Abstract
This work aimed to achieve long-lasting delivery of radix ophiopogonis polysaccharide (ROP) by sucrose acetate isobutyrate (SAIB)-based in situ forming systems (ISFSs) alone or combined with mono-PEGylation of ROP. When the ‘90%SAIB/10% solvent’ system was used, the mean residence time (MRT) of ROP was prolonged by 4.3 5 ∼ 7.00 times and the initial release rate was reduced significantly. However, this system was only suitable for days-long sustained release of ROP in short-term therapy. As to the ‘SAIB/additives/solvent’ system containing mono-PEGylated ROP, the results indicated that SAIB/poly(d,l-lactide-co-glycolide) (PLGA)/N-methyl-2-pyrrolidone (NMP) was superior to SAIB/polylactic acid (PLA)/NMP and SAIB/PLA/ethanol in controlled release. Moreover, weeks- to months-long (16–60 d) smooth release of ROP could be achieved by varying the concentration (10–30%) and molecular weight (MW) of PLGA (10–50 kDa) or by employing a moderate MW of PEGylated ROP (∼20 or ∼30 kDa). With further increasing the conjugate MW to ∼40 kDa, the contribution of drug elimination to its plasma retention seemed to surpass that of the SAIB-based system, resulting in that the system no longer had an obvious influence on the in vivo behavior of the conjugate. Besides, the results of host response confirmed that with less solvent being used, the SAIB-based systems showed a higher biocompatibility than the PLGA-based systems, suggesting that they could be freely chosen in the prevention and/or cure of chronic diseases.
1. Introduction
Though macromolecule-based active agents, e.g. proteins, polypeptides, genes, and polysaccharides, are of great value in the treatment of a variety of diseases, unfavorable properties including low oral bioavailability, short plasma half-lives, and/or potential degradation in drug delivery, make their clinical applications quite challenging (Bao et al., Citation2016; Li et al., Citation2016; O’Neill et al., Citation2017; Wang & Burdick, Citation2017). Long-acting drug delivery systems are desired to improve patient compliance, reduce administration frequency, and minimize adverse effects. To date, the main strategies used in long-acting delivery of hydrophilic macromolecular drugs are (i) using sustained-release delivery systems and (ii) chemical modification of parent drugs.
Among sustained-release delivery systems, in situ forming systems (ISFSs) have become the focus of attention with the advantages of ease of administration, potential for local/site- specific delivery, potential for improved drug stability, and simplified manufacturing (Wright & Burgess, Citation2012). Sucrose acetate isobutyrate extended-release (SABER) systems are composed of sucrose acetate isobutyrate (SAIB), and one or more pharmaceutically acceptable solvents (ethanol, N-methyl-2-pyrrolidone, dimethyl sulfoxide, benzyl alcohol, benzyl benzoate (BB), etc.) as well as other excipients. SAIB is a fully acrylated derivative of sucrose with the characteristics of biodegradability and high viscosity. When SAIB is mixed with pharmaceutically acceptable solvents, the viscosity of the system can be dramatically reduced, making it possible to be injected through standard needles. Moreover, SAIB has been approved for oral consumption and is generally regarded as safe (GRAS) by the Food and Drug Administration (FDA) (Wright & Burgess, Citation2012; Jølck et al., Citation2014). As a non-polymeric liquid carrier, SAIB does not crystallize but exists as a highly viscous and hydrophobic liquid. When the system is injected, the diffusion of solvents out of the depot results in an increase in viscosity, thus decreasing the release rate of drugs trapped in depots and obtaining a desired extended release behavior. Significant advantages of the SABER system are a reduction in the amount of organic solvent, ease of use, lower production costs, and excellent sustained-release behavior that can be adjusted by additives from a few days to several months (Okumu et al., Citation2002). The most commonly used additives are biocompatible polymers, such as polylactic acid (PLA) and poly(d,l-lactide-co-glycolide) (PLGA) (Lu et al., Citation2008; Lin et al., Citation2012; Geng et al., Citation2016). However, there are few reports about the application of SABER systems in long-lasting delivery of bioactive polysaccharides.
The second strategy, i.e. the covalent derivatization of drug molecules, is a relatively novel approach, among which the well-established technique is PEGylation (i.e. the conjugation of polyethylene glycol (PEG) chain(s) to a drug molecule, thus resulting in a prolonged biological half-life, improved stability in vivo, and enhanced therapeutic efficacy of parent drugs) (Shahsavari et al., Citation2016; Haruta et al., Citation2017). Since 1990, several PEGylated products have been approved by the FDA, and at present, PEGylation has been established as a method of choice for improving the pharmacokinetics and pharmacodynamics of parenteral agents (Jevsevar et al., Citation2010; Shi et al., Citation2014). In our previous study, radix ophiopogonis polysaccharide (ROP) was successfully mono-PEGylated using a moderate coupling reaction between amino-terminated methoxy-PEG and excessive ROP with activated hydroxyls to markedly reduce the injection-administered frequency without sacrificing the therapeutic efficacy (Sun et al., Citation2013). While because of its relatively high peak value and steep plasma profile in a certain period as well as limited capability in sustained release, the combined use of ROP with sustained-release delivery systems (e.g. ISFSs, microspheres, and liposomes) is in demand.
ROP, a natural herb fructan with a weight-average molecular weight (MW) of 4.8 kDa (Xu et al., Citation2005), was found to play a critical role in anti-myocardial ischemia through protecting myocardial cells from damage induced by ischemia and promoting formation of microvessels in ischemic zones (Wang et al., Citation2010, Citation2012). However, as a highly hydrophilic agent, its low oral bioavailability (∼2%) and short plasma half-life (∼0.5 h) make it inconvenient in clinical application (Lin et al., Citation2005, Citation2006), which not only compromises its efficacy but necessitates frequent administration via injection.
To our best knowledge, there is no application of SABER systems alone or the coupling of PEGylation technology with SABER systems in long-acting delivery of bioactive polysaccharides. Therefore, sustained release of ROP was firstly investigated by the application of SABER systems with biocompatible solvents of ethanol, N-methyl-2-pyrrolidone (NMP), BB, and triacetin (TA). Further, to achieve a weeklong or even month-long steady delivery behavior, mono-PEGylated ROPs with different MW were employed in combination with the SABER systems and biocompatible additives (PLA and PLGA). The goal of this investigation was to determine the feasibility of SABER systems as well as the combination of PEGylation with SABER systems in long-lasting delivery of highly water-soluble polysaccharides.
2. Materials and methods
2.1. Materials and animals
ROP was prepared according to a previously reported method (Xu et al., Citation2005). Linear amino-terminated poly(ethylene glycol) methyl ether (mPEG-NH2) hydrochloride, with a MW of ∼20, ∼30 or ∼40 kDa, was purchased from Jenkem Technology Co., Ltd. (Beijing, PR China). SAIB was purchased from Jiangxi Tianyi Fragrance Flavors Co., Ltd. (Jiangxi, China). PLA with a MW of ∼10 kDa and free carboxylic acids at the ends of the polymeric backbone chain as well as poly (d, l-lactide-co-glycolide) copolymer (PLGA) with molar lactide to glycolide ratio being 50/50 and a MW of ∼10, ∼30, ∼40, or ∼50 kDa, and free carboxylic acids at the ends of the polymeric backbone chain, were purchased from Jinan Daigang Biomaterial Co., Ltd. (Shandong, China). Fluorescein isothiocyanate (FITC) was purchased from Sigma (St. Louis, MO). Extra dry dimethyl sulfoxide (DMSO) and N-methyl-2-pyrrolidone (NMP) were purchased from Acros Organics (Geel, Belgium). Ethanol, BB and TA were purchased from Sinopharm Chemical Reagent Ltd (Shanghai, China). All the other chemicals were of reagent grade and purchased from commercial sources.
Male Sprague-Dawley (SD) rats, weighing 240–280 g, were supplied by Lab Animal Center of Shanghai University of Traditional Chinese Medicine. All procedures of the animal experiments were approved by the Animal Ethical Experimentation Committee of Shanghai University of TCM according to the requirements of the National Act on the Use of Experimental Animals (PR China).
2.2. Preparation and characterization of FITC-labeled Mono-PEGylated ROPs (FMP-R)
With employment of a moderate coupling reaction between amino-terminated mPEG and hydroxyl-activated ROP, mono-PEGylated ROPs (MP-R) were synthesized, and the conjugates were characterized by anthrone-sulfuric acid colorimetry coupled high-performance gel permeation chromatography and 1H NMR (Sun et al., Citation2013). To determine their levels in plasma, the conjugates were pre-labeled with FITC before administration (Lin et al., Citation2006). The amounts of FITC labeled were determined by a Hitachi F-4500 fluorescence spectrophotometer (Hitachi, Tokyo, Japan) and were calculated in terms of the fluorescence intensity.
2.3. Preparation of SABER systems
Injectable SABER systems without additives were prepared by dispersing certain amounts of SAIB in various kinds of solvents (). Formulations with different MWs of PLGA (∼10, ∼30, ∼40, and ∼50 kDa) or PLA (∼10 kDa) as additives were prepared by mixing SAIB with PLGA or PLA solutions at 60 °C for a certain period with intermittent stirring until clear solutions were formed (). The drug-contained formulations were prepared by dispersing a designated portion of drugs in a certain volume of SAIB solutions in a water bath at 40 °C.
Table 1. SAIB-based in situ forming formulations and dosage regimen for FITC-labeled ROP (FROP) or mono-PEGylated ROPs (FMP-R) (n = 3).
Table 2. Pharmacokinetic parameters of Table FootnoteFROP released from 90%SAIB/10% solvent. (n = 3).
2.4. Rheological studies
The viscosity of ISFSs was investigated using a controlled stress mode of the plate/cone viscometer (Physica MCR-101, Anton Paar, Austria) at 20 and 37 °C, respectively. The flow properties of formulations were studied by using the plate and cone of 60 mm diameter/1 angle. The shear rate sweeps were measured whilst increasing the shear rate linearly from 10 to 500 s−1 over 2 min. Viscosity values were obtained by averaging recorded values.
2.5. Biocompatibility studies
Male SD rats were randomly divided into three groups, i.e. the control group, the ISFS ‘30%PLGA50k/NMP (w/w)’ group, and the ISFS ‘SAIB/PLGA50k/NMP (3.5:2:4.5, w/w/w)’ group. Under anesthesia, rats of ISFS groups were dosed with blank formulations, respectively. All the formulations were injected subcutaneously to the same position on the back of rats with 12-gauge needles at a dosage of 2 ml/kg. Rats that did not receive injection serviced as a control. Each group contained three rats and animals that administrated with formulations were sacrificed at day 1, 15, and 30-post injection, respectively. The subcutaneous tissues were harvested at the injection site and before being embedded in paraffin, the tissues were fixed in 10% formalin for a certain period. Cross sections were stained with hematoxylin and eosin (HE) stain to examine inflammation or any adverse effects. The image was taken using a microscope (Nikon, ECLIPSE Ci, Minato, Japan) equipped with a digital camera.
2.6. In vivo studies
Rats were dosed with different formulations according to a predetermined dosage regimen (). All the formulations were injected subcutaneously to the same position on the back of rats with 12-gauge needles. After injection, the pinpoint in the skin was sealed with medical glue (5 μl) in case the given formulation leaked out. Blood samples (∼400 μl) were collected into heparin-contained tubes by retro-orbital puncture at the specified time points and then immediately centrifuged at 4000 rpm for 10 min. The separated plasma was frozen at −20 °C for subsequent analysis. Each plasma sample (100 μl) was mixed with 40 μl of 1 M perchloric acid to precipitate plasma proteins. After centrifuging at 10,000 rpm for 2 min, 90 μl of the supernatant was transferred to another clean tube and neutralized using 30 μl of 1 M NaOH. After centrifuging again, a certain amount of the supernatant was taken out and diluted with phosphate-buffered saline (pH 7.4) to a detectable concentration before being determined spectrofluorimetrically at λex 495 nm and λem 515 nm. Pharmacokinetic software (DAS 2.1.1, Chinese Pharmacology Society, Beijing, China) was used to calculate major pharmacokinetic parameters by noncompartmental analysis. Values were reported as the mean ± standard deviation (SD). One-way analysis of variance was used for comparison among groups. A p value <.05 was considered to be statistically significant.
3. Results and discussion
3.1. Rheological studies
In order to have a better understanding on drug release and injectability of SABER formulations, rheological studies were carried out. The results showed (Refer Supporting Information Figure 1), except for 90% SAIB/10% BB, the relative viscosity of formulations increased with increasing hydrophobicity of the system, and significant reduction in viscosity was observed as the temperature changed from 20 to 37 °C. Because of the reason that formulations with lower viscosity are preferred in the application of ISFSs due to higher injectability, it is an effective way to preheat the formulations with high viscosity to ∼37 °C before injection to improve their syringeability. Due to the higher viscosity of TA, 90% SAIB/10% TA owned a higher viscosity than 90% SAIB/10% BB regardless of TA’s relatively low hydrophobicity. Thus, it can be easily concluded that the viscosity of the solvent might directly influence the final viscosity of the SABER systems. It is well known that with increasing the viscosity, drug’s diffusion rate decreased, thus formulations with high viscosity, like 90% SAIB/10% TA, might obtain a superior ability in controlling drug release.
Regarding the rheological results obtained for formulations with polymers as additives in SABER formulations (Refer Supporting Information Figure 2), relatively low viscosity was found at 37 °C. And, it was obvious that the formulation of SAIB/additives/solvent showed lower viscosity than 90% SAIB/10% solvent at both 20 and 37 °C. In terms of the viscosities of formulations with different polymer weight and different combinations, it was found that the viscosity of formulation decreased sharply as the ratio of solvent in the formulation increased regardless of the MW of PLGA. Compared to the formulation F (SAIB/PLGA30k/NMP (4:2:4, w/w/w)), the G (SAIB/PLGA40k/NMP (4:2:4, w/w/w)) showed a higher viscosity due to the higher MW of PLGA used. This might result in a further controlled drug release from the depot since the depot viscosity can affect not only the rate of solvent exchange in the initial release stage but also the diffusion of SAIB and drugs in the constant release period. Besides, it could be concluded that with the increasing of the ratio of polymer, the depot viscosity increased at both 20 and 37 °C regardless of different polymer weight and different combination of formulations. Regarding different additives, the results indicated that when polymers employed in SAIB/polymer/NMP (4:3:3, w/w/w) changed from PLGA to PLA, the system viscosity increased obviously. Also, it was found that as the solvent used in SAIB/PLA10k/solvent (4:3:3, w/w/w) changed from NMP to ethanol, the system’s viscosity decreased. As the ratio of SAIB used in the above three formulations was the same, NMP was thought to be the energetically favorable solvent of PLGA and can hinder polymer–polymer contacts. On the other hand, because of the predomination of the interaction between ethanol and PLA over polymer–polymer ones, the viscosity of SAIB/PLA10k/ethanol (4:3:3, w/w/w) decreased sharply when compared to SAIB/PLA10k/NMP (4:3:3, w/w/w). Considering the fact that viscosity can not only affect drug release from depots but also the injectability of formulations, a balance between the two parts needed to be made to achieve a desirable release behavior and a higher clinical compliance. In this study, because of the lowest viscosity, the combination of SAIB/PLGA/NMP was thought to be the most preferable one in clinical application of SABER systems.
3.2. Biocompatibility studies
As a representative formulation of PLGA-based ISFSs that have been reported to have a certain ability of long-lasting delivery of ROP (Shi et al., Citation2015), 30% PLGA50k/70% NMP (w/w) was chosen as a contrast to demonstrate the superior biocompatibility of SAIB/PLGA50k/NMP (3.5:2:4.5, w/w/w). In general, HE staining of the two formulations showed different degrees of cell edema, congestion, and inflammatory cell infiltration in the subcutaneous connective tissue at day 1 post injection, and the host response was reduced significantly at day 15 and day 30 post injection as only traces of the above abnormity could be observed (Refer Supporting Information Figure 3). It is known that once solvent-induced ISFSs are injected into an aqueous environment, the exchange of organic solvent of ISFSs with environmental non-solvent (i.e. water) will be triggered (Brodbeck et al., Citation1999; Solorio et al., Citation2015), and with the diffusion of solvent, tissues surrounding the depot might be stimulated. Compared to the normal control, cells from the ISFS groups after subcutaneous injection were loosely arranged, which was believed to be due to the diffusion of solvent from the depots to the environment. With complete exchange between the solvent and non-solvent, the stimulation of surrounding tissues caused by the diffusion and implanted biomaterials should be diminished. In this study, a significant reduction in inflammatory infiltrates and regular cell structures were found at day 15 and day 30 in both of the two groups, indicating that the inflammation caused by the ISFSs had been relieved. It was worth noting that the cell edema, congestion and inflammatory cell infiltration showed by the pathological sections for 30% PLGA50k/70% NMP (w/w) were more obvious than those for SAIB/PLGA50k/NMP (3.5:2:4.5, w/w/w) not only in day 1, but also in day 15. This should be attributed to the significantly higher level of solvent contained by the former. In clinical applications, in order to improve patient compliance and reduce adverse effects caused by stimulation, formulations with higher biocompatibility are needed. Therefore, although the host response caused by the two formulations can be diminished and relieved automatically, SABER systems provide a superior choice in terms of safety for long-lasting delivery of ROP compared to the PLGA-based ISFSs in which a large amount of organic solvent was used and a serious inflammatory response was found.
3.3. In vivo studies
3.3.1. SABER systems for parent ROP
The plasma level-time profiles of ROP achieved by 90% SAIB (w/w) in different solvents were shown in and the main pharmacokinetic parameters were listed in . In general, all the formulations studied resulted in a remarkably delayed and broadened plasmatic peak compared to the aqueous solution of ROP. For example, compared to the aqueous solution 2, these SAIB-based formulations only caused a 1.6–2.1 times higher maximum concentration in plasma (Cmax) after administration at a 4-fold higher dose. On the other hand, the time taken to reach Cmax (Tmax) was retarded from 0.33 h to 0.78–1.33 h, and more importantly, the plasma mean residence time (MRT) of ROP was prolonged significantly from 2.3 h to 10.1 ∼ 16.2 h. The release of drugs loaded in SAIB-based ISFSs was biphasic, characterized by ROP being released initially via the solvent exchange, followed by a constant release period (Lin et al., Citation2012). The SAIB/TA (9:1, w/w) combination achieved the most stable release of ROP from depots in the constant release period as the ratio values of Cmax/Cs (29.33 ± 19.38, Cs: the steady state concentration, is the average value of the plasma levels from 24 to 48 h) and Cmax/Cmin (54.71 ± 8.53, Cmin: the minimal plasma concentration determined after peak) were the lowest among all the formulations. However, the most preferable release of ROP from depots was obtained by SAIB/NMP (9:1, w/w) as the initial release rate of ROP from the system during 0 to Tmax after subcutaneous injection was the lowest (slope0–Tmax = 83.3, r2 = 0.9441). It was intriguing that regardless of the hydrophobicity of solvent, SAIB/ethanol (9:1, w/w) and SAIB/BB (9:1, w/w) caused a similar behavior of ROP in vivo, indicating that the viscosity of such formulations was more critical to drug release than the hydrophobicity of solvent used. In addition, the ratios of AUC0–12 h to AUC0–t (57.6–77.6%) clearly indicated that there was still a high rate of drug release from all of the SAIB/solvent (9:1, w/w) systems during the initial 12 h due to the time lag between the injection and the formation of depots. In terms of the data of bioavailability, the release of ROP from the depots was close to complete in about 72 h.
Figure 1. Plasma FROP concentration-time profiles achieved by the SAIB/solvent systems. (□) aqueous solution 1, (☆) aqueous solution 2, (◆) SAIB/ethanol (9:1, w/w), (▲) SAIB/NMP (9:1, w/w), (▼) SAIB/TA (9:1, w/w), (•) SAIB/BB (9:1, w/w). The dose for all the SAIB/solvent systems was 200 mg/kg, while those for the control aqueous solutions 1 and 2 were 15 and 50 mg/kg, respectively. FROP: FITC-labeled radix ophiopogonis polysaccharide; FITC: fluorescein isothiocyanate; SAIB: sucrose acetate isobutyrate; BB: benzyl benzoate; NMP: N-methyl-2-pyrrolidone; TA: triacetin.
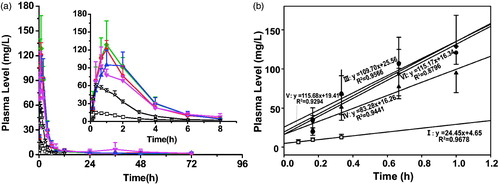
As to ISFSs, because of the reason that solvents employed generally have some contribution to drug release from the depots, it is vital to choose a ‘good’ solvent with proper solvent strength and aqueous miscibility to achieve a preferable sustained release of drugs. It is known that phase inversion can be triggered after the injection of ISFSs into aqueous environment, and due to the exchange of solvent-non-solvent, a high viscosity is obtained, thus the release rate of drugs from depots can be reduced by the denser structure and a long-lasting delivery of drugs can be achieved. In this study, a variety of solvents, including ethanol, NMP, TA, and BB, were investigated. The results showed that the initial release rate of ROP from the SAIB/solvent system (9:1, w/w) was reduced in the solvent order of TA > BB > ethanol > NMP. The release slopes during 0–Tmax after subcutaneous injection were 115.7, 115.2, 109.7, and 83.3, respectively, indicating that NMP was the most preferable solvent for the sustained delivery of ROP. And because of the broad plasma peak, half-day high and full ROP exposure, and 2–3-fold delayed Tmax, it was indicated that the ROP-loaded SAIB/solvent systems were suitable for short-term acute therapies of myocardial ischemia. However, it seemed impossible to obtain a week-long or month-long release of ROP with the employment of such systems as long-term precaution and therapy of myocardial ischemia. To combat this, mono-PEGylating ROP combined with release-modifying agents were tested.
3.3.2. SABER systems for PEGylated-ROP
To investigate the feasibility of SAIB-based ISFSs in combination with PEGylated ROPs and release-modifying agents for long-lasting delivery of ROP, the SAIB/PLGA/NMP system with a series of PLGA MW and 30 kDa PEG mono-PEGylated ROP (MP30k-R) were tested with the results shown in and . With the same drug loading (150 mg/ml), all the FMP30k-R-loaded formulations studied showed an ability of controlled release as the value of Cmax was reduced by 19.6–78.1% and the ratio values of Cmax/Cs and Cmax/Cmin were reduced by 42.1–74.9% and 39.4–81.1%, respectively, when compared to the aqueous solution 4 of FMP30k-R. Also, the initial release rate of FMP30k-R from SAIB/PLGA/NMP systems was significantly reduced as the release slopes from 0 to Tmax decreased from 128.5 to as low as 11.6. In terms of long-lasting steady delivery of FMP30k-R, the formulation with higher PLGA MW showed a more stable drug release. For example, the values of Cmax and Cmax/Cmin obtained by SAIB/PLGA40k/NMP (4:2:4, w/w/w) were 2.30- and 2.12-fold lower than those by SAIB/PLGA30k/NMP (4:2:4, w/w/w), respectively. In this study, two preferable profiles were achieved by the formulations SAIB/PLGA40k/NMP (4:2:4, w/w/w) and SAIB/PLGA50k/NMP (3.5:2:4.5, w/w/w) in terms of their even lower values of Cmax (52.6 and 58.0 mg/l vs. 59.5 mg/l) and Cmax/Cmin (7.58 and 8.36 vs. 9.01) as well as the lower initial release rates (slope0–Tmax =11.6 and 19.7 vs. 29.8) than those achieved by the aqueous solution 3 of FMP30k-R, which contained a 4-fold lower dose of FMP30k-R than the two formulations. When the MRT values obtained by the aqueous solutions of ROP and FMP30k-R were compared (2.3 h vs. 3.2 d), it was clear that PEGylation was an effective way to prolong the plasma exposure of ROP. Also, it was obvious that the MRT of FMP30k-R could be further significantly increased from 3.2 d to as long as 19.7 d by SABER systems. Overall, SABER systems with PEGylated ROP and PLGA as drug and release-modifying agent, respectively, provide a new choice for long-lasting delivery of ROP by ISFSs. Also, the results showed that the addition of PLGA makes it feasible to decrease the initial burst release of drugs from depot.
Figure 2. Plasma FMP30k-R concentration-time profiles achieved by the SAIB/PLGA(PLA)/NMP systems (a, profiles achieved by the SAIB/PLGA/NMP systems with different molecular weights of PLGA; b, profiles achieved by the SAIB/PLGA10k/NMP systems with different ratio; c, profiles achieved by the different SAIB/additive/solvent systems; and d, profiles achieved by the SAIB/PLGA40k/NMP systems with different ratio.). (□) aqueous solution 3, (☆) aqueous solution 4, (◆) SAIB/PLGA10k/NMP (5:2:3, w/w/w), (•) SAIB/PLGA30k/NMP (4:2:4, w/w/w), (▲)SAIB/PLGA40k/NMP (4:2:4, w/w/w), (▼) SAIB/PLGA50k/NMP (3.5:2:4.5, w/w/w), (⬡) SAIB/PLGA10k/NMP (4.5:2.5:3, w/w/w), (※) SAIB/PLGA10k/NMP (4:3:3, w/w/w), (^) SAIB/PLA10k/NMP (4:3:3, w/w/w), (△)SAIB/PLA10k/Ethanol (4:3:3, w/w/w), (◃) SAIB/PLGA40k/NMP (4.5:1.5:4, w/w/w), (▷)SAIB/PLGA40k/NMP (5:1:4, w/w/w). The dose for all the SAIB-based systems and aqueous solution 4 was 300 mg/kg, while that for the control aqueous solution 3 was 75 mg/kg. FMP30k-R: FITC-labeled 30 kDa PEG mono-modified ROP; SAIB: sucrose acetate isobutyrate; FITC: fluorescein isothiocyanate; PEG: polyethylene glycol; ROP: radix ophiopogonis polysaccharide; PLGA: poly(d,l-lactide-co-glycolide); PLA: polylactic acid; NMP: N-methyl-2- pyrrolidone; 10k, 10 kDa; 30k, 30 kDa; 40k, 40 kDa; and 50k, 50 kDa.
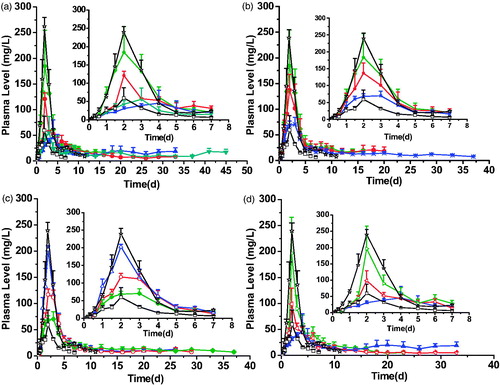
Table 3. Pharmacokinetic parameters of FMP-R released from SAIB/additives/solvent (n = 3).
Focuses needed to be paid on SAIB/PLGA10k/NMP (5:2:3, w/w/w) as it showed an unsatisfied initial release rate (slope0–Tmax =106.0, r2 =0.9780) and initial cumulative release (AUC0–5d/AUC0–t =66.0%) of FMP30k-R (). It is known that with PLGA being employed in ISFSs as a release-modifying agent, a semisolid shell can be formed on the surface of the depot as soon as the system is injected into aqueous environment. PLGA coatings on SAIB provides mechanically stable shells as well as a diffusion barrier for the encapsulated drugs, thus the systems can restrict mass transfer into and out of the interior of depots and obtain a steady exchange between solvent-non-solvent as well as sustained release of drugs from depots (Wang et al., Citation2007; Lin et al., Citation2012). In this study, to obtain a remarkably delayed and broadened plasmatic FMP30k-R peak by the addition of PLGA10k, which had a relatively faster biodegradable rate, lower viscosity, and faster dissolution rate in NMP than PLGAs with higher MW, three levels of PLGA10k (20, 25, and 30%) were investigated with the ratio of NMP staying the same (30%) in SAIB/PLGA10k/NMP (w/w/w). The results showed that the effect of PLGA levels in SABER systems was significant ( and ). As a whole, the most satisfactory release behavior was achieved by SAIB/PLGA10k/NMP (4:3:3, w/w/w) as the value of Cmax achieved was even comparable to that achieved by the aqueous solution 3 of FMP30k-R with a 4-fold lower dose. With the PLGA10k level increasing from 20 to 30%, linear decreases in Cmax (192.7, 137.2, and 76.1 mg/l, r2 =0.9993) and AUC0–5d (453.8, 345.4, and 226.3 mg/l*d, r2 =0.9993) were observed and the MRT appeared to be prolonged from 5.0 d to 13.1 d. A nearly zero-order release of FMP30k-R with a pretty low Cmax/Cs (5.13 ± 0.49) was observed as the PLGA10k level increased to 30% and the plasma sustained time of FMP30k-R was extended by 5.29 folds, from 7 d to 37 d compared to the aqueous solution 3 of FMP30k-R.
Investigations were further carried out to find whether it was applicable to obtain a nearly zero-order release of FMP30k-R by altering the kind of release-modifying agents and solvents with the formulation ‘SAIB/Release-modifying agent/Solvent (4:3:3, w/w/w)’ being employed. The results were shown in . In general, all the formulations studied showed a certain degree of long-lasting delivery of FMP30k-R in terms of the relatively lower value of Cmax (76.1–202.9 mg/l vs. 239.6 mg/l) and longer MRT (6.6–13.1 d vs. 3.2 d) compared to the aqueous solution 4 of FMP30k-R with the same dose. As to the formulations ‘SAIB/PLGA10k/NMP (4:3:3, w/w/w)’ and ‘SAIB/PLA10k/NMP (4:3:3, w/w/w)’, it was noticeable that a relatively stable release was achieved by the former regardless of its lower viscosity. This is believed to be mainly caused by the higher hydrophobicity of PLA10k and thus relatively slower formation of the semisolid shell that is conducive to controlling the drug release (Dong et al., Citation2011; Lin et al., Citation2012). The results indicate that not only viscosity but also hydrophobicity plays a critical role in drug release from ISFSs. It’s also confirmed that NMP is the energetically favorable solvent of PLGA. The formation of a dense polymer shell on the surface of the depot is thought to play a critical role in retarding drug release because of the reason that limited water miscibility can slow the dynamics of phase inversion and solidification process and thus can influence the diffusion rate that drug molecules take as they leave the implant (Lin et al., Citation2012; Chen & Singh, Citation2005). For formulations with different solvents, ‘SAIB/PLA10k/NMP (4:3:3, w/w/w)’ achieved a more preferable release profile than ‘SAIB/PLA10k/Ethanol (4:3:3, w/w/w)’ in terms of a lower initial release rate (slope0–Tmax =114.1 vs. 64.5) and a more stable release in the constant release period (Cmax/Cs =13.2 vs. 10.9). From these cases, it can be concluded that different release behaviors of FMP30k-R could be achieved by varying the combination of release-modifying agent and solvent in SABER systems, thus making it possible to obtain several formulations with nearly zero-order drug release to meet the demand of clinical applications in the long-acting delivery of bioactive polysaccharides.
For SABER systems with polymers like PLGA and PLA as release-modifying agents, SAIB serves as a matrix to reserve the drug and the precipitation of polymers, triggered by solvent-non-solvent exchange between SAIB and the aqueous injection site after administration, makes the matrix be enclosed and the path that drug molecules take as they leave the depot be plugged, and upon activation, the drug diffuses through the membrane at a finite and controllable rate (Chen & Singh, Citation2005; Lu et al., Citation2008; Lin et al., Citation2012). Therefore, it is vital to choose a ‘good’ solvent as well as an optimal biocompatible polymer with desirable hydrophobicity to achieve expected controlled release of drugs. By altering solvents and polymers used in SABER systems, the characteristics of the formulation (such as viscosity, water miscibility, and rate of solvent exchange) can be modified, and different drug release profiles can be obtained, thus making this drug delivery technology flexible in formulation design to achieve specific aims and/or fit with drugs with specific properties.
To confirm the applicability of high MW PLGAs as release-modifying agent, studies were carried out and the results, shown in , clearly indicated that significantly different release behaviors could be achieved by changing the ratio of PLGA40k from 10 to 20%. The initial release rate was decreased with increasing PLGA’s concentration, e.g. the release slopes of formulations decreased sharply from 128.5 to 11.6 and the initial cumulative release (AUC0–5d/AUC0–t) decreased obviously from 57.0 to 24.3%. With the PLGA40k level changing from 10 to 20%, linear decreases in Cmax (196.6, 98.5, and 52.6 mg/l, r2 =0.9581) and AUC0–5d (349.8, 219.8, and 145.7 mg/l*d, r2 =0.9755) were also observed with a linear increase in MRT (6.2, 9.7, and15.2 d, r2 =0.9860). The steadiest and slowest release of FMP30k-R was achieved by SAIB/PLGA40k/NMP (4:2:4, w/w/w) consisting of the lowest initial release rate (slope0–Tmax =11.6), the most stable release in the constant release period (Cmax/Cs =3.27 ± 1.91, Cmax/Cmin=7.58 ± 6.60), and the most significantly prolonged MRT (15.2 ± 3.1 d) ( and ). What’s more, the values of Cmax, Cmax/Cs, and Cmax/Cmin obtained by SAIB/PLGA40k/NMP (4:2:4, w/w/w) were even lower than those of the aqueous solution 3 of FMP30k-R with a 4-fold lower dose, indicating that a superior prolonged release could be achieved by the employment of a high MW PLGA at relatively low concentration in SABER systems. As to formulations with different MW of PLGAs as additives, both SAIB/PLGA40k/NMP (4:2:4, w/w/w) and SAIB/PLGA10k/NMP (4:3:3, w/w/w) obtained a nearly zero-order and comparable release of FMP30k-R from SABER systems (), while it was noteworthy that the sustained release of FMP30k-R from the latter was obtained at a cost of higher PLGA concentration.
Not only additives but also drugs used in ISFSs can affect drug release from depots (Brodbeck et al., Citation1999; Zhang et al., Citation2015). Therefore, mono-PEGylated-ROPs with MWs of ∼20, ∼30, and ∼40 kDa, respectively, were used for the first time to clarify the effect of PEG MW on drug release from SABER systems. The results were shown in and . Compared to their respectively aqueous solutions, the SABER systems with a 4-fold higher dose achieved a comparable Cmax for FMP20k-R and FMP30k-R, together with 6- and 7-fold increases in MRT, respectively; however, a 3-fold higher Cmax was observed for FMP40k-R although its MRT was prolonged 3.8-fold by the SABER system. That is to say that the controlled-release effect of the SABER system decreased sharply when the conjugate MW increased to ∼40 kDa. After subcutaneous administration of drugs loaded in SABER systems, there are three critical processes that together affect the ultimate plasma profile: (i) release from depots, (ii) absorption into the blood circulation; and (iii) plasma elimination of drugs. The release process is determined by both the SABER system and drug properties (especially the – MW and polarity) while the other two depend on drug properties only. With the MW of MP-R increasing from below to above the filtration thresholds of blood vessels and glomeruli, the influence of the processes (ii) and (iii) on its plasma profile will increase and might become the rate-limiting ones, resulting in the weakening of influence of SABER systems. This should be just the case for FMP40k-R, whose MW is markedly higher than the reported glomerular filtration threshold for PEG (∼30 kDa) (Yamaoka et al., Citation1994; Greenwald et al., Citation1996). A dilemma for PEGylated drugs is that the stronger plasma retention ability they have, the higher the extent of their accumulation in vivo is (Yamaoka et al., Citation1994). The results obtained in this study might provide a solution to the above dilemma. Namely, with the reasonable combination of SABER systems with PEGylated drugs having a MW around the glomerular filtration threshold, anticipant long-lasting and steady plasma exposure of drugs could be achieved without bringing the in vivo accumulation problem.
Figure 3. Plasma FMP-R concentration-time profiles achieved by the SAIB/PLGA50k/NMP systems loaded with different molecular weight FMP-R. (□) Aqueous solution 5 (FMP20k-R), (^) Aqueous solution 3 (FMP30k-R), (☆) aqueous solution 4 (FMP30k-R), (△) aqueous solution 6 (FMP40k-R), (▪) SAIB/PLGA50k/NMP (3.5:2:4.5, w/w/w, FMP20k-R), (•) SAIB/PLGA50k/NMP (3.5:2:4.5, w/w/w,FMP30k-R), (▲) SAIB/PLGA50k/ NMP (3.5:2:4.5, w/w/w, FMP40k-R). The dose for all the SAIB-based systems and aqueous solution 4 was 300 mg/kg, while that for the control aqueous solutions 3, 5, and 6 was 75 mg/kg. FMP20k-R: FITC-labeled 20 kDa PEG mono-modified ROP; FMP30k-R: FITC-labeled 30 kDa PEG mono-modified ROP; FMP40k-R: FITC-labeled 40 kDa PEG mono-modified ROP; FITC: fluorescein isothiocyanate; PEG: polyethylene glycol; ROP: radix ophiopogonis polysaccharide; SAIB: sucrose acetate isobutyrate; PLGA: poly(d,l-lactide-co- glycolide); NMP: N-methyl-2-pyrrolidone; 50k, 50 kDa.
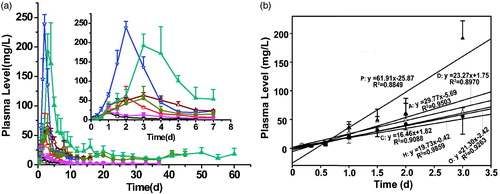
Overall, release behaviors of MP-R from SABER systems can be tailored effectively by altering type-and-level combinations of solvent and additives as well as the MW of MP-R.
3.4. Some general formulation and application principles
Nowadays, with the emerging developments in natural products, a number of polysaccharides have been approved to be effective in anti-tumor (Sun et al., Citation2015; Fan et al., Citation2017), immunoregulation (Auyeung et al., Citation2016; Liu et al., Citation2016), and other disorders (Hu et al., Citation2016; Li et al., Citation2016; Jiao et al., Citation2017). However, their injection-only nature with short half-life makes their clinical applications inconvenient. Therefore, it is urgent to investigate a suitable dosage form of bioactive polysaccharides with poor pharmacokinetic properties.
To meet the demand of short-term and high exposure of drugs, the SAIB/solvent systems might be a choice because of their gentle and flexible controlled-release ability. Drug release behaviors could be adjusted simply by changing the type and level of solvent in this system. For example, all the four formulations presented in this study prolonged the MRT of ROP by 4.35–7.00 times and an approximately 3 d long sustained release was achieved.
When week-long or even month-long drug release is needed for the prevention and/or cure of cancer and other chronic diseases (Parent et al., Citation2013; Chu et al., Citation2016; Fernando et al., Citation2016; Han et al., Citation2016), other pharmaceutical technologies could be combined with SABER systems. In this study, the combination of release-modifying agent-contained SABER systems with PEGylation was tested for the first time and a nearly month-long release of ROP was achieved either by improving the concentration and MW of additives or by employing a moderate MW of PEGylated drugs. For example, with a higher MW of PLGA or higher level of PLGA being used, a superior release behavior of FMP30k-R was achieved by SAIB/PLGA40k/NMP (4:2:4, w/w/w) compared to SAIB/PLGA30k/NMP (4:2:4, w/w/w) (MRT: 9.77 vs. 5.03 d, Cmax/Cs: 3.27 vs. 6.93, Cmax/Cmin: 7.58 vs. 24.26), and SAIB/PLGA40k/NMP (5:1:4, w/w/w) (MRT: 9.77 vs. 6.16 d, Cmax/Cs: 3.27 vs. 9.57, Cmax/Cmin: 7.58 vs. 49.20). Similarly, as the MW of PEGylated-ROPs loaded in SAIB/PLGA50k/NMP (3.5:2:4.5, w/w/w) increased from ∼20 to ∼30 kDa, the value of MRT was prolonged from 13.2 to 19.7 d with the decrease of Cmax/Cs from 7.52 to 5.11 and Cmax/Cmin from 12.56 to 8.36. Overall, formulations with both a relatively lower initial release rate and lower ratio values of Cmax/Cs and Cmax/Cmin are preferable for week-long or month-long sustained delivery of polysaccharides, while those with higher initial release rates and peak values of plasma concentration are promising for acute therapies.
4. Conclusion
The release behaviors of drugs from SABER systems can be tailored effectively by altering type-and-level combinations of solvent and additives as well as the MW of drugs, thus making this drug delivery technology flexible in formulation design to achieve specific aims and/or fit with drugs with specific properties. With low level of organic solvent being used, SABER systems provide a superior choice in terms of safety for long-lasting delivery of drugs compared to PLGA-based ISFSs. The application of SABER systems in long-lasting delivery of ROP could not only obtain several days long sustained release of ROP but also weeks or a month-long sustained release of mono-PEGylated ROPs with additives being employed in formulations. Moreover, with the reasonable combination of SABER systems with PEGylated drugs having a MW around the glomerular filtration threshold, superior long-lasting and steady plasma exposure of drugs would be achieved without bringing the problem of accumulation in vivo.
IDRD_Lin_et_al_Supplemental_Content.docx
Download MS Word (2 MB)Disclosure statement
The authors report no conflicts of interest in this work.
Additional information
Funding
References
- Auyeung KK, Han QB, Ko JK. (2016). Astragalus membranaceus: a review of its protection against inflammation and gastrointestinal cancers. Am J Chin Med 44:1–22.
- Bao L, Cai X, Wang J, et al. (2016). Anti-fatigue effects of small molecule oligopeptides isolated from Panax ginseng C. A. Meyer in mice. Nutrients 8:807–17.
- Brodbeck KJ, DesNoyer JR, McHugh AJ. (1999). Phase inversion dynamics of PLGA solutions related to drug delivery. Part II. The role of solution thermodynamics and bath-side mass transfer. J Control Release 62:333–44.
- Chen S, Singh J. (2005). Controlled delivery of testosterone from smart polymer solution based systems: in vitro evaluation. Int J Pharm 295:183–90.
- Chu X, Liu XJ, Qiu JM, et al. (2016). Effects of Astragalus and Codonopsis pilosula polysaccharides on alveolar macrophage phagocytosis and inflammation in chronic obstructive pulmonary disease mice exposed to PM2.5. Environ Toxicol Pharmacol 48:76–84.
- Dong S, Wang S, Zheng C, et al. (2011). An in situ-forming, solid lipid/PLGA hybrid implant for long-acting antipsychotics. Soft Matter 7:5873–8.
- Fan S, Zhang J, Nie W, et al. (2017). Antitumor effects of polysaccharide from Sargassum fusiforme against human hepatocellular carcinoma HepG2 cells. Food Chem Toxicol 102:53–62.
- Fernando IP, Nah JW, Jeon YJ. (2016). Potential anti-inflammatory natural products from marine algae. Environ Toxicol Pharmacol 48:22–30.
- Geng Z, Luo X, Zhang Z, et al. (2016). Study of an injectable in situ forming gel for sustained-release of Ivermectin in vitro and in vivo. Int J Biol Macromol 85:271–6.
- Greenwald RB, Gilbert CW, Pendri A, et al. (1996). Drug delivery systems: water soluble taxol 2’-poly(ethylene glycol) ester prodrugs-design and in vivo effectiveness. J Med Chem 39:424–31.
- Han SY, Hu MH, Qi GY, et al. (2016). Polysaccharides from polygonatum inhibit the proliferation of prostate cancer-associated fibroblasts. Asian Pac J Cancer Prev 17:3829–33.
- Haruta K, Otaki N, Nagamine M, et al. (2017). A novel PEGylation method for improving the pharmacokinetic properties of anti-interleukin-17A RNA aptamers. Nucleic Acid Ther 27:36–44.
- Hu Y, Sheng Y, Yu M, et al. (2016). Antioxidant activity of Inonotus obliquus polysaccharide and its amelioration for chronic pancreatitis in mice. Int J Biol Macromol 87:348–56.
- Jevsevar S, Kunstelj M, Porekar VG. (2010). PEGylation of therapeutic proteins. Biotechnol J 5:113–28.
- Jiao Y, Wang X, Jiang X, et al. (2017). Antidiabetic effects of Morus alba fruit polysaccharides on high-fat diet- and streptozotocin-induced type 2 diabetes in rats. J Ethnopharmacol 199:119–27.
- Jølck RI, Binderup T, Hansen AE, et al. (2014). Injectable colloidal gold in a sucrose acetate isobutyrate gelating matrix with potential use in radiation therapy. Adv Healthc Mater 3:1680–7.
- Li K, Zhuo C, Teng C, et al. (2016). Effects of Ganoderma lucidum polysaccharides on chronic pancreatitis and intestinal microbiota in mice. Int J Biol Macromol 93:904–12.
- Li Z, Li H, Wang C, et al. (2016). Sodium dodecyl sulfate/β-cyclodextrin vesicles embedded in chitosan gel for insulin delivery with pH-selective release. Acta Pharm Sin B 6:344–51.
- Lin X, Xu DS, Feng Y, et al. (2005). Determination of Ophiopogon japonicus polysaccharide in plasma by HPLC with modified postcolumn fluorescence derivatization. Anal Biochem 342:179–85.
- Lin X, Xu DS, Feng Y, et al. (2006). Release-controlling absorption enhancement of enterally administered Ophiopogon japonicus polysaccharide by sodium caprate in rats. J Pharm Sci 95:2534–42.
- Lin X, Yang S, Gou J, et al. (2012). A novel risperidone-loaded SAIB-PLGA mixture matrix depot with a reduced burst release: effects of solvents and PLGA on drug release behaviors in vitro/in vivo. J Mater Sci Mater Med 23:443–55.
- Liu Z, Xing J, Zheng S, et al. (2016). Ganoderma lucidum polysaccharides encapsulated in liposome as an adjuvant to promote Th1-bias immune response. Carbohydr Polym 142:141–8.
- Lu Y, Tang X, Cui Y, et al. (2008). In vivo evaluation of risperidone-SAIB in situ system as a sustained release delivery system in rats. Eur J Pharm Biopharm 68:422–9.
- Okumu FW, Dao l. N, Fielder PJ, et al. (2002). Sustained delivery of human growth hormone from a novel gel system: SABER. Biomaterials 23:4353–8.
- O’Neill HS, O’Sullivan J, Porteous N, et al. (2017). A collagen cardiac patch incorporating alginate microparticles permits the controlled release of HGF and IGF-1 to enhance cardiac stem cell migration and proliferation. J Tissue Eng Regen Med. DOI:10.1002/term.2392.
- Parent M, Nouvel C, Koerber M, et al. (2013). PLGA in situ implants formed by phase inversion: critical physicochemical parameters to modulate drug release. J Control Release 172:292–304.
- Shahsavari A, Azad M, Mobarra N, et al. (2016). Calprotectin pegylation enhanced its physical and structural properties. Protein J 35:363–70.
- Shi X, Lin X, Yao C, et al. (2015). Injectable long-acting in situ forming systems for radix ophiopogonis polysaccharide. Int J Biol Macromol 72:553–9.
- Shi X, Lin X, Zheng X, et al. (2014). Injectable long-acting systems for radix ophiopogonis polysaccharide based on mono-PEGylation and in situ formation of a PLGA depot. Int J Nanomedicine 9:5555–63.
- Solorio L, Sundarapandiyan D, Olear A, et al. (2015). The effect of additives on the behavior of phase sensitive in situ forming implants. J Pharm Sci 104:3471–80.
- Sun G, Lin X, Shen L, et al. (2013). Mono-PEGylated radix ophiopogonis polysaccharide for the treatment of myocardial ischemia. Eur J Pharm Sci 49:629–36.
- Sun M, Zhao W, Xie Q, et al. (2015). Lentinan reduces tumor progression by enhancing gemcitabine chemotherapy in urothelial bladder cancer. Surg Oncol 24:28–34.
- Wang LL, Burdick JA. (2017). Engineered hydrogels for local and sustained delivery of RNA-interference therapies. Adv Healthc Mater 6:1601041.
- Wang S, Zhang Z, Lin X, et al. (2010). A polysaccharide, MDG-1, induces S1P1 and bFGF expression and augments survival and angiogenesis in the ischemic heart. Glycobiology 20:473–84.
- Wang X, Wenk E, Hu X, et al. (2007). Silk coatings on PLGA and alginate microspheres for protein delivery. Biomaterials 28:4161–9.
- Wang S, Lin X, Wang LY, et al. (2012). A polysaccharides MDG-1 augments survival in the ischemic heart by inducing S1P release and S1P1 expression. Int J Biol Macromol 50:734–40.
- Wright JC, Burgess DJ, eds. (2012) Long acting injections and implants, advances in delivery science and technology. New York (NY): Springer.
- Xu D, Feng Y, Lin X, et al. (2005). Isolation, purification and structural analysis of a polysaccharide MDG-1 from Ophiopogon japonicas. Acta Pharmaceutica Sinica 40:636–9.
- Yamaoka T, Tabata Y, Ikada Y. (1994). Distribution and tissue uptake of poly(ethylene glycol) with different molecular weights after intravenous administration to mice. J Pharm Sci 83:601–6.
- Zhang K, Shi X, Lin X, et al. (2015). Poloxamer-based in situ hydrogels for controlled delivery of hydrophilic macromolecules after intramuscular injection in rats. Drug Deliv 22:375–82.