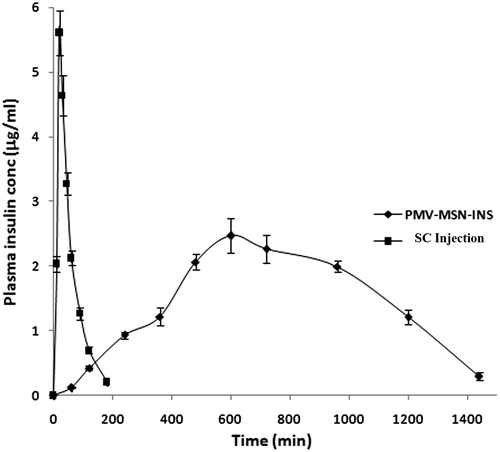
Table 1. Textural characteristics of different MSNs in relation to BET Surface area, mesopore surface area and micropore surface area and pore width.
Table 2. Comparative pharmacokinetic parameters of insulin following administration of subcutaneous injection and oral PMV-coated insulin-loaded MSN (MSN-PMV-INS).
Conclusion
In summary, we have designed a novel-coated mesoporous carrier for delivery of insulin via oral route. In this report, therapeutic insulin was successfully loaded onto mesoporous support (MSN). pH responsive behavior of PMV has helped in monitoring insulin release. Compatibility of excipients was confirmed from FT-IR studies. Pharmacodynamic and pharmacokinetic response of orally administered MSN-PMV-INS has proved potential of this novel formulation. Moreover this approach might be considered as potential alternative in the field of oral delivery of insulin. In future, this system can be fabricated using smart tactic to improve its efficacy. More site and time-specific release of cargo could be achieved through modification of the said strategy.
Declaration of interest
The authors report no declarations of interest. We greatly acknowledge Indian Council of Medical Research (ICMR), India for funding this adhoc project [35/52/2010-BMS].
References
- Babu VR, Pradip P, Raghavendra CM, et al (2008). Developments in polymeric devices for oral insulin delivery. Expert Opin Drug Deliv 5:403–15
- Bérubé F, Kaliaguine S. (2008). Calcination and thermal degradation mechanisms of triblock copolymer template in SBA-15 materials. Microporous Mesoporous Mater 115:469–79
- Brange J. (1987). Galenics of insulin: the physico-chemical and pharmaceutical aspects of insulin and insulin preparations. Berlin: Springer
- Carniato F, Bisio C, Paul G, et al (2010). Salvatore Coluccia and Leonardo Marchese, on the hydrothermal stability of MCM-41 mesoporous silica nanoparticles and the preparation of luminescent materials. J. Mater Chem 20:5504–9
- Clark MA, Jepson MA, Hirst BH. (2001). Exploiting M cells for drug and vaccine delivery. Adv Drug Deliv Rev 50:81–106
- Etzkorn T. (1999). Gas-phase absorption cross sections of 24 monocyclic aromatic hydrocarbons in the UV and IR spectral ranges. Atmos Environ 33:525–40
- Fleming MS, Mandal TK, Walt DR. (2001). Nanosphere-microsphere assembly: methods for core-shell materials preparation. Chem Mater 13:2210–6
- Florence AT, Hussain N. (2001). Transcytosis of nanoparticle and dendrimer delivery systems: evolving vistas. Adv Drug Deliv Rev 50:S69–89
- Gao Q, Xu Y, Wu D, et al (2009). pH-responsive drug release from polymer-coated mesoporous silica spheres spheres. J Phys Chem C 113:12753–8
- Grieken RF, Calleja G, Stucky GD, et al (2003). Supercritical fluid extraction of a nonionic surfactant template from SBA-15 materials and consequences on the porous structure. Langmuir 19:3966–73
- Hosta-Rigau L, Chandrawati R, Saveriades E, et al (2010). Noncovalent liposome linkage and miniaturization of capsosomes for drug delivery. Biomacromolecules 11:35–48
- Jani PU, Halbert GW, Langridge J, Florence AT. (1990). Nanoparticle uptake by the rat gastrointestinal mucosa: quantitation and particle size dependency. J Pharm Pharmacol 42:821–6
- Kiran C, Kuntal G, Mallikarjuna NN, Tejraj MA. (2013). Polymeric hydrogels for oral insulin delivery. J Control Release 165:129–38
- Lai CY, Trewyn BG, Jeftinija DM, et al (2003). A mesoporous silica nanosphere-based carrier system with chemically removable CdS nanoparticle caps for stimuli-responsive controlled release of neurotransmitters and drug molecules. J Am Chem Soc 125:4451–9
- Lee CH, Wong ST, Lin TS, Mou CY. (2005). Characterization and biomimetic study of a hydroxo-bridged dinuclear phenanthroline cupric complex encapsulated in mesoporous silica: models for catechol oxidase. J Phys Chem B 109:775
- Liu R, Liao P, Liu J, Feng P. (2011). Responsive polymer-coated mesoporous silica as a pH-sensitive nanocarrier for controlled release. Langmuir 27:3095–9
- Lowman AM, Morishita M, Kajita M, et al (1999). Oral delivery of insulin using pH-responsive complexation gels. J Pharm Sci 88:933–7
- Mabato RI, Narang AS, Thoma L, Miller DD. (2003). Emerging trends in oral delivery of peptide and protein drugs. Crit Rev Ther Drug Carrier Syst 20:153–214
- McDonald RS. (1958). Surface functionality of amorphous silica by infrared spectroscopy. J Phys Chem 62:1168–78
- Mishler IIRE. (2009). Solvent-washable polymer templated synthesis of mesoporous materials and solid-acid nanocatalysts in one-pot. Chem Commun 41:6201–3
- Radu DR, Lai CY, Jeftinija K, et al (2004). A polyamidoamine dendrimer-capped mesoporous silica nanosphere-based gene transfection reagent. J Am Chem Soc 126:13216–7
- Raghavendra CM, Vidhya R, Tejraj MA. (2011a). pH-Sensitive oral insulin delivery systems using Eudragit microspheres. Drug Dev Ind Pharm 37:977–85
- Raghavendra CM, Vidhya R, Tejraj MA. (2011b). Poly(N-vinylcaprolactam-co-methacrylic acid) hydrogel microparticles for oral insulin delivery. J Microencapsul 28:384–94
- Shen WC. (2003). Oral peptide and protein delivery: unfulfilled promises? Drug Discov Today 8:607–8
- Shen YQ, Zhou Z, Sui M, et al (2010). Charge-reversal polyamidoamine dendrimer for cascade nuclear drug delivery. Nanomedicine 5:1205
- Stein A, Melde BJ, Schroden RC. (2000). Hybrid inorganic–organic mesoporous silicates—nanoscopic reactors coming of age. Adv Mater 12:1403–19
- Sun JT, Hong CY, Pan CY. (2010). Fabrication of PDEAEMA-coated mesoporous silica nanoparticles and pH-responsive controlled release. J Phys Chem C 114:12481–6
- Thompson CJ, Tetley L, Cheng WP. (2010). The influence of polymer architecture on the protective effect of novel comb shaped amphiphilic poly (allylamine) against in vitro enzymatic degradation of insulin—towards oral insulin delivery. Int J Pharm 383:216–27
- Tian B, Lui X, Yu C, et al (2002). Microwave assisted template removal of siliceous porous materials. Chem Commun 11:1186–7
- Urbina MC, Zinoveva S, Miller T, et al (2008). Investigation of magnetic nanoparticle − polymer composites for multiple-controlled drug delivery. J Phys Chem C 112:11102
- US Department of HEALTH and Human Services. (2001). Food and drug administra-tion, guidance for industry: bioanalytical method validation. Rockville, MD: Centre for Drugevaluation and Research
- Vallet-Regí M, Balas F. (2006). Revisiting silica based ordered mesoporous materials: medical applications. J Mater Chem 16:26–31
- Vijayakumar S, Ganesan S. (2012). In vitro cytotoxicity assay on gold nanoparticles with different stabilizing agents. J Nanomater 2012:1–9
- Wang YJ, Yu AM, Caruso F. (2005). Nanoporous polyelectrolyte spheres prepared by sequentially coating sacrificial mesoporous silica spheres. Angew Chem 117:2948–52
- Wong ST, Lee CH, Lin TS, Mou CY. (2004). Preparation and characterization of MCM-41-supported hydroxo-bridged dicupric–phenanthroline complex. J Catal 228:1–11
- Yang Q. (2005). pH-responsive carrier system based on carboxylic acid modified mesoporous silica and polyelectrolyte for drug delivery. Chem Mater 17:5999–6003
- Yuan L, Tang Q, Yang D, et al (2011). Preparation of pH-responsive mesoporous silica nanoparticles and their application in controlled drug delivery. J Phys Chem C 115:9926–32
- Zhang Y. (2012). Mesoporous silica nanoparticles for increasing the oral bioavailability and permeation of poorly water soluble drugs. Mol Pharm 9:505–13
- Zhao DY. (1998). Triblock copolymer syntheses of mesoporous silica with periodic 50 to 300 angstrom pores. Science 279:548–52
- Zhao DY, Huo Q, Feng J, et al (1998). Nonionic triblock and star diblock copolymer and oligomeric surfactant syntheses of highly ordered, hydrothermally stable, mesoporous silica structures. J Am Chem Soc 120:6024–36