Abstract
Glioblastoma (GBM) is the most common and lethal primary brain tumor which is highly resistant to conventional radiotherapy and chemotherapy, and cannot be effectively controlled by surgical resection. Due to inevitable recurrence of GBM, it remains essentially incurable with a median overall survival of less than 18 months after diagnosis. A great challenge in current therapies lies in the abrogated delivery of most of the chemotherapeutic agents to the tumor location in the presence of blood-brain barrier (BBB) and blood-brain tumor barrier (BBTB). These protective barriers serve as a selectively permeable hurdle reducing the efficacy of anti-tumor drugs in GBM therapy. This work systematically gives a comprehensive review on: (i) the characteristics of the BBB and the BBTB, (ii) the influence of BBB/BBTB on drug delivery and the screening strategy of small-molecule chemotherapeutic agents with promising BBB/BBTB-permeable potential, (iii) the strategies to overcome the BBB/BBTB as well as the techniques which can lead to transient BBB/BBTB opening or disruption allowing for improving BBB/BBTB-penetration of drugs. It is hoped that this review provide practical guidance for the future development of small BBB/BBTB-permeable agents against GBM as well as approaches enhancing drug delivery across the BBB/BBTB to GBM.
1. Introduction
1.1. Glioblastoma (GBM)
Glioblastoma multiforme (GBM) has been designated by the World Health Organization (WHO) as grade IV glioma or grade IV astrocytoma, and is the most common and lethal primary brain tumor, which is associated with its high resistance to conventional radiotherapy and chemotherapy as well as inevitable recurrence. Due to the infiltrative growth behavior of GBM, complete surgical resection is insufficient to control tumors, resulting in high rates of recurrence which is the primary cause of death. Generally, an annual incidence of 0.6–3.7 per 100,000 individuals for GBM is estimated worldwide (Ostrom, Bauchet, et al., Citation2014). Despite aggressive surgical resection with postoperative radiotherapy and concomitant chemotherapy, the median overall survival of GBM patients remains 12–15 months following diagnosis (Ostrom, Gittleman, et al., Citation2014), with less than 5% of people surviving longer than 5 years (Adeberg et al., Citation2014).
Despite continuous effort directly toward developing new therapeutic approaches as well as novel molecularly tumor-targeted chemotherapeutic agents for better control of malignant glioblastoma, GBM remains essentially incurable attribute to its cellular heterogeneity and drug-resistance nature. Currently, the standard therapeutic regimen for GBM involves maximal feasible surgical resection, followed by postoperative radiotherapy with concomitant and adjuvant temozolomide (TMZ) (Cantanhede & de Oliveira, Citation2017; Lim et al., Citation2018). Numerous evidences from clinical trails have demonstrated that, the standard treatment is able to significantly extend the time to recurrence and median survival of the patients who were under 70 years old with newly diagnosed GBM (Davis, Citation2016). In addition, a number of active chemotherapeutic drugs including the nitrosourea compounds carmustine (BCNU) and lomustine (CCNU) as well as the platinum agents cisplatin and carboplatin, have been considered for GBM treatment as well. Especially, cisplatin and carboplatin were the drugs that have been given as first line agents in patients with GBM in the past. However, the most widely used chemotherapeutic drug is temozolomide (TMZ), an oral small-molecule alkylating agent, which is an imidazotetrazine derivative of dacarbazine. It exhibits cytotoxic effect via preferentially O6-methylation of guanine in DNA, leading to G2/M-phase arrest in tumor cells (Pourgholi et al., Citation2016). TMZ is capable of penetrating into the central nervous system (CNS) and has 96–100% bioavailability (Davis, Citation2016). In 1999, TMZ was first approved by the U.S. Food and Drug Administration (FDA) for the therapeutic agent against maglinant gliomas. According to preliminary clinical studies, serving as a single agent, TMZ displayed a good response rate of 11% and stable disease in 47% of GBM patients. Further, TMZ was found to be continuously effective with a response rate of 23.8% in patients with GBM in clinical phase II studies (Pourgholi et al., Citation2016; Cantanhede & de Oliveira, Citation2017). In comparison to several chemotherapeutic agents, such as BCNU, CCNU, and platinum drugs, TMZ was the best option that improved the median survival time in GBM patients (Hardell et al., Citation2009). Moreover, TMZ chemotherapy was commonly included in combination treatment with radiotheapy. In a large phase III trail involving 575 patients with GBM, compared with radiotherapy alone, concurrent radiation and TMZ treatment improved the median overall survival from 12.1 to 14.6 months while the 2-year survival rate was increased from 10% to 27% (Khosla, Citation2016; Lim et al., Citation2018). The concomitant radiotherapy with TMZ chemotherapy have many advantages including minimal additional toxicity (Lim et al., Citation2018) and improved radio-sensitivity of tumor cells (Chamberlain et al., Citation2007). Furthermore, radiotherapy has been reported not only to facilitate spontaneous conversion of TMZ into its active component in the brain, but also to confer TMZ with a good permeability across the blood-brain barrier (BBB) (Lim et al., Citation2018).
The accumulation of TMZ in tumors is still disappointing, although it is capable of penetrating the BBB. TMZ has been found to be one of the substrates of P-glycoprotein (P-gp), an important efflux pump which locates on the apical membrane side of endothelial cells forming BBB and serves as a maintainer of the integrity and the polarity of BBB (Goldwirt et al., Citation2014). Due to the presence of P-gp at the BBB, only 20% of TMZ regarding a systemic dose is able to enter the cerebral parenchyma (Ostermann et al., Citation2004). Additionally, BBB also accounts for the limited efficacy of other chemotherapeutic agents in GBM, such as etoposide, irinotecan, vincristine, and cisplatin. Undeniably, BBB and blood-brain tumor barrier (BBTB) greatly contribute to the therapy resistance in GBM. Therefore, more and more efforts deserve to be devoted to developing novel therapeutic strategies to surpass or to bypass these obstacles, in order to improve the GBM treatment.
1.2. BBB
Despite ongoing development of novel chemotherapeutic agents against GBM, achieving effective drug delivery into the tumors remains a big challenge, which results in a poor prognosis for this malignant cancer. The key hurdle to effective treatments is the BBB. The BBB serves as an anatomical and physiological barrier which is crucial for protecting the CNS from the potentially harmful substances, such as pathogens and neurotoxic compounds circulating in the blood stream. In addition to its protective function, the BBB modulates the metabolic exchanges between the brain parenchyma and blood for maintaining CNS fluid homeostasis, which is essential for normal function of the brain. In essence, the BBB is composed of non-fenestrated brain endothelial cells (BECs) of the capillary wall, which is surrounded with pericytes, astrocytes, perivascular neurons, a basal membrane, and extracelluar matrix, forming the highly organized neurovascular unit () (Abbott et al., Citation2006; Deeken & Löscher, Citation2007; Winkler et al., Citation2011). Pericytes share a basal lamina with endothelial cells and play a critical role in the maintenance of the structural integrity of the BBB (Obermeier et al., Citation2013). The basement membrane, which ensheaths both pericytes and endothelial cells, is surrounded with the plasma membranes of astrocytic end-feet, providing biochemical support to the endothelium and contributing to the barrier properties of the BBB (Abbott et al., Citation2006; Alvarez et al., Citation2013). Furthermore, astrocytes are contiguous with neurons, which allows for the communication between brain vasculature and neuronal metabolic demand (Winkler et al., Citation2011).
Figure 1. Schematic representation of (A) neurovascular unit; (B) paracellular transport pathway and transcellular transport pathway of BBB; (C) tight junction (TJ) associated components.
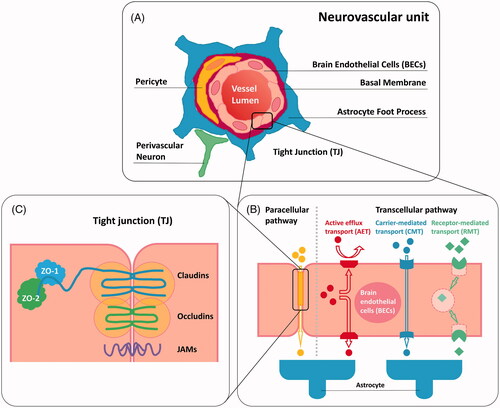
The human brain contains over 100 billion capillaries with a total length of ∼400 miles, which endows the BBB with a complete surface area of 20 m2 (Pardridge, Citation2005). Generally, small or gaseous molecules such as H2O, O2, and CO2 are able to freely traverse the BBB via passive diffusion, as can some small highly liposoluble/hydrophobic molecules (Wong et al., Citation2013; Azad et al., Citation2015). Contrarily, the transport of most of hydrophilic compounds and macromolecules, such as immunoglobulins, are tightly restricted by the BBB (Rodriguez et al., Citation2015; Weidle et al., Citation2015; Miranda et al., Citation2017a). These substances that cannot readily penetrate through the plasma membranes of BECs have to take an active transport pathway across the BBB (Weidle et al., Citation2015). The endothelial barrier defense system involves different protective mechanisms including transport barrier (paracellular and transcellular), enzymatic barrier, and immunologic barrier (Hendricks et al., Citation2015), in which the transport barrier plays a predominant role in the entire brain shielding system. In the strict sense, the major barrier function of the BBB is endorsed by the tight junctions (TJs) regulating the paracellular transport, and by the active efflux transporters (AETs) that mainly prevent brain entry of many anti-tumor drugs along transcellular transport pathway (). TJs appear as a branching network of sealing strands that effectively stitch adjacent endothelial cells together into a barricade () (Giepmans & van Ijzendoorn, Citation2009). Being an important type of cell-cell junction, TJs serve as a highly selective permeable dynamic biointerface between the blood and brain to control the passage of solutes by paracellular trafficking in between BECs (Abbott et al., Citation2006; Giepmans & van Ijzendoorn, Citation2009; Daneman & Prat, Citation2015). Generally, the diffusion of the biological compounds such as proteins and carbohydrates is restricted, whereas the penetration is allowed for hydrophobic molecules. The tight junction associated complex that expressed in the connective region between the BECs comprises a variety of transmembrane proteins such as occludins (1, 2, and 3) and claudins (1, 3, 5, and 12) as well as junctional adhesion molecules (JAM-A, -B and -C) (Wolburg & Lippoldt, Citation2002; Ballabh et al., Citation2004; Wolburg et al., Citation2007; Abbott et al., Citation2010). Occludins and claudins are two key components of TJs, playing a pivotal role in hampering paracellular diffusion. Claudin-1 and occludins are capable of binding tight junction associated proteins (Cingulin and 7H6) via zona occludens protein 1 (ZO-1), leading to regulation of the tight junction properties (Yu et al., Citation2005; Stamatovic et al., Citation2008). Moreover, claudin-3, claudin-5, and potentially claudin-12 are associated with the limitation of the small ions delivery (Wolburg & Lippoldt, Citation2002).
In addition to preventing paracellular transport, most of molecules, especially a large number of anti-tumor drugs, are not allowed for the transcellular diffusion across the BBB, which is mainly attributed to the presence of AETs. A variety of AETs, including P-glycoprotein (P-gp) (Demeule et al., Citation2002), breast cancer resistance protein (BCRP) (Huang & Sadée, Citation2006) and members of multidrug resistance-associated protein (MRP) (Kruh & Belinsky, Citation2003; Potschka et al., Citation2003; Zhang et al., Citation2004; Bronger et al., Citation2005; Soontornmalai et al., Citation2006), are present at the luminal side (blood side) of the BBB endothelium. These transporters belong to a superfamily of membrane proteins termed ATP-binding cassette (ABC) transporters which consists of seven different subfamilies (ABCA to ABCG) (Moitra & Dean, Citation2011). They act as ‘gatekeepers’ to control brain entry of xenobiotic compounds including molecularly targeted and non-targeted agents by actively effluxing these substances into luminal spaces for detoxification (Hartz & Bauer, Citation2011; Miranda et al., Citation2017a). The predominant AETs refer to P-gp and BCRP, both of them mainly control brain distribution of many anti-tumor drugs and thus reinforce the BBB (Agarwal et al., Citation2010; Agarwal, Hartz, et al., Citation2011; Agarwal, Sane, et al., Citation2011; Agarwal, Sane, Ohlfest, et al., Citation2011). P-gp is a 170 kD transmembrane protein which is encoded by the ABCB1 gene (Ambudkar et al., Citation1992). It has been extensively studied and reported to confer the tumors with significant multidrug resistance. P-gp resides only on the apical membrane of endothelial cells, which modulates drug transport in a unidirectional manner (Fung et al., Citation2014). It was already known that almost 60% of all marketed anti-tumor agents could be recognized by P-gp and then were pumped out of the cells back to the blood flow, resulting in reduced therapeutic efficacy and poor brain accumulation of drugs (van Tellingen et al., Citation2015). In addition to P-gp at the BBB, BCRP and other key efflux transporters such as MRP 1–5 that belong to the ABCC transporter family, play a critical role in restricting brain penetration of a large number of anti-tumor agents (Durmus et al., Citation2012; Lin, de Gooijer, et al., Citation2013; Gerber et al., Citation2014). Moreover, the fact that only a few pinocytic vesicles can be generated in BECs for transcellular transport of molecules is responsible for the limited drug penetration across the BBB as well (Hülper et al., Citation2013).
Next to the transport barrier, enzymatic barrier and immunologic barrier are another two defense mechanisms that contribute to the BBB. Some neurotoxins and drugs can be degradated by several intra- and extracellular enzymes in the BECs, such as esterase, peptidase, phosphatase, monoamine oxidase, and cytochrome P450, which act as a potentially metabolic hurdle to brain entry of drugs (van Tellingen et al., Citation2015). Furthermore, immunological responses can be triggered by a variety of BBB supporting cells including microglia and perivascular macrophages, providing a immunologic obstacle to drug delivery (van Tellingen et al., Citation2015). Taken together, the presence of BBB explains the inefficacy of most of chemotherapeutic agents that otherwise are potent to different cancers when tested for GBM therapy (Agarwal, Sane, et al., Citation2011; Jue & McDonald, Citation2016; Karim et al., Citation2016). Therefore, a potential approach to overcome the low access of anti-tumor agents to the tumor cells has become a major issue in the treatment of GBM.
1.3. BBTB
In GBM, the organization and function of the BBB can be impacted due to a series of pathological alterations caused by malignant tumor cells, leading to a tumor-specific delivery pattern of chemotherapeutic agents traversing the BBB. The barrier system in GBM is characterized by excessive vascularization with enhanced BBB permeability, which locates between capillary vessels and brain tumor tissues and is thus termed blood-brain tumor barrier (BBTB) (van Tellingen et al., Citation2015; Miranda et al., Citation2017a). The aberrant vascularization and dysfunction of the BBTB are mainly ascribed to over-expression of vascular endothelial growth factor (VEGF) and angiogenesis, which are triggered by tumor-induced hypoxic regions (Plate et al., Citation2012; van Tellingen et al., Citation2015). In addition to VEGF, some other pro-angiogenic factors released by GBM tumor cells, such as cytokines, are able to lead to BBB disruption (Oberoi et al., Citation2016).
Despite the observation of a dysfunctional BBTB in GBM, the degree of breakdown is not homogeneous in the entire barrier system, and an intact BBB occurs in the tumor tissues of many GBM patients (Oberoi et al., Citation2016). In general, the tumor bulk in GBM can be schematically divided into three major moieties: (i) the tumor core where the normal tissue is completely replaced by neoplastic cells and an enhanced permeability of the blood vessels is present, (ii) the angiogenic forehead which is mainly driven by VEGF expression, (iii) the brain adjacent to tumor, where the invading tumor cells infiltrate into normal brain tissue and the vasculature remains intact (Woodworth et al., Citation2014; van Tellingen et al., Citation2015; Dréan et al., Citation2016). In the past, a more plausible theory used by many is, that accumulation of therapeutic agents in the tumor tissues is favored due to the enhanced permeability and retention (EPR) effect originated from a leaky BBTB (Hendricks et al., Citation2015). Importantly, however, the GBM is well-known for highly invasive nature and thus does cause widespread proliferation of tumor cells which are located away from the tumor core with a compromised BBTB. These invasive tumor cells favor extending to otherwise healthy cerebral parenchyma with a tight BBB as well as with less marked EPR effect. Chemotherapeutic agents which otherwise are unable to cross the intact BBB can readily reach the main central areas of GBM through the disrupted BBTB, instead of the infiltration areas of GBM with a normal BBB (Sarkaria et al., Citation2018). Since the less altered BBB region is present in the entire BBB/BBTB system in GBM, a disrupted BBTB actually does not much conduce to drug accumulation in all tumor sites. This might help to understand the disappointing efficacy of most of anti-tumor agents in GBM therapy (Hendricks et al., Citation2015; Karim et al., Citation2016) as well as the inevitable recurrence of GBM even after a complete surgical resection of all contrast-enhanced regions of tumor (Hou et al., Citation2006). Being a key part of the healthy BBB, tight junctions (TJs) effectively prevent the transportation of a variety of chemotherapeutics into the CNS via paracellular pathway. However, the alterations in organization and structure of TJs under GBM conditions have been observed, which is associated with BBB dysfunction. Claudin-3, a critical component of TJs, is lost from the BBB in GBM as reported by Wolburg et al., implying its potential influence on the BBB integrity (Wolburg et al., Citation2003). Furthermore, a loss of claudin-1 in tumor microvessels as well as down-regulation of claudin-5 and occludin in excessive vascularization have been found, which are responsible for the leakiness of TJs and enhanced permeability of cerebral capillaries, and subsequently, for BBB dysfunction (Liebner et al., Citation2000). In addition, GBM is commonly featured with over-expression of the drug efflux transporters at the endothelial cells of the BBB/BBTB, especially P-glycoprotein (P-gp) (Leweke et al., Citation1998). Within the tumor necrotic regions, the protective role of P-gp is grossly reduced attributed to a compromised BBTB. Instead, P-gp activity is shown at the tumor borders where an intact BBB is present (Deeken & Löscher, Citation2007). This is of clinical significance in GBM treatment following surgical resection since tumor-specific over-expression of P-gp restricts brain entry of chemotherapeutic agents (Cheshier et al., Citation2009). Moreover, the expression of some drug efflux transporters is found in tumor cells as well, which further contributes to tumor multidrug resistance (Lin et al., Citation2014).
Taken together, BBB/BBTB prevents a variety of chemotherapeutic agents from reaching the GBM areas in the brain, particularly the tumor infiltration zone, forming a great challenge towards effective treatment of GBM. However, the mechanisms underlying the BBB pathological alterations associated with drug delivery into the CNS remain unclear. Continuous effort is necessary to direct toward understanding the BBB and the BBTB, achieving a complete and accurate pre-clinical evaluation of drug efficacy in GBM.
2. Delivery of small-molecule chemotherapeutic agents across the BBB/BBTB
In the following parts, we review in detail the delivery of anti-tumor agents across the BBB/BBTB in GBM, particularly small-molecule chemotherapeutic drugs via paracellular and/or transcellular transport pathways. Brain entry of protein therapeutics such as antibodies, as well as drug delivery mediated by carriers including liposomes, micelles, and nanoparticles are not the focus of this review.
2.1. Active efflux transport (AET)-dominated transcellular transport
As outlined above, one of the keys to effective therapy of GBM is achieving adequate accumulation of chemotherapeutic agents in the brain, which refers to their sufficient BBB/BBTB penetration. In general, brain entry of most of the chemotherapeutic agents is blocked due to the presence of endothelial TJs as well as the poor transcytosis (Bauer et al., Citation2014). A variety of active and specific transporter proteins are commonly expressed in the endothelial cells and involved in the transcellular transport systems, which mediate brain uptake and extrusion of various xenobiotic compounds including chemotherapeutic agents. To date, three main mechanisms of transcellular transport systems have been identified in the BBB (), which comprises receptor-mediated transport (RMT), carrier-mediated transport (CMT), and active efflux transport (AET) (Thomsen et al., Citation2012; Mikitsh & Chacko, Citation2014). RMT is one of the specific transcytosis mechanisms, which is based on endocytosis from the luminal side and exocytosis from the abluminal side of the endothelium (Lin, de Gooijer, et al., Citation2013). Some macromolecules including large anti-tumor protein therapeutics can be potentially transported across the BBB via RMT (Pardridge, Citation2003). Nevertheless, this aspect will not be in detail discussed here. CMT refers to the delivery of specific substances such as sugars, amino acids, organic cations and anions, nutrients and metabolites into the brain, which is mediated by a series of the solute carrier (SLC) transporters expressed at the BBB (Hediger et al., Citation2004). Glucose transporters (GLUTs), monocarboxylate transporters (MCTs), organic ion (cation and anion) transporters (OCTs and OATs), and nucleoside transporters are the major SLCs involved in CMT (Hediger et al., Citation2004; Molina-Arcas et al., Citation2009; Wong et al., Citation2013; Karim et al., Citation2016). Reportedly, OAT1, a classical organic anion transporter at the BBB endothelium, was able to modulate the transport of some anti-neoplastic agents, such as methotrexate, doxorubicin, and aclarubicin (Sekine et al., Citation2000).
The most crucial transcellular transport pathway is active efflux transport (AET) mechanism, which is predominant in the BBB for detoxification. AET is an ATP-driven mechanism that not only hinders brain entry of a large number of xenobiotics including potentially toxic substances and therapeutic agents but also transports the compounds that have crossed the BBB back into the circulation. All active efflux transporters (AETs) have been characterized by nucleotide binding sites that work as a catalytic domain for ATP hydrolysis, which is also reportedly associated with substrate recognition (Hollenstein et al., Citation2007). The AETs have a wide endogenous and exogenous substrate spectrum. The aforementioned P-gp (ABCB1) is able to identify non-polar and less amphiphilic molecules. Lipids, steroid hormones, and cytokines are the endogenous substrates of P-gp. Moreover, small-molecule chemotherapeutic agents like temozolomide, methotrexate, paclitaxel, anthracyclines, and vinca alkaloids have been proven to be the substrates of P-gp (Demeule et al., Citation2002; Agarwal, Sane, et al., Citation2011; Munoz et al., Citation2014). P-gp is able to actively pump these clinically used drugs out of the tumor region and the BBB/BBTB endothelium back to the brain capillary lumen, which explains their poor response in GBM therapy. Substrates of BCRP (ABCG2) include glutathione and steroid hormones (Mao & Unadkat, Citation2005) as well as some anti-tumor chemotherapeutic agents like methotrexate, mitoxantrone, and topotecan (Huang & Sadée, Citation2006), which overlap with those of P-gp (Staud & Pavek, Citation2005). Next to P-gp and BCRP, MRP refers to the ABCC transporter subfamily, which has 5 members (ABCC1-5) that locates at the BBB (Kruh & Belinsky, Citation2003; Potschka et al., Citation2003; Zhang et al., Citation2004; Bronger et al., Citation2005; Soontornmalai et al., Citation2006). MRPs are involved in BBB penetration of more polar molecules, including endogenous metabolites and drugs (Slot et al., Citation2011). However, both MRP1 (ABCC1) and MRP4 (ABCC4) have overlapping substrate profiles with those of P-gp and BCRP, as suggested by their similar substrate recognition of camptothecins (Lin, Marchetti, et al., Citation2013) and methotrexate (Kruh & Belinsky, Citation2003; Sane et al., Citation2014). MRP1 is also able to recognize vinca alkaloids and anthracyclines which are listed in the substrate spectrum of P-gp as well (Kruh & Belinsky, Citation2003). Together, most of the AETs effectively clear the small-molecule chemotherapeutic agents that are their substrates by modulating efflux of these drugs from the BBB/BBTB endothelium into the vascular lumen away from the cerebral parenchyma, which have major clinical significance for resistance to chemotherapy in the treatment of GBM. A list of the drug substrates of AETs in relation to GBM is summarized in .
Table 1. Summary of approved small-molecule chemotherapeutic agents for the treatment of brain tumors and their substrate status of AETs.
2.2. BBB/BBTB permeation of approved small-molecule agents for GBM
Till now, most curative chemotherapeutic agents with better BBB/BBTB passage for GBM therapies are temozolomide (TMZ) (Reyderman et al., Citation2004; Portnow et al., Citation2009; Goldwirt et al., Citation2014), lomustine (CCNU) (Taal et al., Citation2014), procarbazine (Sekine et al., Citation2000; Weidle et al., Citation2015), and carboplatin (Jacobs et al., Citation2010; Weidle et al., Citation2015) (). TMZ is to date the most frequently used and the FDA-approved chemotherapeutic agent for GBM treatment (Fernandes et al., Citation2019). In human brain adjacent to tumor, TMZ displayed a brain/plasma ratio of 18% and a cerebrospinal fluid (CSF)/plasma ratio of 20–40% (Portnow et al., Citation2009; Goldwirt et al., Citation2013). On an in vivo model of mice, maximal exposure of TMZ in plasma and brain was almost reached concomitantly, namely, the close Tmax for both cases, indicating good BBB/BBTB penetration (Reyderman et al., Citation2004). However, the expression level of ABCB1 at the BBB/BBTB can affect brain uptake of TMZ, strongly suggesting that TMZ is one of the ABCB1 substrates (Reyderman et al., Citation2004). Compared with ABCB1, the role of ABCG2 on transport of TMZ across the BBB/BBTB is negligible. In addition, higher tumor accumulation of TMZ is partially attributed to the enhanced permeability and retention (EPR) effect resulted from a less intact BBB (Zhou & Gallo, Citation2009). CCNU is another chemotherapeutic drug which has been currently tested in clinical trial dedicated to GBM patients (Sepulveda-Sanchez et al., Citation2015). CCNU exhibited a lower brain/plasma ratio of 20% in rats compared with 22–41% for TMZ, although it is not involved in the substrate spectrum of any ABC transporter (Ostermann et al., Citation2004; Azad et al., Citation2015; Dréan et al., Citation2016). By contrast, procarbazine and carboplatin revealed lower or medium BBB/BBTB passage (Jacobs et al., Citation2010; Dréan et al., Citation2016) and higher CNS toxicity (Newton, Citation2012). The clinical efficacy of them for treatment of brain tumors was still under investigation (Owonikoko et al., Citation2014).
Figure 2. Chemical structures and molecular weight of small-molecule chemotherapeutic agents with good BBB/BBTB permeability in treatment of GBM.
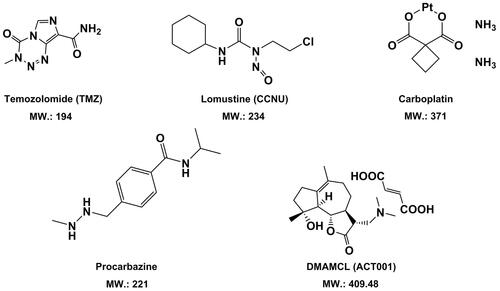
What most worth mentioning is, that a novel small-molecule chemotherapeutic agent against glioblastoma, DMAMCL (also registered as ACT001), which is featured with a framework of sesquiterpene lactone, has been newly discovered (Zhang et al., Citation2012; An et al., Citation2015). Reportedly, it revealed up to 88% tumor inhibition effect in glioblastoma murine models (An et al., Citation2015). Notably, ACT001 displayed high permeability across the BBB/BBTB in vivo. As a novel anti-GBM drug, ACT001 has currently entered in Phase I clinical trials in Australia (trial ID: ACTRN12616000228482) and received the orphan drug designation for GBM from both the FDA in the USA (https://www.accessdata.fda.gov/scripts/opdlisting/oopd/detailedIndex.cfm?cfgridkey=610217.) and the EMA in Europe.
An empirical approach to simply estimate BBB permeability of small-molecule anti-tumor agents, namely ‘the rule of 5’, has been developed by Lipinski in 2001 (Lipinski et al., Citation2001). To date, this criteria has been still widely applied for predictions of drug-ability and increasingly served as a useful tool for BBB-permeable screening of compounds in drug discovery, although it is not always in line with the experimental data. Herein, instead of going to in detail elucidate the rule of 5, we would introduce four parameters that are considered to be globally associated with BBB penetration, which are molecular weight (MW), Log P, the number of hydrogen bond donors and the number of hydrogen bond acceptors (Lipinski et al., Citation2012). Better BBB crossing is possible for a new compound when its MW is less than 500 Da; the Log P is below 5; the sum of OH and NH groups (hydrogen bond donors) and the sum of oxygen and nitrogen (hydrogen bond acceptors) are less than 5 and 10, respectively. Due to a cutoff value of 5 or a multiple of 5 for each of the four parameters, it is called ‘the rule of 5’ and is convenient in application. Lipinski’s criteria can also be further simplified, where the limitation of the number of hydrogen bond acceptors is omitted. The rule of 5 has successfully predicted BBB/BBTB passage in vivo of the aforementioned chemotherapeutic agents against GBM, as shown in Table S1 in Supplemental Material. For instance, TMZ possesses the lowest experimental Log P value of −2.8 compared with other drugs, indicating the best penetration across the BBB/BBTB. In addition to the rule of 5, rotatable bond count and polar surface area (PSA) of a molecule are another two parameters related to BBB permeability of drugs as well. In general, molecules with more than 10 rotatable bonds are usually believed to have poor BBB permeability. All the drugs shown in have rotatable bond count either below or equal to 5. The polar surface area (PSA) refers to the overall surface over all the polar atoms of a molecule, including oxygen, nitrogen, sulfur, phosphorus as well as the attached hydrogens. For a new drug candidate against brain tumors, permeating the BBB/BBTB into the CNS is favored when the PSA is less than 0.90 nm2 (And & Pennington, Citation2006). However, it is still controversial since the prediction from the PSA is not all the time in consistence with that of Lipinski’s criteria. As seen in , the PSA of TMZ is 1.06 nm2 which is greater than 0.53 nm2 of procarbazine and 0.62 nm2 of CCNU, implying that TMZ is less able to traverse the BBB/BBTB. Instead, the rule of 5 predicts that TMZ is more readily to cross the BBB/BBTB than procarbazine and CCNU, which is also in agreement with the reported experimental data in vivo (Jacobs et al., Citation2010; Azad et al., Citation2015; Dréan et al., Citation2016). As for the new small anti-GBM agent ACT001, a physio-chemical profile that hits the limitations of both Lipinski’s rule and PSA is shown (see Table S1 in Supplemental Material) and a convictive BBB/BBTB permeation has been observed in murine models (An et al., Citation2015). Apart from the molecules displayed in , the capacity of penetrating the BBB/BBTB for many other small anti-tumor agents can be estimated by Lipinski’s criteria coupled with the PSA parameters as well. Methotrexate (454 Da) and doxorubicin (544 Da) are two well-known agents which bear a small molecular size of ∼500 Da and have been used as mono-therapy in GBM patients (Newton, Citation2012). Based on Lipinski’s criteria, methotrexate and doxorubicin are predicted to be poor at permeating the BBB/BBTB, as suggested by 12 hydrogen bond acceptors for both (see Table S1 in Supplemental Material). Their large PSA of greater than 2 nm2 indicating poor lipophilicity also support the estimation of the rule of 5. And these predictions are consistent with the experimental data in vivo (Van et al., Citation1999; Westerhout et al., Citation2014).
2.3. BBB/BBTB permeation of drug candidates with anti-GBM potential
Based on the medicinal chemistry strategies in the development of small molecules for GBM chemotherapy, some anti-GBM drug candidate compounds derived from pyrimidines, quinolines, indazoles, and triazines have been identified in the last decade (Fernandes et al., Citation2019). These compounds have been demonstrated to effectively inhibit the GBM tumor growth in a panel of models in vivo, indicating their better BBB/BBTB permeable potential. Several candidate compounds with superior anti-GBM activity in vivo are displayed in . (The parameters related with their BBB/BBTB penetration efficacy are summarized in Table S2 in Supplemental Material).
Figure 3. Chemical structures and molecular weight of small candidate molecules (1–6) with promising anti-GBM activity in vivo.
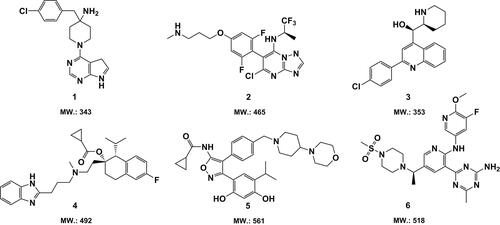
The pyrimidine-based compounds 1, namely the serine-threonine kinase AKT inhibitor CCT128930, induced remarkable tumor growth inhibition in a human GBM xenograft mice model (Yap et al., Citation2011). Another pyrimidine-based candidate compound 2 exhibited potent efficacy against human GBM U87MG cells xenografted in mice at a single dose of 25 kg/mg (Beyer et al., Citation2008). Compound 3, which contains a quinoline moiety, has been reported to be able to put a brake on the human U3013 GBM cells proliferation in an orthotopic zebrafish model xenografted and meanwhile showed promising bioavailability in mouse tissue (Hammarström et al., Citation2016). The experimental data implies, that compound 1–3 might have potential to effectively traverse the BBB/BBTB, which is also suggested by the prediction of the rule of 5 (see Table S2 in Supplemental Material). Compound 4–6, which are respectively derived from indazoles (Kim et al., Citation2015), triazines (Norman et al., Citation2012), and oxygen-containing heterocyclics (Chen et al., Citation2014), displayed significant inhibitive effect on tumor growth of U87MG GBM cells xenografted in mice in vivo. Thus, it is possible that they are capable of crossing the BBB/BBTB. However, the parameters shown in Table S2 gave the opposite predictions. Note that compound 6 bears not only a bigger molecular size than 500 Da (518 Da) but also a PSA of 1.50 nm2 greater than 0.90 nm2 and 12 hydrogen bond acceptors beyond the threshold of 10, which strongly suggests that compound 6 is less lipophilic and thus might be not a potential BBB/BBTB permeable agent. The cases of compound 4–6 state the limits of predictive Lipinski’s criteria.
2.4. Drug re-purposing and some BBB-permeable small molecules
Besides to the traditional medicinal chemistry strategies, drug re-purposing approach could accelerate the identification of new small drug candidates with favorable BBB/BBTB permeable properties since many existing drugs have previously revealed acceptable safety and pharmacokinetic profiles (Sminia & Westerman, Citation2016). Hence, we need to temporarily look away from GBM and re-focus on the already marketed drugs, and the candidate compounds which have been selected for other diseases and are potentially effective in GBM therapy. A library of clinically approved drugs that are routinely employed against several disorders, such as anti-psychotic drugs, could be re-positioned as anti-tumor agents with certain BBB/BBTB permeable potential, as shown in .
Figure 4. Chemical structures and molecular weight of (A) approved small-molecule drugs (7–10) re-positioned as anti-GBM agents and (B) imidazolium-based candidate molecules (11–16) with promising BBB permeability.
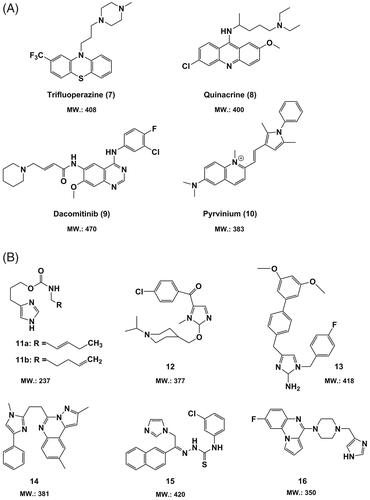
Trifluoperazine (7), a typical phenothiazine-based dopaminergic ligand bearing a piperazine cyclic moiety, has been largely used as a dopamine receptor D2 antagonist in schizophrenia treatment. This indicates its better BBB permeability, which is in line with the prediction by Lipinski’s criteria (see Table S2 in Supplemental Material). Trifluoperazine (7) displayed a potent anti-GBM activity of 1.34 and 1.85 μM EC50 against U3046MG and U3005MG GBM cells, respectively, and was thus identified as a potential anti-GBM drug of traversing the BBB/BBTB (Pinheiro et al., Citation2017). Quinacrine (8), an anti-malarial drug that is derived from acridine, has been demonstrated to be able to induce apoptosis and endoplasmic reticulum stress in vivo, which led to cell death of U87 GBM cells xenografted in mice (Golden et al., Citation2015). The EGFR tyrosine kinase inhibitor dacomitinib (9), which has been primarily used against non-small-cell lung carcinoma, significantly inhibited intracranial tumor growth by using mice xenografted with GBM cells, which was evidenced by a reduction of tumor cells proliferation and an induction of apoptosis of tumor cells (Zahonero et al., Citation2015). The anthelmintic drug pyrvinium (10) were reportedly able to abrogate the formation and the recurrence of GBM in GBM xenograft mice (Venugopal et al., Citation2015). For drug 8–10, nearly all the parameters related with BBB/BBTB crossing reached the requirements of the rule of 5 (see Table S2 in Supplemental Material), which implies their better BBB/BBTB penetration in vivo.
In addition to the re-purposed small-molecule drugs with potential anti-GBM activity, a large number of imidazolium-based drug candidates including anti-histaminic, anti-neuropathic, and serotonin (5-HT)-targeted compounds, were selected for their capability to pass the BBB (Sminia & Westerman, Citation2016). As shown in , compound 11a and 11 b are two imidazolium carbamates bearing an unsaturated alkyl chain attached to the amino group. Both of them showed highly H3 receptor (H3R) antagonistic activity in vivo and were able to penetrate across the BBB after per-oral administration to Swiss mice (Łazewska et al., Citation2009). Compound 12 containing a piperidine group was also a highly H3R-selective antagonist with a low Ki of 3 nM, and displayed remarkable brain entry effect in vivo (Ishikawa et al., Citation2010). Being a potent anti-AD drug candidate, compound 13 which is featured with an active moiety of 2-aminoimidazole, revealed a desired BACE-1-targeted activity (IC50=7.4 μM) as well as promising BBB-permeable properties (Chiriano et al., Citation2012). The imidazole derivative compound 14 served as a highly selective phosphodiesterase 10 A (PDE10A) inhibitor (IC50 = 16 nM) for the treatment of Parkinson’s disease (PD). In comparison to its benzimidazole analog, compound 14 revealed an improved BBB penetration efficacy (Wager et al., Citation2010; Asproni et al., Citation2011). Compound 15, a Schiff-base derivative, worked as an effective anti-convulsant candidate agent together with an appropriate liposolubility favoring BBB passage (Caliş et al., Citation2011). The imidazolium-based compound 16 with a quinoxaline segment was not only a highly 5-HT3 receptor-targeted drug candidate but also identified to be a good BBB-permeable agent (Butini et al., Citation2009). Note that, all the imidazolium derivatives included in are predicted to able to pass the BBB but compound 13, as suggested by the parameters summarized in Table S2 in Supplemental Material.
2.5. A simple screening strategy of small BBB/BBTB-permeable drugs
In order to further identify new small-molecule anti-GBM candidate compounds with desired pharmacokinetic profile of BBB/BBTB permeation, the structure-activity relationship (SAR) of the aforementioned drugs and drug candidates i.e. the relationship between their structural features and their BBB/BBTB permeability is described here. First of all, the SAR pointed out that the lipophilic moieties like aromatic rings and alicyclic rings were indispensable for effective BBB/BBTB passage of small molecules. And the substitution of the hydrogen on the benzene ring by chlorine (compound 1, 2, 3, 8, 9, 12, 15), fluorine (compound 2, 4, 6, 9, 13, 16), methoxyl group (compound 6, 8, 9, 13), and trifluoromethyl group (trifluoperazine (7) was beneficial for improving BBB/BBTB permeability since they were able to boost up the lipid-solubility of drug molecules. Secondly, an active nitrogen posed as a trialkylamine form was present in most of the drugs and drug candidates above mentioned. Such active nitrogen seems most likely to play a vital role in increasing the oil-water partition coefficient of drug molecules and to contribute to their anti-GBM activity. Furthermore, the introduction of a panel of heterocycles containing tertiary amines, including piperidine (compound 1, 3, 5, 9, 12), piperazine (compound 6, 7, 16) and morpholine (compound 5), had a positive influence on BBB/BBTB passage, which was reflected by the SAR as well. Finally, it was worthy to note that, most of the aforementioned drugs and drug candidates reached the limitations given by Lipinski’s criteria with respect to the prediction of their BBB/BBTB penetration. According to all the above-discussed points of the SAR, a simple and optimized screening strategy which combines the advantages of medicinal chemistry approaches and drug re-purposing programs to identify the small-molecule drug candidates with superior BBB/BBTB permeable efficacy could be concluded as follows:
the molecule possesses appropriate lipid-solubility, which can be estimated by the count of aromatic rings and/or alicyclic rings;
the molecule is structurally featured with the trialkylamine-based moiety, including dimethylamine and a series of nitrogen-containing heterocycles such as piperidine, piperazine, and morpholine;
the molecule is predicted to be able to traverse the BBB/BBTB based on the rule of 5.
Following this integrated approach, people could efficiently select small molecules with optimal characteristics of high BBB/BBTB passage from a large number of drug candidates bearing anti-GBM potential. In addition, many public data sources with respect to a variety of bio-pharmaceutic parameters of drugs, such as bio-availability, molecular fingerprint, and neurotoxicity, are also helpful for the identification of BBB/BBTB-permeable drugs (Sminia & Westerman, Citation2016). Nonetheless, the drug screening process is time-consuming and complex, and it is hard to discover a drug molecule that is inherently able to traverse the BBB/BBTB, given a limited number of small molecules overcoming or bypassing the impermeable obstacle of BBB/BBTB. Therefore, there is a great need to develop a series of approaches and techniques that could enable the opening or the disruption of the BBB/BBTB system to improve drug delivery within the GBM tumor site.
3. Strategies for overcoming the BBB/BBTB
Despite many ongoing efforts during the past decades to develop novel chemotherapeutic agents to treat GBM, none of them showed the satisfactory therapeutic effect or led to the enhanced quality of life for GBM patients. The failed attempts are mainly ascribed to the inadequate drug delivery into the tumor tissue because the BBB/BBTB greatly prohibits brain entry of a number of anti-tumor drugs including small-molecule chemotherapeutic agents. To counteract the protective effect of BBB/BBTB, a variety of approaches and techniques have been emerged and become more refined. Basically, these methods were established with the goal of circumventing and modulating the entire BBB/BBTB system, which consists of active efflux transporter (AET) targeted and tight junction (TJ) targeted strategies. The former refers to overcoming active efflux at the BBB/BBTB via structural modification of drug candidates as well as pharmacological inhibition of active efflux transporters (AETs). The TJ-targeted ones involve a series of approaches and techniques applied for opening or disruption of BBB/BBTB, which include chemical-mediated BBB/BBTB disruption, hyper-osmotic BBB/BBTB disruption, and thermotherapy-induced BBB/BBTB opening such as focused ultrasound (FUS), radio-frequency microwaves, and laser interstitial thermotherapy (LITT). Several of them have shown promise and many of the patients with GBM could benefit from these improved drug delivery regimens. In the following section, we will discuss in detail about the current efforts and advances in each of these strategies, excluding nanosystem-based delivery, convection-enhanced delivery, and other delivery systems related to therapeutic interventions.
3.1. AET-targeted BBB/BBTB disruption
To reach a drug molecule with enhanced BBB/BBTB permeability, current chemical modification of existing drugs have focused on minimizing their molecular size and increasing their lipophilicity. Given the main defense mechanisms of BBB/BBTB to drug uptake, an anti-GBM drug candidate could be modified to an analog of the ligand against the specific receptor at the BBB/BBTB or could be linked to the ligand to receptor-mediated transport (RMT). For instance, addition of transferrin to drugs can promote their BBB/BBTB passage via transcytosis mediated by transferrin receptor (TfR) (Zhang et al., Citation2015). The modification of a marketed anti-cancer drug Doxil® (PEGylated liposomes of doxorubicin) with glutathione groups showed a 4.8-fold increased brain/plasma ratio in comparison to Doxil® alone in preclinical studies (Birngruber et al., Citation2014; Gaillard et al., Citation2014). Lipid carriers like fatty acids can also be employed to improve drug penetration through the BBB/BBTB. Small drug paclitaxel acquires increased BBB/BBTB permeability by covalent conjugation of N-docosahexaenoic acid (DHA) (Bradley et al., Citation2001). In addition to the biochemical modifications using the ligand to RMT, structural refinement of drugs to diminish their affinity to AETs at the BBB/BBTB greatly contributes to their brain entry, given that a number of anti-tumor drugs especially molecularly targeted agents have proven substrate liability for both P-gp (ABCB1) and BCRP (ABCG2) (Oberoi et al., Citation2016). BMK120, a phosphatidylinositol-3 kinase (PI3K) targeted inhibitor, displayed not only superior BBB/BBTB penetration as a consequence of structural modification of minimizing its affinity to AETs but also promising effect in GBM models in vivo (Wen et al., Citation2012). The best approach to overcome active efflux of anti-tumor drugs is using pharmacological inhibitors of AETs. Since most agents are substrates of both P-gp and BCRP (), inhibition of both AETs should be mandatory to achieve adequate brain uptake of drugs (van Tellingen et al., Citation2015). A number of inhibitors including cyclosporin A, valspodar, elacridar, and tariquidar are able to modulate the activity of both P-gp and BCRP in multiple pre-clinical models (Bankstahl et al., Citation2013). As a representative of the first generation inhibitors, cyclosporin A can effectively reverse the efflux effect of P-gp at the BBB/BBTB and an increased brain distribution of docetaxel was observed in mice (Kemper, Boogerd, et al., Citation2004). However, direct inhibition of AETs at the BBB/BBTB by using most of the first generation inhibitors did not appear sufficient efficacy in vivo. This was attributable to their poor binding affinities, which requires the usage of high doses and thus results in intolerable toxicities (Doyle & Ross, Citation2003; Bhowmik et al., Citation2015). The treatment of valspodar, a second generation P-gp inhibitor, was reported to induce 9.08-fold increase in brain uptake of vinblastine in rats (Drion et al., Citation1996). Similarly, brain accumulation of docetaxel was also enhanced when it was co-injected with valspodar in mice (Kemper, Boogerd, et al., Citation2004). However, both the first and second generation inhibitors have been met with moderate efficacy and limited safety, which restricts their application in clinic. Accordingly, the third generation inhibitors with high affinity to AETs and acceptable tolerability have been developed. Currently, elacridar and tariquidar are the two most promising options in all the third generation inhibitors of AETs. Increased brain concentrations of molecularly targeted agents gefitinib (Agarwal et al., Citation2010) and vandetanib (Minocha et al., Citation2012) have been observed in mice with co-injection of elacridar. Moreover, as reported by Kemper et al., paclitaxel uptake was improved 5-fold in the brain with co-administration of elacridar (Kemper et al., Citation2003). Agarwal et al. showed higher brain accumulation of erlotinib by combinatorial treatment of elacridar in an orthotopic rat xenograft model of U87 GBM (Agarwal et al., Citation2013). Furthermore, elacridar is also able to inhibit P-gp mediated efflux of docetaxel (Kemper, Boogerd, et al., Citation2004) and topotecan (de Vries et al., Citation2007) from the brain. Unfortunately, the excess toxicity induced by the concomitant use of elacridar and cytotoxic chemotherapeutic agents like doxorubicin was also present (Planting et al., Citation2005). Hence, developing an inhibitor with high specificity to AETs as well as suitable tolerability is necessitated for overcoming active efflux of drugs and worth pursuing in the future.
3.2. TJ-targeted BBB/BBTB disruption
Apart from the AET-targeted BBB/BBTB breakdown as discussed above, another attempt to facilitate brain entry of small-molecule drugs is opening or disruption of BBB/BBTB using a panel of chemical and pharmacological agents as well as techniques that are TJ targeted. Since the drug paracellular transport is mainly modulated by tight junctions, it is possible that TJ-targeted means favor the passive diffusion of anti-tumor agents across a compromised BBB/BBTB.
Chemical-mediated BBB/BBTB disruption involves exposing the barrier system to a specific chemical agent that is able to alter the BBB/BBTB integrity. A panel of vasoactive compounds like histamine (Oberoi et al., Citation2016; Miranda et al., Citation2017b), bradykinin (Azad et al., Citation2015; Oberoi et al., Citation2016; Miranda et al., Citation2017b), alkylglycerols (Erdlenbruch et al., Citation2003; Hülper et al., Citation2013), and tumor necrosis factor ɑ (TNF-ɑ)/interferon γ (INF-γ) (Lopez-Ramirez et al., Citation2012) can make tight junctions (TJs) disrupted through stimulating B2 receptors at the endothelium and thus induce a transiently increased cytosolic Ca2+, which leads to opening of BBB/BBTB (Kemper, Verheij, et al., Citation2004). Bradykinin and its agonist RMP-7 are two agents that have been most widely studied for chemical-mediated disruption approach. Bradykinin can selectively target B2 receptors, leading to a reduced BBB/BBTB integrity and an elevated drug concentration in cerebral parenchyma (Alyautdin et al., Citation2014; Jue & McDonald, Citation2016). In comparison to bradykinin, RMP-7 is not only more potent and B2-selective but also resistant to degradation of bradykinin-metabolizing enzymes. In an RG2 rat-glioma model, the delivery of carboplatin to tumors was improved by 30–80% with co-administration of RMP-7 (Borlongan & Emerich, Citation2003). Another option for opening the BBB by chemical agents is the utilization of alkylglycerols (AGs). Two short-chain AGs, 1-O-pentylglycerol and 2-O-hexyldiglycerol, showed a reversible increase in BBB permeability without alterations of TJ strand complexity in an in vitro BBB model of primary rat brain endothelial cells co-cultured with rat cerebral glial cells (Hülper et al., Citation2013). Moreover, the intracarotid injection of 200 mM 1-O-pentylglycerol led to a significant increase in brain concentration of the co-injected methotrexate in a concentration-dependent manner in nude mice (Erdlenbruch et al., Citation2003). Despite the complex nature of chemical-mediated BBB/BBTB disruption, involving limited effectiveness and side effects, this regimen continues to remain an active area of BBB/BBTB breaching due to the BBB/BBTB interactive potential of vasoactive mediators.
Similarly, hyper-osmotic BBB/BBTB disruption refers to a technique that has been widely applied for transiently opening the BBB/BBTB and increasing BBB/BBTB permeability by hyperosmolar agents, most commonly mannitol. Following the administration of mannitol, water was withdrawn from the endothelial cells into the vascular lumen, thus effectively contracting intracellular volume. Dehydration of cerebral endothelium and shriveling of endothelial cells cause a consequent opening of TJs and an increased BBB permeability (Rodriguez et al., Citation2015). This allows for a therapeutic window of a few hours, which can then be employed for the intra-arterial (i.a.) administration of the chemotherapeutic agents, such as methotrexate or carboplatin (Gerber et al., Citation2014). Generally, a chemotherapeutic agent of interest was i.a. delivered following the injection of a standard dose of 1.4 M mannitol (10 mL) over two minutes (Burkhardt et al., Citation2012). Several studies suggest that the method to disrupt the BBB/BBTB by mannitol increases brain entry of anti-tumor chemotherapeutic agents by 10- to 100-fold compared to administrating the drug alone (Miller, Citation2002). Remarkable evidence of tumor reduction and prolonged progression-free survival have been reported in a phase I trial in the patients with malignant gliomas after mannitol-induced BBB/BBTB disruption followed by i.a. administration of melphalan/carboplatin (Guillaume et al., Citation2010). Although some studies supported the effectiveness and the safety of this approach (Doolittle et al., Citation2000), the technical challenges of the procedure, as well as systemic neurological toxicity, (Kemper, Verheij, et al., Citation2004) limit the feasibility of osmotic disruption technique in pre-clinical and clinical trials.
Being different from the approaches disrupting the BBB/BBTB mediated by chemicals and hyperosmotic agents, thermotherapy-induced BBB/BBTB opening encompasses a variety of physical techniques for generating alterations of the barrier integrity. Most relevant to this strategy are the techniques of focused ultrasound (FUS), radio-frequency microwaves, and laser interstitial thermotherapy (LITT). These techniques induce a mechanical destabilization of TJs in the cerebrovasculature and thus enhance the barrier permeability by producing intracranial hyperthermia. FUS is a noninvasive technique which thermo-mechanically disrupts the BBB/BBTB through transcranial delivery of low-frequency ultrasound waves (Hendricks et al., Citation2015; Rodriguez et al., Citation2015). FUS itself is able to thermally ablate the tumor tissue and the heating effect on the area of interest can be enhanced in combination with microbubbles (Kim et al., Citation2008). More importantly, the simultaneous utilization of microbubbles with low-intensity FUS induces transient opening of TJs by producing shear stress in endothelial cells (VanBavel, Citation2007) or by activation of signaling pathways modulating the barrier permeability (Jalali et al., Citation2010), leading to a tumor-located and reversible BBB/BBTB disruption (Sheikov et al., Citation2004). Typical settings of FUS are repeated ultrasonic exposure bursts at the frequency of 1 Hz at 10 ms used for 20–30 s durations (Rodriguez et al., Citation2015). Intravenous (i.v.) administration of microbubbles has been used for lowing the ultrasound intensity required for BBB/BBTB disruption (Hynynen et al., Citation2001). Moreover, the size selectivity of BBB/BBTB disruption can be manipulated by the applied FUS, allowing for BBB/BBTB crossing of the agents bearing a molecular weight up to 2000 kD (Chen & Konofagou, Citation2014). Several pre-clinical studies have approved the feasibility of BBB/BBTB disruption by FUS in the administration of chemotherapeutic agents for GBM therapy. The FUS-induced BBB/BBTB disruption was reported to improve brain accumulation of temozolomide (TMZ) from 6.98 to 19 ng/mg in nude mice bearing human U87 glioma cells (Liu et al., Citation2014). In another pre-clinical study, cerebrospinal fluid (CSF)/plasma ratio of TMZ was increased from 22.7% to 38.6% by application of FUS in rats with gliomas (Wei et al., Citation2013). In healthy rabbits, delivery of Irinotecan was elevated from 206% to 331% via FUS-induced disruption technique (Beccaria et al., Citation2016). Moreover, prolonged survival has been observed in rats with glioma that treated by FUS accompanied by BBB-impermeable liposomal doxorubicin (Aryal et al., Citation2013). Reportedly, the microbubble-enhanced FUS was shown to promote brain entry of carmustine (BCNU) in rats, as described by Ting et al. (Ting et al., Citation2012). Despite the risk of uncontrolled thermal injury in brain as well as undesirable side effects of edema and intracerebral hemorrhage, FUS technique displayed several advantages over other approaches for BBB/BBTB disruption because it is noninvasive, repeatable, and economical to conduct. Recently, this technique has been translating from pre-clinical studies to the clinic. In a clinical case, in a patient with recurrent GBM 0.7 cc of the tumor, which corresponds to 10% of the enhancing tumor volume, was ablated following the FUS application of 25 sonications of 10–25 s and 150–950 Watts of acoustic energy used for only 5 h (Coluccia et al., Citation2014). Therefore, FUS-induced BBB/BBTB disruption coupled with microbubbles seems very feasible in GBM patients in the future clinical trials and shows translational study success to warrant further optimization of this approach in clinical trials.
Radio-frequency microwaves and laser interstitial thermotherapy (LITT) are two additional modalities that induce BBB/BBTB disruption through the administration of heat. The former originates from the typical utility of radiation therapy to ablate the brain tumor by inducing DNA damage. Since a minimal invasive and selective BBB/BBTB disruption by radio-frequency microwaves was seen in several animal and human models (Patel & Mehta, Citation2007; Lemasson et al., Citation2010), radiation therapy is expected to be applied in both tumor ablation and BBB/BBTB disruption in the future clinical studies. By contrast, LITT is a novel technique that allows for destroying the tumor tissue via laser ablative hyperthermia (Rodriguez et al., Citation2015). LITT is able to cause a destruction of cell membranes, consequently leading to coagulative necrosis of the tumor. Meanwhile, cell membrane destruction also induces BBB/BBTB disruption, which may provide a chance for brain entry of anti-tumor agents. To date, LITT has been successfully used for the treatment of a panel of tumors including GBM (Carpentier et al., Citation2012; Sloan et al., Citation2013). Importantly, the LITT-induced thermal ablation region where the compromised BBB/BBTB occurs has been observed by followed gadolinium-enhanced magnetic resonance imaging (MRI) (Hawasli et al., Citation2014). Nonetheless, whether BBB/BBTB crossing of chemotherapeutic agents is enhanced following LITT application remains still unknown.
4. Conclusions and prospect
Till date, GBM remains one of the most difficult-to-treat central nervous system tumors, and the most aggressive in nature. The grim prognosis and inevitable recurrence characterize this neoplasm, which is at least in part ascribed to lack of effective brain entry of most anti-tumor chemotherapeutic agents to achieve sufficient accumulation in the invasive zone beyond the bulk GBM. Both BBB and BBTB serve as the major hurdles hampering effective treatment of GBM by denying chemotherapeutic agents reach the tumor infiltration regions. In this review, we have focused exclusively on the delivery of small-molecule anti-tumor agents across the BBB/BBTB as well as advances in development of therapeutic strategies to overcome the BBB/BBTB. Bearing in mind the irreplaceable role of chemotherapy in treatment of GBM, identification of novel small-molecule chemotherapeutic agents with both potent anti-GBM activity and promising brain entry efficacy is always commendable and is in urgent need. Herein we have presented a comprehensive synopsis concerning evaluation of the BBB/BBTB permeability of marketed drugs including temozolomide (the current standard chemotherapy in GBM) and their anti-tumor efficacy in vivo as well as a simple drug screening strategy combining the advantages of medicinal chemistry and drug re-purposing. In parallel, opening or disruption of BBB/BBTB also hold the key to enhance the delivery of small anti-tumor agents to the brain. Targeted inhibition of active efflux transporters (AETs) at the BBB/BBTB might be preferred to confer an improved drug delivery. In addition, multiples new chemical and physical therapeutic approaches have been introduced for BBB/BBTB disruption, such as chemical and osmotic disruption technique as well as thermotherapy. Investigation into these new approaches and techniques will provide the fundamental basis for an optimized combinatorial treatment and accordingly abrogate the obstacles that previously hindered brain access of anti-tumor drugs.
As we stand today, GBM remains incurable worldwide and few patients with malignant GBM have yet profited although considerable effort and investment have been devoted to the development of novel chemotherapeutic drugs and new therapeutic approaches for GBM. The battle against lethal GBM demands more powerful weapons and strategies. Continuous efforts deserve to be directed toward medicinal chemistry to discover more potent small-molecule chemotherapeutic agents and toward advancing the current approaches for BBB/BBTB disruption to clinical applications in GBM patients. Convincingly, a growing number of small anti-GBM drugs bearing superior BBB/BBTB-penetrating characteristics as well as innovative strategies and techniques breaching the BBB/BBTB are going to emerge in the foreseeable future and to give us breakthrough news. Hopefully, stepwise advancements of therapeutic benefit can be approved in a few years ahead.
Supplemental_Material-2.docx
Download MS Word (15.4 KB)Supplemental_Material-1.docx
Download MS Word (17.6 KB)Disclosure statement
No potential conflict of interest was reported by the authors.
Additional information
Funding
References
- Abbott NJ, Patabendige AA, Dolman DE, et al. (2010). Structure and function of the blood-brain barrier. Neurobiol Dis 37:13–25.
- Abbott NJ, Rönnbäck L, Hansson E. (2006). Astrocyte-endothelial interactions at the blood-brain barrier. Nat Rev Neurosci 7:41–53.
- Adeberg S, Bostel T, König L, et al. (2014). A comparison of long-term survivors and short-term survivors with glioblastoma, subventricular zone involvement: a predictive factor for survival. Radiat Oncol 9:95.
- Agarwal S, Hartz AM, Elmquist WF, Bauer B. (2011). Breast cancer resistance protein and P-glycoprotein in brain cancer: two gatekeepers team up. CPD 17:2793–802.
- Agarwal S, Manchanda P, Vogelbaum MA, et al. (2013). Function of the blood-brain barrier and restriction of drug delivery to invasive glioma cells: findings in an orthotopic rat xenograft model of glioma. Drug Metab Dispos 41:33–9.
- Agarwal S, Sane R, Gallardo JL, et al. (2010). Distribution of gefitinib to the brain is limited by P-glycoprotein (ABCB1) and breast cancer resistance protein (ABCG2)-mediated active efflux. J Pharmacol Exp Ther 334:147–55.
- Agarwal S, Sane R, Oberoi R, et al. (2011). Delivery of molecularly targeted therapy to malignant glioma, a disease of the whole brain. Expert Rev Mol Med 13:e17.
- Agarwal S, Sane R, Ohlfest JR, Elmquist WF. (2011). The role of the breast cancer resistance protein (ABCG2) in the distribution of sorafenib to the brain. J Pharmacol Exp Ther 336:223–33.
- Alvarez JI, Katayama T, Prat A. (2013). Glial influence on the blood brain barrier. Glia 61:1939–58.
- Alyautdin R, Khalin I, Nafeeza MI, et al. (2014). Nanoscale drug delivery systems and the blood-brain barrier. Int J Nanomedicine 9:795–811.
- Ambudkar SV, Lelong IH, Zhang J, et al. (1992). Partial purification and reconstitution of the human multidrug-resistance pump: characterization of the drug-stimulatable ATP hydrolysis. Proc Natl Acad Sci USA 89:8472–6.
- An Y, Guo W, Li L, et al. (2015). Micheliolide derivative DMAMCL inhibits glioma cell growth in vitro and in vivo. PLoS One 10:e0116202.
- And SAH, Pennington LD. (2006). Structure-brain exposure relationships. J Med Chem 49:7559–83.
- Aryal M, Vykhodtseva N, Zhang YZ, et al. (2013). Multiple treatments with liposomal doxorubicin and ultrasound-induced disruption of blood-tumor and blood-brain barriers improve outcomes in a rat glioma model. J Control Release 169:103–11.
- Asproni B, Murineddu G, Pau A, et al. (2011). Synthesis and SAR study of new phenylimidazole-pyrazolo[1,5-c]quinazolines as potent phosphodiesterase 10A inhibitors. Bioorg Med Chem 19:642–9.
- Azad TD, Pan J, Connolly ID, et al. (2015). Therapeutic strategies to improve drug delivery across the blood-brain barrier. Neurosurg Focus 38:E9.
- Ballabh P, Braun A, Nedergaard M. (2004). The blood-brain barrier: an overview: structure, regulation, and clinical implications. Neurobiol Dis 16:1–13.
- Bankstahl JP, Bankstahl M, Römermann K, et al. (2013). Tariquidar and elacridar are dose-dependently transported by P-glycoprotein and Bcrp at the blood-brain barrier: a small-animal positron emission tomography and in vitro study. Drug Metab Dispos 41:754–62.
- Bauer HC, Krizbai IA, Bauer H, Traweger A. (2014). "You Shall Not Pass"-tight junctions of the blood brain barrier. Front Neurosci 8:392.
- Beccaria K, Canney M, Goldwirt L, et al. (2016). Ultrasound-induced opening of the blood-brain barrier to enhance temozolomide and irinotecan delivery: an experimental study in rabbits. J Neurosurg 124:1602–10.
- Beyer CF, Zhang N, Hernandez R, et al. (2008). TTI-237: a novel microtubule-active compound with in vivo antitumor activity. Cancer Res 68:2292–300.
- Bhowmik A, Khan R, Ghosh MK. (2015). Blood brain barrier: a challenge for effectual therapy of brain tumors. Biomed Res Int 2015:320941.
- Birngruber T, Raml R, Gladdines W, et al. (2014). Enhanced doxorubicin delivery to the brain administered through glutathione PEGylated liposomal doxorubicin (2B3-101) as compared with generic Caelyx,(®)/Doxil(®)–a cerebral open flow microperfusion pilot study. J Pharm Sci 103:1945–8.
- Borlongan CV, Emerich DF. (2003). Facilitation of drug entry into the CNS via transient permeation of blood brain barrier: laboratory and preliminary clinical evidence from bradykinin receptor agonist, Cereport. Brain Res Bull 60:297–306.
- Bradley MO, Webb NL, Anthony FH, et al. (2001). Tumor targeting by covalent conjugation of a natural fatty acid to paclitaxel. Clin Cancer Res 7:3229–38.
- Bronger H, König J, Kopplow K, et al. (2005). ABCC drug efflux pumps and organic anion uptake transporters in human gliomas and the blood-tumor barrier. Cancer Res 65:11419–28.
- Burkhardt JK, Riina H, Shin BJ, et al. (2012). Intra-arterial delivery of bevacizumab after blood-brain barrier disruption for the treatment of recurrent glioblastoma: progression-free survival and overall survival. World Neurosurg 77:130–34.
- Butini S, Budriesi R, Hamon M, et al. (2009). Novel, potent, and selective quinoxaline-based 5-HT(3) receptor ligands. 1. Further structure-activity relationships and pharmacological characterization. J Med Chem 52:6946–50.
- Caliş U, Septioğlu E, Aytemir MD. (2011). Synthesis and anticonvulsant evaluation of some novel (thio)semicarbazone derivatives of arylalkylimidazole. Arzneimittelforschung 61:327–34.
- Cantanhede IG, de Oliveira J. (2017). PDGF family expression in glioblastoma multiforme: data compilation from ivy glioblastoma atlas project database. Sci Rep 7:15271.
- Carpentier A, Chauvet D, Reina V, et al. (2012). MR-guided laser-induced thermal therapy (LITT) for recurrent glioblastomas. Lasers Surg Med 44:361–8.
- Chamberlain MC, Glantz MJ, Chalmers L, et al. (2007). Early necrosis following concurrent Temodar and radiotherapy in patients with glioblastoma. J Neurooncol 82:81–3.
- Chen D, Shen A, Li J, et al. (2014). Discovery of potent N-(isoxazol-5-yl)amides as HSP90 inhibitors. Eur J Med Chem 87:765–81.
- Chen H, Konofagou EE. (2014). The size of blood-brain barrier opening induced by focused ultrasound is dictated by the acoustic pressure. J Cereb Blood Flow Metab 34:1197–204.
- Cheshier SH, Kalani MY, Lim M, et al. (2009). A neurosurgeon's guide to stem cells, cancer stem cells, and brain tumor stem cells. Neurosurgery 65:237–49. discussion 249–50; quiz N6.
- Chiriano G, De SA, Mancini F, et al. (2012). A small chemical library of 2-aminoimidazole derivatives as BACE-1 inhibitors: structure-based design, synthesis, and biological evaluation. Eur J Med Chem 48:206–13.
- Coluccia D, Fandino J, Schwyzer L, et al. (2014). First noninvasive thermal ablation of a brain tumor with MR-guided focused ultrasound. J Ther Ultrasound 2:17.
- Daneman R, Prat A. (2015). The blood-brain barrier. Cold Spring Harb Perspect Biol 7:a020412.
- Davis ME. (2016). Glioblastoma: overview of disease and treatment. Clin J Oncol Nurs 20:S2.
- de Vries NA, Zhao J, Kroon E, et al. (2007). P-glycoprotein and breast cancer resistance protein: two dominant transporters working together in limiting the brain penetration of topotecan. Clin Cancer Res 13:6440–49.
- Deeken JF, Löscher W. (2007). The blood-brain barrier and cancer: transporters, treatment, and Trojan horses. Clin Cancer Res 13:1663–74.
- Demeule M, Régina A, Jodoin J, et al. (2002). Drug transport to the brain: key roles for the efflux pump P-glycoprotein in the blood-brain barrier. Vascul Pharmacol 38:339–48.
- Doolittle ND, Miner ME, Hall WA, et al. (2000). Safety and efficacy of a multicenter study using intraarterial chemotherapy in conjunction with osmotic opening of the blood-brain barrier for the treatment of patients with malignant brain tumors. Cancer 88:637–47.
- Doyle L, Ross DD. (2003). Multidrug resistance mediated by the breast cancer resistance protein BCRP (ABCG2). Oncogene 22:7340–58.
- Drion N, Lemaire M, Lefauconnier JM, Scherrmann JM. (1996). Role of P-glycoprotein in the blood-brain transport of colchicine and vinblastine. J Neurochem 67:1688–93.
- Dréan A, Goldwirt L, Verreault M, et al. (2016). Blood-brain barrier, cytotoxic chemotherapies and glioblastoma. Expert Rev Neurother 16:1285–300.
- Durmus S, Sparidans RW, Wagenaar E, et al. (2012). Oral availability and brain penetration of the B-RAFV600E inhibitor vemurafenib can be enhanced by the P-GLYCOprotein (ABCB1) and breast cancer resistance protein (ABCG2) inhibitor elacridar. Mol Pharm 9:3236–45.
- Erdlenbruch B, Alipour M, Fricker G, et al. (2003). Alkylglycerol opening of the blood-brain barrier to small and large fluorescence markers in normal and C6 glioma-bearing rats and isolated rat brain capillaries. Br J Pharmacol 140:1201–10.
- Fernandes G, Fernandes BC, Valente V, Dos Santos JL. (2019). Recent advances in the discovery of small molecules targeting glioblastoma. Eur J Med Chem 164:8–26.
- Fung KL, Pan J, Ohnuma S, et al. (2014). MDR1 synonymous polymorphisms alter transporter specificity and protein stability in a stable epithelial monolayer. Cancer Res 74:598–608.
- Gaillard PJ, Appeldoorn CC, Dorland R, et al. (2014). Pharmacokinetics, brain delivery, and efficacy in brain tumor-bearing mice of glutathione pegylated liposomal doxorubicin (2B3-101). PLoS One 9:e82331.
- Gerber NU, Mynarek M, von Hoff K, et al. (2014). Recent developments and current concepts in medulloblastoma. Cancer Treat Rev 40:356–65.
- Giepmans BN, van Ijzendoorn SC. (2009). Epithelial cell-cell junctions and plasma membrane domains. Biochim Biophys Acta 1788:820–31.
- Golden EB, Cho HY, Hofman FM, et al. (2015). Quinoline-based antimalarial drugs: a novel class of autophagy inhibitors. Neurosurg Focus 38:E12.
- Goldwirt L, Beccaria K, Carpentier A, et al. (2014). Irinotecan and temozolomide brain distribution: a focus on ABCB1. Cancer Chemother Pharmacol 74:185–93.
- Goldwirt L, Zahr N, Farinotti R, Fernandez C. (2013). Development of a new UPLC-MSMS method for the determination of temozolomide in mice: application to plasma pharmacokinetics and brain distribution study. Biomed Chromatogr 27:889–93.
- Guillaume DJ, Doolittle ND, Gahramanov S, et al. (2010). Intra-arterial chemotherapy with osmotic blood-brain barrier disruption for aggressive oligodendroglial tumors: results of a phase I study. Neurosurgery 66:48–58. discussion 58.
- Hammarström LG, Harmel RK, Granath M, et al. (2016). The oncolytic efficacy and in vivo pharmacokinetics of [2-(4-chlorophenyl)quinolin-4-yl](piperidine-2-yl)methanol (vacquinol-1) are governed by distinct stereochemical features. J Med Chem 59:8577–92.
- Hardell L, Carlberg M, Hansson Mild K. (2009). Epidemiological evidence for an association between use of wireless phones and tumor diseases. Pathophysiology 16:113–22.
- Hartz AM, Bauer B. (2011). ABC transporters in the CNS - an inventory. Curr Pharm Biotechnol 12:656–73.
- Hawasli AH, Kim AH, Dunn GP, et al. (2014). Stereotactic laser ablation of high-grade gliomas. Neurosurg Focus 37:E1.
- Hediger MA, Romero MF, Peng JB, et al. (2004). The ABCs of solute carriers: physiological, pathological and therapeutic implications of human membrane transport proteinsIntroduction. Pflugers Arch 447:465–68.
- Hendricks BK, Cohen-Gadol AA, Miller JC. (2015). Novel delivery methods bypassing the blood-brain and blood-tumor barriers. Neurosurg Focus 38:E10.
- Hollenstein K, Dawson RJ, Locher KP. (2007). Structure and mechanism of ABC transporter proteins. Curr Opin Struct Biol 17:412–18.
- Hou LC, Veeravagu A, Hsu AR, Tse VC. (2006). Recurrent glioblastoma multiforme: a review of natural history and management options. Neurosurg Focus 20:E5.
- Huang Y, Sadée W. (2006). Membrane transporters and channels in chemoresistance and -sensitivity of tumor cells. Cancer Lett 239:168–82.
- Hynynen K, McDannold N, Vykhodtseva N, Jolesz FA. (2001). Noninvasive MR imaging-guided focal opening of the blood-brain barrier in rabbits. Radiology 220:640–6.
- Hülper P, Veszelka S, Walter FR, et al. (2013). Acute effects of short-chain alkylglycerols on blood-brain barrier properties of cultured brain endothelial cells. Br J Pharmacol 169:1561–73.
- Ishikawa M, Watanabe T, Kudo T, et al. (2010). Investigation of the histamine H3 receptor binding site. Design and synthesis of hybrid agonists with a lipophilic side chain. J Med Chem 53:6445–56.
- Jacobs S, McCully CL, Murphy RF, et al. (2010). Extracellular fluid concentrations of cisplatin, carboplatin, and oxaliplatin in brain, muscle, and blood measured using microdialysis in nonhuman primates. Cancer Chemother Pharmacol 65:817–24.
- Jalali S, Huang Y, Dumont DJ, Hynynen K. (2010). Focused ultrasound-mediated bbb disruption is associated with an increase in activation of AKT: experimental study in rats. BMC Neurol 10:114.
- Jue TR, McDonald KL. (2016). The challenges associated with molecular targeted therapies for glioblastoma. J Neurooncol 127:427–34.
- Karim R, Palazzo C, Evrard B, Piel G. (2016). Nanocarriers for the treatment of glioblastoma multiforme: current state-of-the-art. J Control Release 227:23–37.
- Kemper EM, Boogerd W, Thuis I, et al. (2004). Modulation of the blood-brain barrier in oncology: therapeutic opportunities for the treatment of brain tumours. Cancer Treat Rev 30:415–23.
- Kemper EM, van Zandbergen AE, Cleypool C, et al. (2003). Increased penetration of paclitaxel into the brain by inhibition of P-Glycoprotein. Clin Cancer Res 9:2849–55.
- Kemper EM, Verheij M, Boogerd W, et al. (2004). Improved penetration of docetaxel into the brain by co-administration of inhibitors of P-glycoprotein. Eur J Cancer 40:1269–74.
- Khosla D. (2016). Concurrent therapy to enhance radiotherapeutic outcomes in glioblastoma. Ann Transl Med 4:54.
- Kim KH, Kim D, Park JY, et al. (2015). NNC 55-0396, a T-type Ca2+ channel inhibitor, inhibits angiogenesis via suppression of hypoxia-inducible factor-1α signal transduction. J Mol Med 93:499–509.
- Kim YS, Rhim H, Choi MJ, et al. (2008). High-intensity focused ultrasound therapy: an overview for radiologists. Korean J Radiol 9:291–302.
- Kruh GD, Belinsky MG. (2003). The MRP family of drug efflux pumps. Oncogene 22:7537–52.
- Lemasson B, Serduc R, Maisin C, et al. (2010). Monitoring blood-brain barrier status in a rat model of glioma receiving therapy: dual injection of low-molecular-weight and macromolecular MR contrast media. Radiology 257:342–52.
- Leweke F, Damian MS, Schindler C, Schachenmayr W. (1998). Multidrug resistance in glioblastoma. Chemosensitivity testing and immunohistochemical demonstration of P-glycoprotein. Pathol Res Pract 194:149–55.
- Liebner S, Fischmann A, Rascher G, et al. (2000). Claudin-1 and claudin-5 expression and tight junction morphology are altered in blood vessels of human glioblastoma multiforme. Acta Neuropathol 100:323–31.
- Lim M, Xia Y, Bettegowda C, Weller M. (2018). Current state of immunotherapy for glioblastoma. Nat Rev Clin Oncol 15:422–42.
- Lin F, de Gooijer MC, Hanekamp D, et al. (2013). Targeting core (mutated) pathways of high-grade gliomas: challenges of intrinsic resistance and drug efflux. CNS Oncol 2:271–88.
- Lin F, de Gooijer MC, Roig EM, et al. (2014). ABCB1, ABCG2, and PTEN determine the response of glioblastoma to temozolomide and ABT-888 therapy. Clin Cancer Res 20:2703–13.
- Lin F, Marchetti S, Pluim D, et al. (2013). Abcc4 together with abcb1 and abcg2 form a robust cooperative drug efflux system that restricts the brain entry of camptothecin analogues. Clin Cancer Res 19:2084–95.
- Lipinski CA, Lombardo F, Dominy BW, Feeney PJ. (2001). Experimental and computational approaches to estimate solubility and permeability in drug discovery and development settings. Adv Drug Deliv Rev 46:3–26.
- Lipinski CA, Lombardo F, Dominy BW, Feeney PJ. (2012). Experimental and computational approaches to estimate solubility and permeability in drug discovery and development settings. Adv Drug Deliv Rev 64:4–17.
- Liu HL, Huang CY, Chen JY, et al. (2014). Pharmacodynamic and therapeutic investigation of focused ultrasound-induced blood-brain barrier opening for enhanced temozolomide delivery in glioma treatment. PLoS One 9:e114311.
- Lopez-Ramirez MA, Fischer R, Torres-Badillo CC, et al. (2012). Role of caspases in cytokine-induced barrier breakdown in human brain endothelial cells. J Immunol 189:3130–9.
- Mao Q, Unadkat JD. (2005). Role of the breast cancer resistance protein (ABCG2) in drug transport. Aaps J 7:E118–33.
- Mikitsh JL, Chacko AM. (2014). Pathways for small molecule delivery to the central nervous system across the blood-brain barrier. Perspect Medicin Chem 6:11–24.
- Miller G. (2002). Drug targeting. Breaking down barriers. Science 297:1116–8.
- Minocha M, Khurana V, Qin B, et al. (2012). Co-administration strategy to enhance brain accumulation of vandetanib by modulating P-glycoprotein (P-gp/Abcb1) and breast cancer resistance protein (Bcrp1/Abcg2) mediated efflux with m-TOR inhibitors. Int J Pharm 434:306–14.
- Miranda A, Blanco-Prieto M, Sousa J, et al. (2017a). Breaching barriers in glioblastoma. Part I: molecular pathways and novel treatment approaches. Int J Pharm 531:372–88.
- Miranda A, Blanco-Prieto MJ, Sousa J, et al. (2017b). Breaching barriers in glioblastoma. Part II: targeted drug delivery and lipid nanoparticles. Int J Pharm 531:389–410.
- Moitra K, Dean M. (2011). Evolution of ABC transporters by gene duplication and their role in human disease. Biol Chem 392:29–37.
- Molina-Arcas M, Casado FJ, Pastor-Anglada M. (2009). Nucleoside transporter proteins. Curr Vasc Pharmacol 7:426–34.
- Munoz JL, Rodriguez-Cruz V, Greco SJ, et al. (2014). Temozolomide induces the production of epidermal growth factor to regulate MDR1 expression in glioblastoma cells. Mol Cancer Ther 13:2399–411.
- Newton HB. (2012). Neurological complications of chemotherapy to the central nervous system. Handb Clin Neurol 105:903–16.
- Norman MH, Andrews KL, Bo YY, et al. (2012). Selective class I phosphoinositide 3-kinase inhibitors: optimization of a series of pyridyltriazines leading to the identification of a clinical candidate, AMG 511. J Med Chem 55:7796–816.
- Obermeier B, Daneman R, Ransohoff RM. (2013). Development, maintenance and disruption of the blood-brain barrier. Nat Med 19:1584–96.
- Oberoi RK, Parrish KE, Sio TT, et al. (2016). Strategies to improve delivery of anticancer drugs across the blood-brain barrier to treat glioblastoma. Neuro-oncology 18:27–36.
- Ostermann S, Csajka C, Buclin T, et al. (2004). Plasma and cerebrospinal fluid population pharmacokinetics of temozolomide in malignant glioma patients. Clin Cancer Res 10:3728–36.
- Ostrom QT, Bauchet L, Davis FG, et al. (2014). The epidemiology of glioma in adults: a "state of the science" review. Neuro Oncol 16:896–913.
- Ostrom QT, Gittleman H, Liao P, et al. (2014). CBTRUS statistical report: primary brain and central nervous system tumors diagnosed in the United States in 2007-2011. Neuro Oncol 16:iv1–63.
- Owonikoko TK, Arbiser J, Zelnak A, et al. (2014). Current approaches to the treatment of metastatic brain tumours. Nat Rev Clin Oncol 11:203–22.
- Pardridge WM. (2003). Blood-brain barrier drug targeting: the future of brain drug development. Mol Interv 3:90–105, 51.
- Pardridge WM. (2005). The blood-brain barrier: bottleneck in brain drug development. NeuroRx 2:3–14.
- Patel RR, Mehta MP. (2007). Targeted therapy for brain metastases: improving the therapeutic ratio. Clin Cancer Res 13:1675–83.
- Pinheiro T, Otrocka M, Seashore-Ludlow B, et al. (2017). A chemical screen identifies trifluoperazine as an inhibitor of glioblastoma growth. Biochem Biophys Res Commun 494:477–83.
- Planting AS, Sonneveld P, van der Gaast A, et al. (2005). A phase I and pharmacologic study of the MDR converter GF120918 in combination with doxorubicin in patients with advanced solid tumors. Cancer Chemother Pharmacol 55:91–9.
- Plate KH, Scholz A, Dumont DJ. (2012). Tumor angiogenesis and anti-angiogenic therapy in malignant gliomas revisited. Acta Neuropathol 124:763–75.
- Portnow J, Badie B, Chen M, et al. (2009). The neuropharmacokinetics of temozolomide in patients with resectable brain tumors: potential implications for the current approach to chemoradiation. Clin Cancer Res 15:7092–8.
- Potschka H, Fedrowitz M, Löscher W. (2003). Multidrug resistance protein MRP2 contributes to blood-brain barrier function and restricts antiepileptic drug activity. J Pharmacol Exp Ther 306:124–31.
- Pourgholi F, Hajivalili M, Farhad JN, et al. (2016). Nanoparticles: novel vehicles in treatment of glioblastoma. Biomed Pharmacother 77:98–107.
- Reyderman L, Statkevich P, Thonoor CM, et al. (2004). Disposition and pharmacokinetics of temozolomide in rat. Xenobiotica 34:487–500.
- Rodriguez A, Tatter SB, Debinski W. (2015). Neurosurgical techniques for disruption of the blood-brain barrier for glioblastoma treatment. Pharmaceutics 7:175–87.
- Sane R, Wu SP, Zhang R, Gallo JM. (2014). The effect of ABCG2 and ABCC4 on the pharmacokinetics of methotrexate in the brain. Drug Metab Dispos 42:537–40.
- Sarkaria JN, Hu LS, Parney IF, et al. (2018). Is the blood-brain barrier really disrupted in all glioblastomas? A critical assessment of existing clinical data. Neuro-oncology 20:184–91.
- Sekine T, Cha SH, Endou H. (2000). The multispecific organic anion transporter (OAT) family. Pflugers Arch 440:337–50.
- Sepulveda-Sanchez J, Ramos A, Hilario A, et al. (2015). Brain perfusion and permeability in patients with advanced, refractory glioblastoma treated with lomustine and the transforming growth factor-β receptor I kinase inhibitor LY2157299 monohydrate. Oncol Lett 9:2442–8.
- Sheikov N, McDannold N, Vykhodtseva N, et al. (2004). Cellular mechanisms of the blood-brain barrier opening induced by ultrasound in presence of microbubbles. Ultrasound Med Biol 30:979–89.
- Sloan AE, Ahluwalia MS, Valerio-Pascua J, et al. (2013). Results of the neuroblate system first-in-humans phase I clinical trial for recurrent glioblastoma: clinical article. J Neurosurg 118:1202–19.
- Slot AJ, Molinski SV, Cole S. (2011). Mammalian multidrug-resistance proteins (MRPs). Essays Biochem 50:179–207.
- Sminia P, Westerman BA. (2016). Blood-brain barrier crossing and breakthroughs in glioblastoma therapy. Br J Clin Pharmacol 81:1018–20.
- Soontornmalai A, Vlaming ML, Fritschy JM. (2006). Differential, strain-specific cellular and subcellular distribution of multidrug transporters in murine choroid plexus and blood-brain barrier. Neuroscience 138:159–69.
- Stamatovic SM, Keep RF, Andjelkovic AV. (2008). Brain endothelial cell-cell junctions: how to "open" the blood brain barrier. Curr Neuropharmacol 6:179–92.
- Staud F, Pavek P. (2005). Breast cancer resistance protein (BCRP/ABCG2). Int J Biochem Cell Biol 37:720–5.
- Taal W, Oosterkamp HM, Walenkamp AM, et al. (2014). Single-agent bevacizumab or lomustine versus a combination of bevacizumab plus lomustine in patients with recurrent glioblastoma (BELOB trial): a randomised controlled phase 2 trial. Lancet Oncol 15:943–53.
- Thomsen LB, Lichota J, Eskehave TN, et al. (2012). Brain delivery systems via mechanism independent of receptor-mediated endocytosis and adsorptive-mediated endocytosis. Curr Pharm Biotechnol 13:2349–54.
- Ting CY, Fan CH, Liu HL, et al. (2012). Concurrent blood-brain barrier opening and local drug delivery using drug-carrying microbubbles and focused ultrasound for brain glioma treatment. Biomaterials 33:704–12.
- Van AJ, Van TO, Tijssen F, et al. (1999). Increased accumulation of doxorubicin and doxorubicinol in cardiac tissue of mice lacking mdr1a P-glycoprotein. Br J Cancer 79:108–13.
- van Tellingen O, Yetkin-Arik B, de Gooijer MC, et al. (2015). Overcoming the blood-brain tumor barrier for effective glioblastoma treatment. Drug Resist Updat 19:1–12.
- VanBavel E. (2007). Effects of shear stress on endothelial cells: possible relevance for ultrasound applications. Prog Biophys Mol Biol 93:374–83.
- Venugopal C, Hallett R, Vora P, et al. (2015). Pyrvinium targets CD133 in human glioblastoma brain tumor-initiating cells. Clin Cancer Res 21:5324–37.
- Wager TT, Hou X, Verhoest PR, Villalobos A. (2010). Moving beyond rules: the development of a central nervous system multiparameter optimization (CNS MPO) approach to enable alignment of druglike properties. ACS Chem Neurosci 1:435–49.
- Wei KC, Chu PC, Wang HY, et al. (2013). Focused ultrasound-induced blood-brain barrier opening to enhance temozolomide delivery for glioblastoma treatment: a preclinical study. PLoS One 8:e58995.
- Weidle UH, Niewöhner J, Tiefenthaler G. (2015). The blood-brain barrier challenge for the treatment of brain cancer, secondary brain metastases, and neurological diseases. Cancer Genomics Proteomics 12:167–77.
- Wen PY, Lee EQ, Reardon DA, et al. (2012). Current clinical development of PI3K pathway inhibitors in glioblastoma. Neuro-oncology 14:819–29.
- Westerhout J, van den Berg D-J, Hartman R, et al. (2014). Prediction of methotrexate CNS distribution in different species - influence of disease conditions. Eur J Pharm Sci 57:11–24.
- Winkler EA, Bell RD, Zlokovic BV. (2011). Central nervous system pericytes in health and disease. Nat Neurosci 14:1398–405.
- Wolburg H, Lippoldt A. (2002). Tight junctions of the blood-brain barrier: development, composition and regulation. Vascul Pharmacol 38:323–37.
- Wolburg H, Lippoldt A, Ebnet K. (2007). Tight junctions in the blood–brain barrier. In: Lajtha A., Reith M.E.A. (eds) Handbook of Neurochemistry and Molecular Neurobiology. Springer, Boston, MA
- Wolburg H, Wolburg-Buchholz K, Kraus J, et al. (2003). Localization of claudin-3 in tight junctions of the blood-brain barrier is selectively lost during experimental autoimmune encephalomyelitis and human glioblastoma multiforme. Acta Neuropathol 105:586–92.
- Wong AD, Ye M, Levy AF, et al. (2013). The blood-brain barrier: an engineering perspective. Front Neuroeng 6:7.
- Woodworth GF, Dunn GP, Nance EA, et al. (2014). Emerging insights into barriers to effective brain tumor therapeutics. Front Oncol 4:126.
- Yap TA, Walton MI, Hunter LJ, et al. (2011). Preclinical pharmacology, antitumor activity, and development of pharmacodynamic markers for the novel, potent AKT inhibitor CCT128930. Mol Cancer Ther 10:360–71.
- Yu AS, McCarthy KM, Francis SA, et al. (2005). Knockdown of occludin expression leads to diverse phenotypic alterations in epithelial cells. Am J Physiol, Cell Physiol 288:C1231–41.
- Zahonero C, Aguilera P, Ramírez-Castillejo C, et al. (2015). Preclinical test of dacomitinib, an irreversible EGFR inhibitor, confirms its effectiveness for glioblastoma. Mol Cancer Ther 14:1548–58.
- Zhang F, Xu CL, Liu CM. (2015). Drug delivery strategies to enhance the permeability of the blood-brain barrier for treatment of glioma. Drug Des Devel Ther 9:2089–100.
- Zhang Q, Lu Y, Ding Y, et al. (2012). Guaianolide sesquiterpene lactones, a source to discover agents that selectively inhibit acute myelogenous leukemia stem and progenitor cells. J Med Chem 55:8757–69.
- Zhang Y, Schuetz JD, Elmquist WF, Miller DW. (2004). Plasma membrane localization of multidrug resistance-associated protein homologs in brain capillary endothelial cells. J Pharmacol Exp Ther 311:449–55.
- Zhou Q, Gallo JM. (2009). Differential effect of sunitinib on the distribution of temozolomide in an orthotopic glioma model. Neuro Oncol 11:301–10.
- Łazewska D, Wiecek M, Ligneau X, et al. (2009). Histamine H3 and H4 receptor affinity of branched 3-(1H-imidazol-4-yl)propyl N-alkylcarbamates. Bioorg Med Chem Lett 19:6682–5.