Abstract
A kind of polymeric lipid vesicles (PLVs) with pH-responsive turning on–off membrane for programed delivery of insulin in gastrointestinal (GI) tract was developed, which was self-assembled from the grafted amphipathic polymer of N-tocopheryl-N′-succinyl-ɛ-poly-l-lysine (TP/SC-g-PLL). By controlling the grafting ratio of hydrophobic alkane and ionizable carboxyl branches, the permeability of membrane was adjustable and thus allowing insulin release in a GI-pH dependent manner. The effects of grafting degree of substitution (DS) on the pH-responsive behavior of the formed vesicles were confirmed by critical aggregation concentration determination, morphology and size characterization. Their transepithelial permeability across the GI tract was proved by both confocal visualization in vitro model of Caco-2 cellular monolayer and in vivo hypoglycemic study in diabetic rats. Accordingly, the work described here indicated that the self-assembled PLVs could be a promising candidate for improving the GI delivery of hydrophilic biomacromolecule agents.
Introduction
Recent advances in molecular biology and genetic recombination technology have allowed production of therapeutic proteins and peptides in commercial quantities (Bakhru et al., Citation2013). However effective absorption of these recombinant agents, especially via the oral route, often suffers great challenges before their clinical applications (Morishita & Peppas, Citation2006; Gupta et al., Citation2013). Due to their large molecular radius, hydrophilic properties and nonresistance to enzymatic degradation, very low permeability across the lipid intestinal mucosa and limited amounts of intact active agents available for systemic absorption are often suffered when these biomacromolecules are administrated via the gastrointestinal (GI) tract (Kammona & Kiparissides, Citation2012).
Therefore novel delivery systems with enough drug capacity, permeation-enhancing ability, and protective effect against adverse external conditions, controllable drug release and insignificant potential toxicological issues are highly on demand (Goldberg & Gomez-Orellana, Citation2003). Enteric coating together with addition of absorption enhancers and digestive enzyme inhibitors has been proposed as a common strategy to achieve the goal (Mitragotri et al., Citation2014). However the sufficient permeability along with no apparent damage on biological membranes still remains challenge. In the past years, nano-particulate carriers made from biodegradable polymers have provided us a promising alternative to increase the uptake and transport of orally administered therapeutic proteins (Adair et al., Citation2010; Torchilin, Citation2014). It has been shown that by endocytosis, generally considered as an energy-dependent process, nanomedicines transport across the epithelium membrane without destroying their integrity (Duncan & Richardson, Citation2012; Xu et al., Citation2013).
Here, we propose a kind of environmentally stimuli-responsive polymeric lipid vesicles (PLVs) with programed drug release and improved absorbability for delivery of insulin via GI route. As a kind of artificial vesicles, PLVs are characterized as nanosized hollow shells that provide an aqueous inner core and lipid interlayer where the hydrophilic and hydrophobic substances can be encapsulated and thus segregated from external environment, respectively (Discher & Eisenberg, Citation2002). Different from common liposomes that composed of small lipid molecules, PLVs are constructed with amphiphilic grafted polymers to form the bilayer membrane (Lee & Feijen, Citation2012). Therefore, the polymeric vesicles exhibit resistance to bile salt damages and thus, higher stability than that of liposomes in GI tract (Christian et al., Citation2009). Furthermore, if some stimuli-sensitive moieties were introduced in the building blocks for membrane construction, the intelligent ones with programed drug release responding to external stimuli, such as pH, temperature, redox potential and magnetic field could be available (Meng et al., Citation2009; Wang et al., Citation2014).
Herein this study, an amphiphilic comb-branched polymers of N-tocopheryl-N′-succinyl-ɛ-polylysine were first synthesized and characterized in chemical structure. The grafted polymers will self-assemble into core–shell vesicles and encapsulate model protein in inner water phase. Upon exposing to low pH condition of gastric acid, the carboxylic acid moieties in grafted polymers are dominated in undissociated form and thus leading to an enhanced hydrophobic interaction between the polymers. As a result, poor drug release rate was expected due to the shrinkage of the polymeric shells. In the case of intestinal juice, pH-induced ionization of carboxyl groups in alkaline environment will lead to mutual electrostatic repulsion between the grafted polymers and loose vesicle structure, thus accelerated release of encapsulated drug was expected. Scheme 1 shows the proposed structure of PLVs used in this study. By controlling the grafting ratio of hydrophobic alkane branches, the permeability of membrane was adjustable and thus making it possible for stimuli-responsive release and uptake synchronously. The physical characteristics of the self-assembled PLVs, including morphology, particle size, surface charge, drug-loading capacities and pH-sensitive behaviors in vitro were studied. To verify the absorption efficiency of the developed vesicles, both in vitro transport ability across Caco-2 monolayer and in vivo pharmacokinetics were evaluated.
Materials and methods
Materials
Epsilon-poly-l-lysine (ɛ-PLL) with molecular weight of 3800–4000 were obtained from Sai Taisi Biotechnology Co., Ltd. (Nanjing, China). Alpha-tocopherol (α-TP) was supplied by Xinchang Pharmaceutical Co., Ltd. (Zhejiang, China). The 1-ethyl-3-(3-dimethylaminopropyl) carbodiimide hydrochloride (EDC, ≥99.0%), N-hydroxysuccinimide (NHS, 98%), 4-(dimethylamino) pyridine (DMAP, ≥99.0%), 2,4,6-trinitrobenzene sulfonic acid (TNBS, 5%(w/v) in H2O), pyrene (≥99.0%), fluorescein isothiocyanate labeled insulin (FITC-Ins), rhodamine-labeled phalloidin and Hoechst 33258 (≥98.0%) were purchased from Sigma-Aldrich (St. Louis, MO). All other reagents and chemicals were of analytical grade.
Synthesis of N-tocopheryl-N′-succinyl-ɛ-PLL (TP/SC-g-PLL)
Synthesis of α-tocopheryl succinate (α-TPS)
The α-TP and succinic anhydride were dissolved in acetone at the molar ratio of 1/2. DMAP was added as catalyst of the esterification reaction at 1.0% weight ratio of reactant. Then the mixture was allowed stirring in nitrogen atmosphere for 4 h at 50 °C. After removing of solvent by rotary evaporation under vacuum, the crude product was washed to neutral with 5% sodium chloride solution. The followed purification by recrystallization in petroleum ether was performed to give the white compound.
Synthesis of N-tocopheryl-ɛ-PLL (TP-g-PLL)
The grafted polymers were synthesized following a modified procedure reported by our group previously (Liang et al., Citation2012). Briefly, α-TPS were dissolved in DMSO and equal amount (1.5 equivalents of α-TPS) of EDC and NHS were added to activate the carboxyl group. Then ɛ-PLL was introduced at various molar ratios to α-TPS and the reaction mixture was kept in agitation at room temperature in dark condition. After a further 24 h incubation, the product was dialyzed against distilled water (MWCO: 1.5 kDa, Viskase Companies Inc., Chicago, IL) successively to remove the reactant remains. The product, N-tocopheryl-ɛ-PLL (TP-g-PLL) was dried under vacuum at 40 °C overnight.
Synthesis of N-tocopheryl-N′-succinyl-ɛ-PLL (TP/SC-g-PLL)
The following succinylation was carried out by co-dissolving TP-g-PLL and succinic anhydride in DMSO at different molar ratio. The mixture was allowed to magnetical stirring at 40 °C for 12 h. The reaction solution was dialyzed against distilled water and following by vacuum drying at 40 °C for recovering the TP/SC-g-PLL powder.
Characterization of grafted polymers
The chemical structures of the grafted polypeptide were analyzed using 1H nuclear magnetic resonance (NMR) spectroscopy (Bruker AVACEAV-500, Billerica, MA) in deuterated dimethyl sulfoxide (DMSO-d6) at 300 MHz. Fourier transform infrared (FT-IR) spectra were recorded on a Bruker IFS55 spectrometer (Bruker, Switzerland) in the range of 4000–500 cm−1. Samples were prepared by mixing sample powder with KBr and scanned at 2 cm−1 resolution. The degree of substitution (DS) of the amino groups in ɛ-PLL was determined by the TNBS method (Tong et al., Citation2010), in which quantitative determination of the number of amines contained within a sample can be accomplished through comparison to a standard curve generated by the use of an amine-containing compound dissolved in a series of known concentrations. The critical aggregation concentration (CAC) of grafted polymers was determined by fluorescence probe technique that involves the use of pyrene based hydrophobic fluorescence dye which exhibits different fluorescence characteristics depending upon the polarity of the local environment (Du et al., Citation2012). The excitation spectra of pyrene at 375 nm (I1) and 384 nm (I3) were recorded on a fluorescence spectrophotometer (F-2500, Hitachi, Tokyo), respectively. The intensity ratio of I3 to I1 was plotted as a function of polymer concentrations to calculate the CAC.
Preparation and characterization of PLVs
The PLVs were prepared according to the modified thin-film hydration method (Pang et al., Citation2010). Briefly, 1 mL chloroform containing 60 mg of the synthesized grafted polymers was subjected to the rotary evaporation under vacuum in a 100-mL round bottom flask. After removal of solvent, the resulting dried polymer film was hydrated overnight with deionized water. The obtained suspensions were subjected to extrusion to make monodispersed vesicles. Then freeze drying were employed for subsequent physical characterizations. For the drug loaded PLVs, the model therapeutic protein of FITC-Ins was added in deionized water during the preparation.
The surface morphology of PLVs in various pH conditions was examined by transmission electron microscopy (TEM) with JEM-1200EX (Tokyo, Japan), which was negatively stained with 0.01% phosphotungstic acid and placed on a copper grid coated with film. The hydrodynamic diameter, polydispersity and zeta potentials of the PLVs were measured by dynamic light scattering (DLS) analysis using Zetasizer Nano ZS90 (Malvern Instruments, Malvern, UK). The amount of FITC-insulin entrapped within the PLVs was determined indirectly, i.e. by measuring the amount of free ones with the RP-HPLC analysis in the supernatant recovered after ultracentrifugation. The drug entrapment efficiency (E.E%) was expressed as a percentage of the fluorescence difference between the initial amount of FITC-Ins and the free amount in the supernatant relative to the total amount used for the PLVs preparation.
In vitro drug release
In vitro release of FITC-Ins from the vesicles was performed in 50 mL of phosphate buffer, in which the medium pH was changed from 2.0 to 7.4 to simulate the physiological conditions of the whole GI tract. The experiment was kept at 37 °C with continuous orbital mixing (50 r/min). At appropriate intervals, the entire suspensions were subjected to refrigerated ultracentrifugation at 20 000 rcf for 10 min. The resulted pellets were re-suspended in equal volume of fresh dissolution medium. The amount of insulin dissolved in the supernatant was evaluated by RP-HPLC analysis (Cui et al., Citation2006). The cumulative insulin percentage dissolved from the self-assemblies was calculated as the ratio of the amount of insulin dissolved at time (t) to the initial amount used.
Cytological studies
Cell culture
Caco-2 cells were cultured in Dulbecco’s Modified Eagle Medium (DMEM) supplemented with 10% fetal bovine serum, 1% non-essential amino acids, 2 mmol/L l-glutamine and an antibiotic/antimycotic solution consisting of 100 U/mL penicillin and 100 μg/mL streptomycin. Cells were seeded at a density of 4 × 105 cells per 75 cm2 flasks and maintained at 37 °C in an atmosphere of 5% CO2 and 90% relative humidity. The culture medium was changed every other day and passage operations were performed with 0.25% trypsin – 0.02% EDTA once the cells reached 80–90% confluency.
Cytotoxicity test
Cytotoxicity of PLVs was carried out using a MTT assay to evaluate their biocompatibility. Briefly, Caco-2 cells were seeded onto 96-well plates at a density of 104 cells per well. After a 48 h culture, the culture medium was replaced by the fresh one containing grafted polypeptide with final concentration of 0–0.2 mg/mL. After 24 h of incubation, the medium was removed and 100 μL of MTT solution (0.5 mg/mL in HBSS) was added for another 3 h of incubation at 37 °C. Then the formed formazan was dissolved by 150 μL of DMSO and the absorbance of each well was measured at 490 nm with a microplate reader (Bio-Rad Model 550, Hercules, CA). The relative cell viability was expressed as the absorbance difference between the test samples and negative control.
Transport studies through Caco-2 cell monolayers
Transepithelial permeability of PLVs was evaluated by transport across a Caco-2 cellular monolayer. Cells were seeded at density of 1.0 × 105/mL onto Transwell® polycarbonate filters (Corning Costar Corp., Cambridge, MA) with 3.0 μm mean pore size and growth area of 1.1 cm2. Following 21–24 days of monolayer growth until they achieved a constant transepithelial electrical resistance (TEER) not less than 500 Ω/cm2, the cells were washed and pre-equilibrated for 1 h with Hank's balanced salt solution (HBSS). Then the apical to basolateral (AP-BL) permeability experiment was started by replacing the donor chamber medium with fresh pre-warmed HBSS (buffered at pH 6.8) containing FITC-Ins loaded PLVs. The free FITC-Ins buffer solution with equivalent dose (50 μg/mL) was set as control. In all cases, the basolateral medium used was HBSS buffered at pH 7.4. During incubation at 37 °C for 4 h, aliquots were withdrawn from the receiver chamber at different time intervals and the samples were analyzed by fluorescence spectrophotometry (Sajeesh et al., Citation2010). At the end of the experiment, TEER values were measured in triplicate to assess the integrity of the cell monolayers. The apparent permeability coefficients (Papp, cm/s) were calculated based on the equation: Papp= (dQ/dt)/(A⋅C0⋅60), where dQ/dt is the cumulative transport rate (μg/min) defined as the slope obtained by linear regression of cumulative transported amount as a function of time (min), A is the surface area (1.12 cm2 in 12-wells) of the monolayers, C0 is the initial concentration of the samples on the donor side (μg/mL).
Confocal laser scanning microcopy (CLSM)
Caco-2 cells were seeded on glass cover slides in 24-well plates at a density of 5 × 104 cells/cm2. Fifteen days after seeding, the growth medium was replaced with FITC-Ins loaded PLVs in HBSS (buffered at pH 6.8) and incubation was performed at 37 °C for 2 h. Then the cells were washed three times with cold HBSS and then fixed with 4% paraformaldehyde. Cells were permeabilized using 0.2% Triton X-100 in blocking solution containing 1% bovine serum albumin in HBSS for 10 min. Then the cells were subjected to F-actin and nuclear staining by incubation with rhodamine–phalloidin solution (0.2 μg/mL) and Hoechst 33258 (1 μg/mL) at room temperature, respectively. After washing with HBSS, cover slips were mounted on slides and examination was performed by the confocal laser scanning microscope (Olympus FV1000-IX81, Japan).
Enzymatic degradation study
The protective effect of PLVs against enzymatic degradation in vitro was carried out in phosphate buffer (pH 6.8) at 37 °C, in which insulin loaded PLVs were incubated in the presence of trypsin (10 000 U/mg) at an enzyme/insulin molar ratio of 1:150. At appropriate intervals, aliquots (0.1 mL) of suspension were withdrawn and 0.4 mL ice acetonitrile containing 0.1% of trifluoroacetic acid and 1% of Triton X-100 were added to terminate enzyme activity and dissociate the vesicles. The samples were then evaluated by RP-HPLC analysis to determine the amount of remaining insulin. Free insulin solution were set as control.
Animal studies
Streptozotocin-induced male diabetic Sprague–Dawley rats (200 ± 20 g, blood glucose levels ≥16 mmol/L) were used for hypoglycemic experiments. The animals were fasted overnight but had free access to water prior to the experiments. All procedures were conducted in accordance with the Animal Ethical Guidelines for Investigations in Laboratory Animals and were approved by the Ethical Committee for Animal Experimentation of the Shenyang Pharmaceutical University. The animals were randomly assigned to three groups (six rats per group) followed by intragastric administration of insulin solution and TP/SC3-g-PLL2 at a single dose of 25 IU/kg, respectively. Positive control group received insulin solution (1 IU/kg) via subcutaneously injection. Blood samples were collected from the jugular vein of the rats at different times after dosing. Plasma was separated by centrifugation to determine the plasma glucose level using the glucose oxidase method (GOD kit, Beijing BHKT Clinical Reagent Co., Ltd., China). The relative pharmacological bioavailability (PB) after intragastric administration was calculated according to the equation: PB = (AACoral×Dosesc)/(AACsc×Doseoral) × 100%, where AAC is the total area above the curve of the plasma glucose concentration versus time profile.
The results were examined for statistically significant differences using a one-way analysis of variance (ANOVA) among more than three groups or Student’s t-test between two groups. The p value less than 0.05 and 0.01 were considered to be statistically significant and highly significant, respectively.
Results and discussion
This work investigated the potential of using a polyamino acid based PLVs for GI delivery of hydrophilic proteins. Native ɛ-poly-l-lysine (ɛ-PLL) is a natural linear homopolymer that comes from essential amino acid of l-lysine and is believed to be cytocompatible (Hyon et al., Citation2014), which makes it an attractive starting material for the synthesis of novel biopolymers for biomedical applications (Hamano, Citation2011). In consideration of harsh environment in GI tract, it is important to transport active macromolecules to the target site precisely and safely with a controlled release. Simultaneously, effective uptake and permeation across intestine mucosa should be guaranteed to achieve the best therapeutic effect. Thus we hypothesize that by hydrophobically modifying ɛ-PLL with native tocopherol branches at appropriate grafting ratio, the polypeptide can be converted into a derivative that self assembles into core–shell structure to protect active agents from too fast release before their absorption by enterocytes (). In addition, stimuli-responsive release was performed by succinylation of primary amino groups in ɛ-PLL according to pH changes of physiological environment in GI tract. The ionization of the carboxyl moieties in weakly alkaline medium of target sites is expected to loose the vesicle membrane by improving hydrophilicity of polymer skeleton, as a result making the contents release.
Design and synthesis of grafted polymers
The grafted polymers were synthesized by conjugating tocopheryl and succinyl moieties serially onto the primary amino groups of ɛ-PLL under the catalysis of EDC and NHS, as shown in Scheme 1. In the designed derivatives, the tocopheryl moieties were used as hydrophobic unit to form vesicles and the other amino residues of ɛ-PLL were substituted with succinyl ones to make it GI pH responsive. As shown in 1H NMR analysis (), the proton peaks of TP-g-PLL at 0.85 ppm and 0.9–1.2 ppm were attributed to the methyl and methylene ones of the long-chain alkyl groups of α-TPS, respectively. Furthermore, the double peaks at 1.8–2.0 ppm belonged to the methyl hydrogen of the benzopyran moiety. Both the results proved the successful conjugation of tocopheryl branches on the polymer skeleton. Compared to that of TP-g-PLL, the newly appeared signals of TP/SC3-g-PLL2 at 12.4 ppm came from the active hydrogens of carboxyl group in hemisuccinate. In addition, the relative intensity of peak at 2.5–2.7 ppm which belongs to the methane hydrogen (–COCH2CH2–) was increased in TP/SC3-g-PLL2 significantly, indicating that the subsequent amino substitutions with succinyl moieties were achieved.
Figure 2. 1H NMR spectra of α-TPS (A), TP-g-PLL (B) and TP/SC3-g-PLL2 (C) in DMSO-d6. (D) FT-IR spectra of ɛ-PLL (a), TP-g-PLL (b) and TP/SC3-g-PLL2 (c).
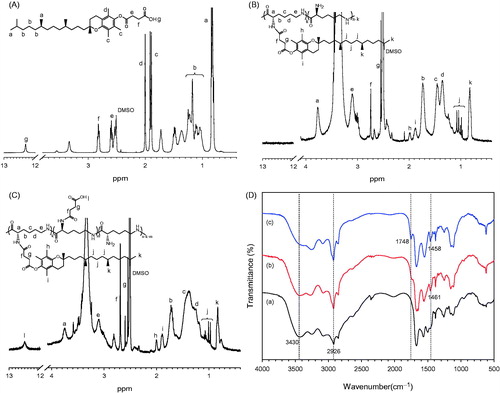
The conjugation of tocopheryl and succinyl moieties to ɛ-PLL was further confirmed by FT-IR analysis. depicts FT-IR spectra of ɛ-PLL, TP-g-PLL and TP/SC3-g-PLL2, two main peaks were observed at 3430 cm−1 and 2926 cm−1, which originated from the υN–H of primary amine and υC–H of methylene, respectively. Compared with that in ɛ-PLL, an increase in the intensity of υN–H peak at 2926 cm−1 and consequent decrease at 3430 cm−1 were observed in both TP-g-PLL and TP/SC3-g-PLL2, suggesting that the substitutions of amino group by tocopheryl moieties were occurred. This reaction can also be proved by the emerging peaks in both TP-g-PLL and TP/SC3-g-PLL2 at 1458–1461 cm−1, that assigned to the C–H bending vibration (δC–H) of methyl and methylene. Furthermore, a new signal was found in TP-g-PLL and especially strengthened in TP/SC3-g-PLL2 at 1748 cm−1 corresponding to C=O stretching vibration (υC=O), indicating the conjugation of succinyl group.
The degree of alkyl acylation (DSacyl) and succinylation (DSsuc) in ɛ-PLL, defined as the number of α-TPS and succinyl moieties per 100 amino acid residues of ɛ-PLL, was determined by TNBS method (). It was found that with increase in molar ratio of α-TPS to the amino group of ɛ-PLL, the DSacyl was increased gradually. In view of the fact that protonation of amino groups in ɛ-PLL will lead to more hydrophilic of the grafted polymers and rapid leakage of contents in gastric acid environment, the residual amino groups in ɛ-PLL were substituted using excess succinic anhydride. It was found that less than 10% of amino group remained, when the three-fold molecular ratio of succinic anhydride to the remaining amino acid residues of ɛ-PLL was employed.
Table 1. Characteristics of the grafted polymers (n = 3).
pH-sensitive characteristics of grafted polymers
The CAC is defined as the concentration of amphiphilic polymers above which vesicles form and all additional polymers added to the system go to vesicles (Smart et al., Citation2010). It is an important parameter that involves the aggregation behavior of polymer vesicles in solution. The lower CAC indicates the stronger self-assembly ability of the amphiphilic polymers and the more structural stability of vesicles in various solutions. A fluorescence measurement was carried out using pyrene as the fluorescent probe to determine the CAC values of grafted polymers with different DS in deionized water. It is well known that the ratio between the fluorescence intensity of the first (375 nm) and third (384 nm) emission peaks of pyrene, denoted as I3/I1, is indicative of the polarity level of its microenvironment. Therefore CAC values represent the ability of various grafted polymers for vesicles formation. As shown in and , an increase of tocopheryl moieties conjugation lead to a decrease of CAC values significantly in the same medium (deionized water). However as seen in , the values were enhanced in the case of representative grafted polymer (TP/SC3-g-PLL2) when the medium pH increased from 2.0 to 8.0 (3.5 μg/mL versus 21.4 μg/mL), indicating an instability of the formed PLVs in alkaline environment.
Figure 3. (A) Changes of pyrene fluorescence intensity ratio (I3/I1) in deionized water as a function of the grafted polymer concentration with different substitution degrees by tocopheryl and succinyl moieties. (B) The concentration of representative grafted polymer (TP/SC3-g-PLL2) dependence of pyrene fluorescence intensity ratio (I3/I1) in different media.
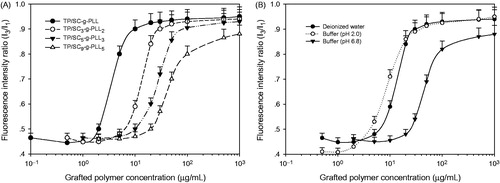
The pH-sensitive characteristics of the grafted polymers induced by succinyl moieties were further verified by observing the change in shape and membrane size of formed vesicles at different pH conditions. As shown in of TEM micrographs, the grafted polymers formed typical vesicles in buffers at pH 2.0 that were characterized as hollow-spherical shape. The mean hydrodynamic size and shell thickness of the self-assembled vesicles were in the range of 80–85 nm and 12–15 nm (), respectively. When the pH value of hydration medium increased to 6.0, the particle diameter and shell thickness increased to 90–95 nm and 20–25 nm, respectively (). This significant swelling of vesicle shell was attributed to the ionization of carboxyl moieties and thus results in mutual electrostatic repulsion between the two layers of PLVs. As seen in , further increasing the pH value of hydration medium to 8.0 will lead to break of the PLVs.
Formation and characterization of PLVs
Self-assembled PLVs were prepared by simple thin-film hydration and following extrusion method. In the presence of aqueous solution, the hydrophilic succinyl groups are attracted to the water in the environment and form a surface facing the outer and inner aqueous phase. The hydrophobic tail groups in the two layers, which are made of a long hydrocarbon chain of the grafted peptide, were repelled by water and thus face each other to form a bilayer structure. The model macromolecule of FITC-Ins was loaded passively into the grafted polymer originated PLVs with different DS. Their physicochemical properties, such as hydrodynamic diameter size (DH), polydispersity index (PDI), zeta potentials and drug encapsulation efficiency (E.E%) are summarized in .
Table 2. Characteristics of the PLVs self-assembled from various grafted polymers in different media (n = 3).
It was found that in the strong acidic environment with pH lower than 2.5, all of self-assembled PLVs were positive charged slightly due to protonation of small amount of the residual primary amino groups in ɛ-PLL. Moreover, their hydrodynamic diameters were in the range of 75–90 nm. Further increasing in pH values of hydration medium would lead to gradual decrease in zeta potentials of self-assembled PLVs and make them negative charged at pH above 3.5–4.0 (). As a result, the vesicles suffered swelling in particle size (such as 90–110 nm at pH 6.8) due to the ionization of carboxyl moieties and thus resultant mutual electrostatic repulsion between their membranes ().
Figure 5. (A) Changes of surface zeta potential of PLVs self-assembled from various grafted polymers as a function of hydration medium pH values. (B) Typical hydrodynamic diameters of PLVs at pH 2.0 and pH 6.8 medium. (C) In vitro release profiles of insulin from PLVs in simulated digestion liquid with different pH values.
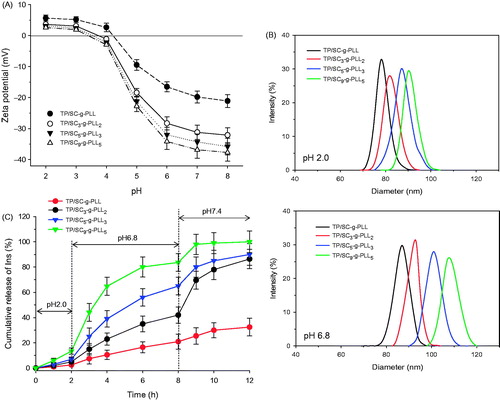
pH-dependent release behaviors of PLVs
In vitro release profiles of insulin from various PLVs were investigated in buffer solution with different pH values. As shown in , there is a strong dependence of the release rates on both the medium pH values and graft tocopheryl ratio. When incubated in acid medium at pH 2.0, all of the vesicles showed negligible cumulative release amounts with no more than 15% of loaded insulin during the first two hours. In contrast, an enhanced release profile was found in medium at pH 6.8 with cumulative release of 18.5% (TP/SC-g-PLL), 37.2% (TP/SC3-g-PLL2), 58.6% (TP/SC5-g-PLL3) and 75.1% (TP/SC9-g-PLL5) of loaded FITC-Ins released in the same period. It was also found that at the same pH condition, the PLVs with different tocopheryl DS presented different drug release behaviors. As there was increase in tocopheryl DS, the pH sensitivity of vesicles was decreased. It was found that no more 30% of loaded insulin released from TP/SC-g-PLL formed vesicles in pH 6.8 medium. However, almost complete drug release was found in TP/SC9-g-PLL5 based ones in the same period.
It is considered that both the ionizable entities and hydrophobic lipid chain give the PLVs pH dependent membrane permeability. The long-chain alkyl groups of α-TPS aggregate to form a membrane via hydrophobic interaction. Attachment of hydrophobic branches to linear water soluble polymers has long been used as a means for obtaining commercial polymer additives called “associative thickeners” that are used to boost the viscosity of solutions and suspensions (Cambon et al., Citation2014). This boost is thought to occur because the associative thickener self-assembles into a transient network, with contacts between the hydrophobic branches acting as physical crosslinks. Thus by hydrophobically modifying native ɛ-PLL with tocopheryl branches of appropriate grafting ratio, we can convert it into a derivative that self assembles into physical networks in vesicle membranes. Meanwhile, succinyl groups are expected to exist as the pH-sensitive moieties between the hydrophilic surface and the hydrophobic membrane. Due to their calculated pKa of 4.5–4.7, more than 95% of the succinyl moieties are to be ionized in buffer with pH above 6.8. As a result, the generated static electricity repulsion disrupts the well-ordered packing of tocopheryl branches thus inducing the contained cargoes to diffuse in through a loosely packed membrane.
In vitro cytotoxicity studies
To estimate their biocompatibility, the Caco-2 cell viability was measured by a typical MTT method after 48 h of exposure to various grafted polymers at serial concentrations. As shown in , all the vesicles from different grafted polymers were found to have no significant cytotoxicity (p > 0.05) and more than 90% of Caco-2 cells survived at the tested concentrations. The results suggested that the grafted polymers were nontoxic and could be candidates for oral delivery application with good biocompatibility.
Figure 6. (A) The viability of Caco-2 cells after 24 h exposure to various grafted polymer formed PLVs. Statistically significant difference from control (*p < 0.05). (B) Cumulative amounts of FITC-Ins transported across Caco-2 cell monolayers from TP/SC9-g-PLL5 and TP/SC3-g-PLL2 formed PLVs as a function of time. The free FITC-Ins solution was set as control. (C) The apparent permeability coefficients (Papp, cm/s) of free FITC-Ins solution, TP/SC9-g-PLL5 and TP/SC3-g-PLL2. (D) Changes in transepithelial electrical resistance (TEER, %) during the transport experiment in Caco-2 cell monolayer.
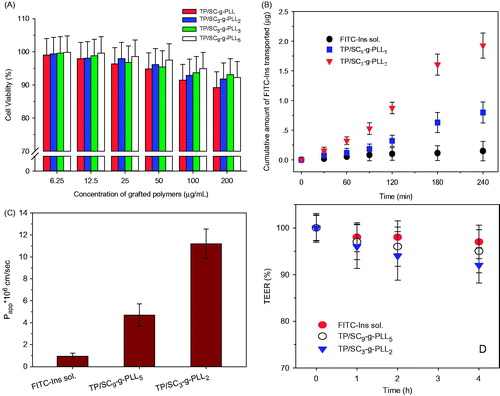
The native ɛ-PLL is recognized as a very safe substance in the scientific community, which has been approved by FDA for use as an antimicrobial agent at levels up to 50 mg/kg of cooked rice (Hiraki et al., Citation2003). The results of an acute toxicity study show that there was no toxicity in reproduction, neurological and immunological functions, embryonic and fetal development, growth offspring and development of embryos, etc. Nevertheless, few reports address ɛ-PLL use in biomaterials for drug delivery.
Transport of PLVs across Caco-2 cell monolayers
The effects of PLVs on the permeability of FITC-Ins across intestinal epithelial cells were evaluated in vitro using a Caco-2 monolayer model. After exposure of the cellular monolayer to vesicles with different DS, the cumulative transported amounts of FITC-Ins from AP to BL compartment were determined to calculate their apparent permeability coefficients (Papp, cm/s). As illustrated in , all of test groups showed a time-dependent progressive increase in the cumulative amount of FITC-Ins transported across the cell monolayer. However, the free FITC-Ins solution group showed a negligible cumulative amount of FITC-Ins transported through Caco-2 cell monolayers (). In contrast, the Papp of both TP/SC9-g-PLL5 (4.7 × 10−6 cm/s) and TP/SC3-g-PLL2 (11.2 × 10−6 cm/s) was increased significantly (p < 0.01), suggesting the PLVs possess the ability of promoting enterocyte permeability of macromolecules. It was also found that as the tocopheryl grafting ratio increases, the permeability of FITC-Ins across cellular monolayer enhanced significantly (p < 0.05). This result is in agreement with the previous reports that surface hydrophobicity plays a significant role in the GI bioavailability of nanoparticles (Sakuma et al., Citation2001; Jain et al., Citation2016). The improved surface hydrophobicity facilitates partition of nanoparticles into lipid-rich cell membrane due to the mucosa hydrophobic interaction.
It is worth mentioning that there were no significant changes in the TEER values prior to and after the transport experiment (), indicating that the presence of the vesicles had little influence on the cellular monolayer and that the integrity of the Caco-2 monolayer was well preserved during the experiments. Furthermore, actin cytoskeleton of Caco-2 was labeled with rhodamine conjugated phalloidin (red fluorescence) to verify the effects of vesicles on the integrity of cellular monolayer (). It can be found that for all of test groups, the Caco-2 cells remained an intact distribution of actin with continuous and intense fluorescence staining around the cell nucleus. The results further confirmed what was already observed in and again suggested that the intercellular tight junction was not changed significantly after exposing to the PLVs.
Figure 7. Confocal laser microscope images of cellular uptake of free FITC-Ins solution, FITC-Ins loaded TP/SC9-g-PLL5 and TP/SC3-g-PLL2 for 2 h.
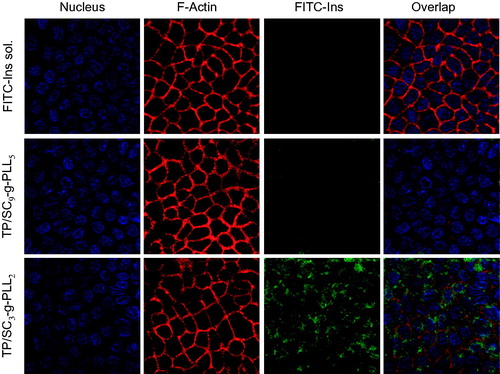
It is believed that potential routes of cargoes (such as nanoparticles and micelles) across GI mucosal epithelial cells might be paracellular transport via tight junctions and transcellular transport (passive or active) via the enterocyte barrier (Salama et al., Citation2006). There are many reports about absorption enhancement effects of polycations by opening intercellular tight junctions, e.g. poly-l-arginine (Ohtake et al., Citation2003), chitosan (du Plessis et al., Citation2010; Rosenthal et al., Citation2012), poly-l-lysine and polyethylenimine (Ranaldi et al., Citation2002). This action is always followed by decrease in TEER and integrity of cell monolayers, and thus leads to risk of infection. In the case of this study, less than 10% reduction in the TEER values of the monolayer throughout the transportation experiments was found, indicating that the integrity of Caco-2 cell monolayers was conserved. This result was also evidenced by CLSM observation, in which no evident alterations in the F-actin cytoskeleton were detected following treatment of the cell monolayers with PLVs. As a result, PLVs transferred across the monolayer without paracellular route due to their negative charge. This finding was consistent with previous reports, in which particles with negative charge achieve permeation while not disrupting cellular tight junctions (Mo et al., Citation2011). Thus we can conclude that transepithelial transport of PLVs occurs by non-paracellular pathway without disrupting the Caco-2 cell monolayers.
Cellular uptake of PLVs
The effects of the different DS on the intracellular uptake of cargo loaded PLVs were further compared visually by CLSM. As shown in , there was no apparent green FITC fluorescence signal of free FITC-Ins observed in cells, suggesting the non-absorbability of this macromolecule. In contrast, the intense green fluorescence of both PLVs was found to locate around nucleus, indicating that they were internalized by the Caco-2 cells and their transport across cell monolayers by an intracellular way. It was also found that as the tocopheryl DS increased, the cellular fluorescence intensity of FITC became stronger. All of these observations were consistent to the results in transport studies.
Protective effect of PLVs against enzymatic degradation
As shown in , insulin is sensitive to trypsin at intestinal pH. After 2 h of incubation, less than 10% of the protein was remained. In contrast, the encapsulation with PLVs leads to the enhanced enzyme resistance of insulin. After 4 h of incubation with trypsin, the remained insulin percent was more than 50%. As is known that ɛ-PLL is a basic homo-polypeptide containing 25–30 l-lysine residues (Yoshida & Nagasawa, Citation2003). It is naturally secreted by various Streptomycetaceae and by some filamentous fungi. In contrast to normal polypeptide bond that is linked by the alpha-carbon group, the lysine molecules are molecularly linked by the epsilon (ɛ) amino group and the alpha carboxyl group. As a consequence of this linkage, it is not hydrolyzed by proteases, such as pepsin and trypsin (Tsujita et al., Citation2006). Therefore, their formed carriers were resistant to degradation by proteases that hydrolyze typical peptide bonds.
Figure 8. (A) Percent of remained insulin as a function of time in enzymatic degradation study. (B) The plasma glucose levels of insulin solution and insulin loaded TP/SC3-g-PLL2 after intragastric administration at 25 IU/kg in diabetic rats. Subcutaneous administration of insulin solution (1 IU/kg) was used as positive control. Data represents the mean ± SD, n = 6 per group.
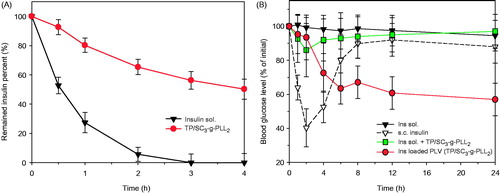
In vivo hypoglycemic studies
In order to confirm their potential use for oral delivery, the pharmacological effects and pharmacokinetic behaviors of the self-assembled vesicles were evaluated in diabetic rats via intragastric administration at a single dose of 25 IU/kg. The changes in level of plasma glucose versus time in vivo are shown in . As expected, intragastric administration of insulin solution at 25 IU/kg has shown limited efficiency to reduce the blood glucose level. In contrast, a significant reduction in plasma glucose (percentage relative to the initial value) was observed in vesicle group (p < 0.05), especially 4–8 h after administration (p < 0.001). Peroral administration of 25 IU/kg insulin loaded PLVs did not show a significant hyperglycemic effect within the first 2 h, however reduced the fasting plasma glucose level to 72% and 63% at 4 h and 6 h post administration, respectively. The pharmacological availability calculated relative to the subcutaneous injection of 1 IU insulin/kg was 8.5%. The observed hypoglycemic effects in diabetic rats agree with the findings in transport studies across Caco-2 cell monolayers and give evidence that PLVs could be a promising candidate for oral delivery of therapeutic proteins.
Conclusions
In this work, a kind of pH-responsive grafted polymers was successfully synthesized, which can self-assemble into bioabsorbable PLVs with tunable on–off membrane for programed delivery of model protein of insulin in GI tract. In vitro and in vivo studies showed that the PLVs could release insulin in a pH-responsive manner and all of the blank vesicles were nontoxic to Caco-2 cells. All of these results allow us to conclude that the grafted polymer based PLVs are potential candidates to improve the GI delivery of hydrophilic therapeutic proteins due to their biocompatible, bioabsorbable and environmental responsive features.
Declaration of interest
The authors wish to thank the National Natural Science Foundation of China (No. 31170967), Liaoning province high school talent support program (No. LJQ2013103) and Shenyang Pharmaceutical University young teachers’ career development support plan for financial support. The authors report no declarations of interest.
References
- Adair JH, Parette MP, Altinoglu EI, et al. (2010). Nanoparticulate alternatives for drug delivery. ACS Nano 4:4967–70.
- Bakhru SH, Furtado S, Morello AP, et al. (2013). Oral delivery of proteins by biodegradable nanoparticles. Adv Drug Deliv Rev 65:811–21.
- Cambon A, Figueroa-Ochoa E, Juarez J, et al. (2014). Complex self-assembly of reverse poly(butylene oxide)–poly(ethylene oxide)–poly(butylene oxide) triblock copolymers with long hydrophobic and extremely lengthy hydrophilic blocks. J Phys Chem B 118:5258–69.
- Christian DA, Cai S, Bowen DM, et al. (2009). Polymersome carriers: from self-assembly to siRNA and protein therapeutics. Eur J Pharm Biopharm 71:463–74.
- Cui F, Shi K, Zhang L, et al. (2006). Biodegradable nanoparticles loaded with insulin-phospholipid complex for oral delivery: preparation, in vitro characterization and in vivo evaluation. J Control Release 114:242–50.
- Discher DE, Eisenberg A. (2002). Polymer vesicles. Science 297:967–73.
- Du Plessis LH, Kotze AF, Junginger HE. (2010). Nasal and rectal delivery of insulin with chitosan and N-trimethyl chitosan chloride. Drug Deliv 17:399–407.
- Du Y, Chen W, Zheng M, et al. (2012). pH-sensitive degradable chimaeric polymersomes for the intracellular release of doxorubicin hydrochloride. Biomaterials 33:7291–9.
- Duncan R, Richardson SC. (2012). Endocytosis and intracellular trafficking as gateways for nanomedicine delivery: opportunities and challenges. Mol Pharm 9:2380–402.
- Goldberg M, Gomez-Orellana I. (2003). Challenges for the oral delivery of macromolecules. Nat Rev Drug Discov 2:289–95.
- Gupta S, Jain A, Chakraborty M, et al. (2013). Oral delivery of therapeutic proteins and peptides: a review on recent developments. Drug Deliv 20:237–46.
- Hamano Y. (2011). Occurrence, biosynthesis, biodegradation, and industrial and medical applications of a naturally occurring ɛ-poly-l-lysine. Biosci Biotechnol Biochem 75:1226–33.
- Hiraki J, Ichikawa T, Ninomiya S, et al. (2003). Use of ADME studies to confirm the safety of epsilon-polylysine as a preservative in food. Regul Toxicol Pharmacol 37:328–40.
- Hyon SH, Nakajima N, Sugai H, et al. (2014). Low cytotoxic tissue adhesive based on oxidized dextran and epsilon-poly-l-lysine. J Biomed Mater Res A 102:2511–20.
- Jain D, Bajaj A, Athawale R, et al. (2016). Surface-coated PLA nanoparticles loaded with temozolomide for improved brain deposition and potential treatment of gliomas: development, characterization and in vivo studies. Drug Deliv 23:999–1016.
- Kammona O, Kiparissides C. (2012). Recent advances in nanocarrier-based mucosal delivery of biomolecules. J Control Release 161:781–94.
- Lee JS, Feijen J. (2012). Polymersomes for drug delivery: design, formation and characterization. J Control Release 161:473–83.
- Liang N, Sun S, Li X, et al. (2012). α-Tocopherol succinate-modified chitosan as a micellar delivery system for paclitaxel: preparation, characterization and in vitro/in vivo evaluations. Int J Pharm 423:480–8.
- Meng F, Zhong Z, Feijen J. (2009). Stimuli-responsive polymersomes for programmed drug delivery. Biomacromolecules 10:197–209.
- Mitragotri S, Burke PA, Langer R. (2014). Overcoming the challenges in administering biopharmaceuticals: formulation and delivery strategies. Nat Rev Drug Discov 13:655–72.
- Mo R, Jin X, Li N, et al. (2011). The mechanism of enhancement on oral absorption of paclitaxel by N-octyl-O-sulfate chitosan micelles. Biomaterials 32:4609–20.
- Morishita M, Peppas NA. (2006). Is the oral route possible for peptide and protein drug delivery? Drug Discov Today 11:905–10.
- Ohtake K, Maeno T, Ueda H, et al. (2003). Poly-l-arginine predominantly increases the paracellular permeability of hydrophilic macromolecules across rabbit nasal epithelium in vitro. Pharm Res 20:153–60.
- Pang Z, Feng L, Hua R, et al. (2010). Lactoferrin-conjugated biodegradable polymersome holding doxorubicin and tetrandrine for chemotherapy of glioma rats. Mol Pharm 7:1995–2005.
- Ranaldi G, Marigliano I, Vespignani I, et al. (2002). The effect of chitosan and other polycations on tight junction permeability in the human intestinal Caco-2 cell line(1). J Nutr Biochem 13:157–67.
- Rosenthal R, Gunzel D, Finger C, et al. (2012). The effect of chitosan on transcellular and paracellular mechanisms in the intestinal epithelial barrier. Biomaterials 33:2791–800.
- Sajeesh S, Bouchemal K, Sharma CP, et al. (2010). Surface-functionalized polymethacrylic acid based hydrogel microparticles for oral drug delivery. Eur J Pharm Biopharm 74:209–18.
- Sakuma S, Hayashi M, Akashi M. (2001). Design of nanoparticles composed of graft copolymers for oral peptide delivery. Adv Drug Deliv Rev 47:21–37.
- Salama NN, Eddington ND, Fasano A. (2006). Tight junction modulation and its relationship to drug delivery. Adv Drug Deliv Rev 58:15–28.
- Smart TP, Ryan AJ, Howse JR, et al. (2010). Homopolymer induced aggregation of poly(ethylene oxide)n-b-poly(butylene oxide)m polymersomes. Langmuir 26:7425–30.
- Tong J, Luxenhofer R, Yi X, et al. (2010). Protein modification with amphiphilic block copoly(2-oxazoline)s as a new platform for enhanced cellular delivery. Mol Pharm 7:984–92.
- Torchilin VP. (2014). Multifunctional, stimuli-sensitive nanoparticulate systems for drug delivery. Nat Rev Drug Discov 13:813–27.
- Tsujita T, Takaichi H, Takaku T, et al. (2006). Antiobesity action of epsilon-polylysine, a potent inhibitor of pancreatic lipase. J Lipid Res 47:1852–8.
- Wang S, Zhang S, Liu J, et al. (2014). pH- and reduction-responsive polymeric lipid vesicles for enhanced tumor cellular internalization and triggered drug release. ACS Appl Mater Interfaces 6:10706–13.
- Xu S, Olenyuk BZ, Okamoto CT, et al. (2013). Targeting receptor-mediated endocytotic pathways with nanoparticles: rationale and advances. Adv Drug Deliv Rev 65:121–38.
- Yoshida T, Nagasawa T. (2003). epsilon-Poly-l-lysine: microbial production, biodegradation and application potential. Appl Microbiol Biotechnol 62:21–6.