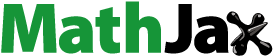
Abstract
Hepatocellular carcinoma (HCC) is the fourth leading cause of cancer-associated death worldwide. Beside early detection, early diagnosis, and early surgery, it is urgent to try new strategies for the treatment of HCC. Triptolide (TPL) has been employed to treat HCC. However, its clinical applications were restricted by the narrow therapeutic window, severe toxicity, and poor water-solubility. In this study, we developed cancer cell membrane-camouflaged biomimetic PLGA nanoparticles loading TPL (TPL@mPLGA) with the homologous targeting property for the treatment of HCC. The TPL@mPLGA was successfully prepared with particle size of 195.5 ± 7.5 nm and zeta potential at −21.5 ± 0.2 mV with good stability. The drug loading (DL) of TPL@mPLGA was 2.94%. After Huh-7 cell membrane coating, the natural Huh-7 cell membrane proteins were found to be retained on TPL@mPLGA, thus endowing the TPL@mPLGA with enhanced accumulation at tumor site, and better anti-tumor activity in vitro and in vivo when compared with TPL or TPL@PLGA. The TPL@mPLGA showed enhanced anti-tumor effects and reduced toxicity of TPL, which could be adopted for the treatment of HCC.
Introduction
Hepatocellular carcinoma (HCC), the most common form of primary liver cancer, is the fourth leading cause of cancer-associated death worldwide (Ganesan & Kulik, Citation2023). HCC is usually caused by viral hepatitis, cirrhosis, or nonalcoholic fatty liver disease. Surgical resection is preferable for HCC treatment. However, most patients are diagnosed at a late stage and considered inoperable for surgery. Many other strategies have been attempted for the treatment of HCC, such as chemoembolization, transarterial embolization and biotherapies (Forner et al., Citation2018; Yu et al., Citation2021). Unfortunately, the survival of HCC patients is still poor due to limitations of the above treatment strategies. In addition, conventional chemotherapy agents such as doxorubicin have not been shown to significantly prolong survival in patients with inoperable HCC (Ling et al., Citation2014). Sorafenib, an oral multikinase inhibitor which suppresses HCC angiogenesis and proliferation, was approved by the FDA as a first-line treatment for advanced HCC in 2007 (Liang et al., Citation2020). Nevertheless, the results of two phase III clinical trials showed that sorafenib could prolong the overall survival of HCC patients by 2–3 months. However, only 30% of patients benefited from sorafenib because drug resistance occurred within 6 months (Feng et al., Citation2020). Therefore, it is urgent to discover and develop more effective HCC treatment strategies.
In recent years, traditional Chinese medicine (TCM) has received increasing attention in cancer therapy due to its broad anticancer effects. Triptolide (TPL), a diterpenoid triepoxide derived from the Chinese herb Tripterygium wilfordii Hook.f., is a promising chemotherapeutic agent which can effectively inhibit the growth of malignant cancers including HCC (Liu et al., Citation2018). TPL, inducing apoptosis in cancer cells through cytochrome c transfer into the cytosol and inhibition of anti-apoptotic protein expression, was found to be more potent in cell models and animal models than doxorubicin, sorafenib, and other clinical first-line drugs against HCC (Sun et al., Citation2017; Zhao et al., Citation2019; Zheng et al., Citation2021). Nevertheless, TPL is highly toxic, with very limited water solubility, narrow therapeutic window, and low bioavailability, which has greatly restricted its clinical use. Over the past decades, various TPL delivery systems have been developed to enhance drug insolubility, alleviate the side effects, and improve the bioavailability of TPL, such as solid lipid nanoparticle, liposome, polymeric micelle, microemulsion, metal-organic framework (MOF), and so on (Zhu et al., Citation2022). However, the therapeutic outcomes of the delivery systems above in cancer therapy are still significantly limited owing to lack of active-targeting. Thus, it is imperious to design new targeted drug delivery systems to overcome the problems mentioned above. In recent years, the emergence of biomimetic nanoparticles has demonstrated considerable potential in improving circulation time, transporting across membranes, enhancing solubility and stability of loaded drugs. And different kinds of cell membranes, such as red blood cells (RBCs) membrane, cancer cell membrane, platelet membrane, stem cell membrane, macrophage membrane, or microorganism membrane have been exploited to develop biomimetic nanoparticles with different functions (Fang et al., Citation2018, Citation2023; Soprano et al., Citation2022). Among them, cancer cell membrane with special properties such as specific recognition, immune escaping and easy availability has attracted great interest in the biomimetic nanoparticle field (Chen et al., Citation2016; Xiong et al., Citation2021).
On the basis of the mature technology of commercial PLGA nanoparticles (Operti et al., Citation2021), we developed cancer cell membrane-camouflaged biomimetic PLGA nanoparticles loading TPL (TPL@mPLGA) for the treatment of HCC. We hypothesized that the TPL@mPLGA could accumulate at tumor site with the homologous targeting property, thus enhancing anti-tumor effects and reducing toxicity of TPL, which could serve as an excellent nanoplatform for the treatment of HCC.
Materials and methods
Chemicals, cells, and animals
TPL (purity ≥98%) was supplied by Shanghai Yuanye Biotech Co., Ltd. (Shanghai, China). Coumarin-6 (C6) was obtained from Sigma-Aldrich Co. (St. Louis, MO). PLGA (50:50, Mw = 50,000) was purchased from Jinan Daigang Biomaterial Co., Ltd (Jinan, China). Cell counting kit-8 (CCK-8) was obtained from Beyotime Biotechnology (Shanghai, China). Fetal bovine serum (FBS) and DMEM medium were bought from Gibco Inc. (Grand Island, NY). Polyvinyl alcohol (PVA) was purchased from Alfa Aesar Inc. (Ward Hill, MA). All other chemicals used were of analytical grade.
The Huh-7 cell line was purchased from the Cell Bank of Typical Culture Preservation Committee of the Chinese Academy of Sciences (Shanghai, China). Huh-7 cells were cultured in DMEM supplemented with 10% FBS and 1% penicillin/streptomycin at 37 °C in a 5% CO2 atmosphere.
The experiment was approved by the Animal Ethics Committee of Shanghai University of Traditional Chinese Medicine before the research. The number of the ethics approval was PZSHUTCM220919014. The animal experiments were conducted following ARRIVE guidelines. Twenty-three healthy Balb/c-nu mice (18 ± 2 g) were randomly assigned to different groups after successful construction of subcutaneously implanted tumor model. At the end of study, the animals were sacrificed with cervical dislocation method following anesthesia with isoflurane.
Methods
Preparation of TPL@mPLGA
TPL@mPLGA was prepared with previously published methods with some modifications (Jin et al., Citation2019; Wang et al., Citation2021). Briefly, 50 mg of PLGA and 2 mg of TPL were dissolved in trichloromethane as the organic phase. The mixture was emulsified with 10 mL of the PVA aqueous solution (1 wt%) using a probe sonication at 100 W for 10 minutes in ice bath. The resulting suspension was stirred for four hours at room temperature to evaporate the acetone and was subsequently centrifuged at 15,000 × g for 30 minutes, followed by washing three times with distilled water to remove the residual PVA and resuspending in PBS. The C6 or ICG labeled PLGA nanoparticles (C6@PLGA or ICG@PLGA) were prepared with the method described above except using 1 mg C6 instead of 2 mg TPL.
Cancer cell membranes were separated using protocols reported previously (Jin et al., Citation2019). To prepare TPL@mPLGA, C6@mPLGA, or ICG@mPLGA, cancer cell membrane and TPL@PLGA/C6@PLGA/ICG@PLGA were mixed with the mass ratio at 1:10 in PBS, followed by water bath sonication for 5 min at a power of 100 W. All the nanoparticles were stored at 4 °C for further use or freeze-drying.
Characterization of the preparations
The particle size and Zeta potential of the nanoparticles were detected by Nano ZS90 Zetasizer (Malvern Instruments, Malvern, UK). The morphology of the nanoparticles was characterized with a JEM-2100 transmission electron microscope (JEOL, Tokyo, Japan). Proteins on the Huh-7 cells, Huh-7 derived cell membrane, and TPL@mPLGA were characterized using SDS-PAGE gel electrophoresis (Zhang et al., Citation2021). The stability of TPL@mPLGA in plasma was studied. The drug loading (DL) was investigated directly by dissolving 4 mg nanoparticles in 1 mL DMSO, and the solution was diluted to be detectable with HPLC (Wang et al., Citation2021). The DL and encapsulation efficiency (EE) of TPL were calculated according to the following equations:
(1)
(1)
(2)
(2)
In vitro drug release
The drug release profiles of free TPL, TPL@PLGA, and TPL@mPLGA were evaluated by resuspending the nanoparticles in a dialysis bag and immersing in 200 mL release buffer (PBS containing 0.1% w/v Tween 80, pH 5.5). All experiments were performed at 37 °C with shaking (100 rpm) for 48 h. At 0.5, 1, 2, 4, 6, 12, 24, and 48 h, 1 mL of the external medium was collected and replaced with an equal volume of pre-heated fresh medium (Wang et al., Citation2019). The concentration of TPL in the samples was analyzed by HPLC.
In vitro cellular uptake studies
For cellular uptake study, free C6, C6@PLGA, and C6@mPLGA were prepared and their uptake by Huh-7 cells was evaluated by a Leica TCS-SP8 confocal laser scanning microscopy (CLSM) and a flow cytometry, respectively. For CLSM, 1 mL Huh-7 cells were seeded into confocal dishes at the density of 5 × 104 cells/mL. Twelve hours later, the cells were treated with free C6, C6@PLGA, or C6@mPLGA for 4 h. Thereafter, the cells were washed with PBS for three times, fixed with 4% paraformaldehyde for 20 min and stained with Hoechst 33342 for 10 min. Finally, the cells were washed with PBS for three times again and observed by CLSM (Lara-Cruz et al., Citation2019).
For flow cytometry analysis, 1 mL Huh-7 cells were seeded into a six-well plate at a density of 5 × 105 cells/mL overnight. Then, the culture medium was removed. Huh-7 cells were treated with free C6, C6@PLGA, or C6@mPLGA for 4 h. Then, the cells were washed with PBS for three times and collected after digesting with trypsin, followed by flow cytometry analysis.
Cytotoxicity
The cytotoxicity of free TPL, TPL@PLGA, and TPL@mPLGA was investigated with the CCK-8 assay. In brief, 100 μL Huh-7 cells were seeded into 96-well plates at a density of 5 × 104 cells/mL overnight. Then, the cells were treated with TPL, TPL@PLGA, and TPL@mPLGA. After 48 h, CCK-8 assay was applied to estimate the cytotoxicity based on the following equation:
(3)
(3)
where ODE, ODC, and ODB are the absorbance of experimental group, control group, and blank group, respectively. Calculations of the 50% inhibitive concentration (IC50) were performed on a CompuSyn software (Biosoft, Cambridge, UK).
Cell apoptosis study
To further investigate the mechanism of TPL@mPLGA in inhibiting cancer, 1 mL Huh-7 cells were seeded into a six-well plate at a density of 5 × 105 cells/mL overnight. Then, the culture medium was removed. Huh-7 cells were treated with free TPL, TPL@PLGA, and TPL@mPLGA. Forty-eight hours later, the cells were collected after digesting with trypsin without EDTA and stained with Annexin V-FITC and propidium iodide (PI). After resuspending in binding buffer, the samples were detected by a flow cytometry (Yuan et al., Citation2022).
In vivo biodistribution of the preparations
To investigate the in vivo distribution of the preparations, BALB/c nude mice were injected subcutaneously with 2 × 106 Huh-7 cells into the right axillary region. When the tumor volume reached about 100 mm3, the tumor bearing mice were i.v. injected with free ICG, ICG@PLGA, or ICG@mPLGA. The mice were then anesthetized and the in vivo biodistribution of the ICG-labeled preparations were imaged by an IVIS Lumina XR Imaging System at 2, 4, 6, and 24 h. Finally, the hearts, livers, spleens, lungs, kidneys, and tumors of the mice were harvested for fluorescence imaging (Zhu et al., Citation2017).
In vivo anti-tumor activity study of the preparations
As mentioned above, subcutaneous tumor model was successfully established. When the tumor volume reached about 50 mm3, the tumor bearing mice were i.v. injected with saline, TPL, TPL@PLGA, and TPL@mPLGA at a dose of 0.5 mg·kg−1 TPL every two days for 14 days. In the meantime, the body weights and tumor volumes of the tumor bearing mice were recorded. Two days after the last injection, the tumors were collected, weighed, photographed, and stained with H&E. The tumor growth inhibitory rate (IR) was calculated by the following equation:
(4)
(4)
where WB is the average tumor weight in saline group and WE is the tumor weight in experimental group, respectively.
Statistical analysis
Data were expressed as mean ± SD. Differences among groups were performed with a one-way analysis of variance (ANOVA) test. p < .05 was considered statistically significant.
Results and discussion
Characterization of the preparations
The TPL@PLGA was prepared with emulsion solvent evaporation process. To realize the homologous targeting, Huh-7 cell membrane was camouflaged to form TPL@mPLGA for the treatment of HCC. The particle sizes of TPL@PLGA and TPL@mPLGA were 179.3 ± 4.5 and 195.5 ± 7.5 nm, and Zeta potentials of TPL@PLGA and TPL@mPLGA were −10.8 ± 1.3 and −21.5 ± 0.2 mV (). As shown in , the particle size of TPL@mPLGA did not change significantly after 72 h in plasma, indicating that the preparation had good stability and no precipitation or aggregation occurred. The DL of TPL in TPL@PLGA and TPL@mPLGA was 3.06% and 2.94%, respectively. The EE of TPL in TPL@PLGA and TPL@mPLGA was 79.7% and 76.5%, respectively. TEM images demonstrated that TPL@mPLGA exhibited a typical core–shell structure and were spherical with good monodispersity (). The increased particle size and decreased Zeta potential confirmed the successful coating of Huh-7 cell membrane with negative-charged and thickness of about 10 nm (Zhang et al., Citation2021). SDS-PAGE gel electrophoresis was used to verify whether the membrane proteins would be damaged during the preparation process of Huh-7 cell membrane and TPL@mPLGA. SDS-PAGE gel electrophoresis of Huh-7 cells, Huh-7 derived cell membrane and TPL@mPLGA presented same protein bands (), indicating that TPL@mPLGA retained the natural Huh-7 cell membrane proteins during the preparation process and that the Huh-7 cell membrane was successfully coated onto the nanoparticles.
Figure 1. Characterization and in vitro drug release of the nanoparticles: (A) the size distribution of TPL@PLGA and TPL@mPLGA; (B) the Zeta potentials of TPL@PLGA and TPL@mPLGA; (C) stability of (SFN + TPL)@LCNPs and (SFN + TPL)@CPLCNPs in plasma; (D) the morphology of TPL@PLGA and TPL@mPLGA; (E) the SDS-PAGE protein expression analysis of 1: Marker; 2: Huh-7 cells; 3: Huh-7 derived cell membrane; 4: TPL@mPLGA; (F) the in vitro release curves of TPL from free TPL, TPL@PLGA, and TPL@mPLGA (n = 3; **p < .01; *p < .05).
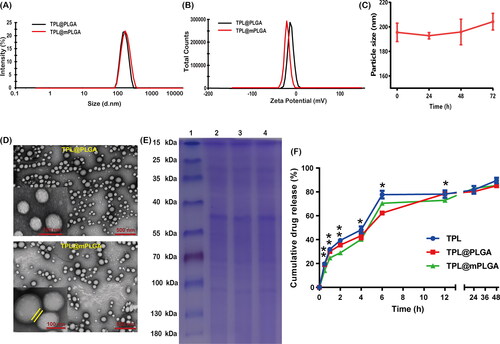
In vitro drug release
In vitro drug release assays of free TPL, TPL@PLGA, and TPL@mPLGA were conducted in 200 mL PBS containing 0.1% w/v Tween 80 at pH 5.5 (simulating the slightly acidic tumor microenvironment). The cumulative release rates of TPL from free TPL, TPL@PLGA, and TPL@mPLGA did not show much difference (). However, the release of TPL from TPL@mPLGA demonstrated significantly slower drug release (72.97%) than from free TPL (78.09%) or TPL@PLGA (78.43%) in the first 12 hours, which might be attributed to the coating of membrane alleviating the release of drug from mPLGA to some extent. Similar results were also seen in in vitro release of other biomimetic PLGA nanoparticles (Su et al., Citation2018; Wu et al., Citation2021).
In vitro cellular uptake studies
To evaluate the homologous tumor targeting effects of nanoparticles in vitro, CLSM and flow cytometry were adopted to evaluate the cellular uptake of free C6, C6@PLGA, and C6@mPLGA by Huh-7 cells. As shown in , the fluorescence intensity of cells in the C6@mPLGA group was much higher than that of free C6 and C6@PLGA group. The results of quantified flow cytometry analysis also presented similar tendency with CLSM. The cellular uptake of C6@mPLGA (97.18%) by Huh-7 cells was also higher than that of free C6, C6@PLGA (42.50% and 54.55%, respectively) (). This suggested that homologous tumor targeting took place between Huh-7 cells and cell membrane-camouflaged C6@mPLGA.
Cytotoxicity and cell apoptosis study
The in vitro cytotoxicity of free TPL, TPL@PLGA, and TPL@mPLGA in Huh-7 cells was investigated. All preparations showed dose-dependent inhibitory activities in Huh-7 cells (). The IC50 of free TPL, TPL@PLGA, and TPL@mPLGA in Huh-7 cells was 23.51 nM, 19.81 nM, and 12.03 nM, respectively. The TPL@mPLGA was found to be more toxic toward Huh-7 cells compared with free TPL and TPL@PLGA, indicating that cancer cell membrane played an important role in the enhancement of in vitro cytotoxicity. TPL can induce apoptosis through the mitochondrial pathway in Huh-7 cells (Zheng et al., Citation2021). The Annexin V-FITC/PI double staining was adopted to investigate the ability of different preparations in inducing Huh-7 cell apoptosis. The results showed that the percentage of early and late apoptotic cells in TPL@mPLGA group was significantly higher (p < .01) than that of free TPL and TPL@PLGA (). These results demonstrated that the cancer cell membrane camouflaged nanoparticles showed better anti-tumor effects in vitro, which might be related to the higher uptake of membrane camouflaged nanoparticles by Huh-7 cells, thus presenting higher cell apoptosis rate and cytotoxicity.
Figure 3. In vitro anti-tumor activity of different formulations: (A) cell viability of Huh-7 cells after treated with different formulations; (B) the statistical results of Huh-7 cell apoptosis rate; (C) induction of apoptosis in Huh-7 cells treated with various formulations tested by flow cytometer (n = 3; **p < .01).
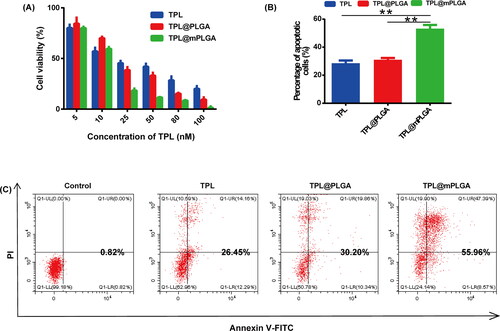
In vivo biodistribution of the preparations
To evaluate the homologous tumor targeting effect of nanoparticles in vivo, the biodistribution of ICG-labeled preparations after i.v. injection was imaged. As shown in , the florescence intensity of ICG in ICG@PLGA or ICG@mPLGA group at the tumor site was higher than that of the free ICG group. Notably, the fluorescence intensity of the ICG@mPLGA group was the highest, which further confirmed that ICG@mPLGA could accumulate at tumor site via the homologous tumor targeting and drug could be successfully delivered into the tumor tissues by loading into mPLGA.
In vivo anti-tumor activity study of the preparations
The preparations including saline, TPL, TPL@PLGA, and TPL@mPLGA were administered via i.v. injection for a total of seven times in 14 days. In the observation period, no body weight decrease was witnessed (). However, a mouse in TPL group died after the fourth i.v. injection. As reported, TPL has narrow therapeutic window and multi-organ toxicity, including damage to the blood circulatory, bone marrow, urinary, and gastrointestinal systems (Zheng et al., Citation2019; Yalikong et al., Citation2021), which might explain the death of the mouse in TPL group and highlight the importance of targeted TPL delivery system. For in vivo anti-tumor study, the TPL@mPLGA significantly inhibited the tumor growth (), which could be attributed to the homologous tumor targeting of TPL@mPLGA. At the end of the treatment, the tumors were collected and weighted. TPL@mPLGA group showed superior anti-tumor efficacy () with significantly lower tumor weight (p < .05) when compared with saline, free TPL, or TPL@PLGA group. The tumor growth IR of TPL@mPLGA was 69.25%. Moreover, H&E staining of tumor tissues indicated that TPL@mPLGA and TPL@PLGA triggered extensive tumor necrosis, and free TPL could also induce somewhat tumor necrosis as compared with saline group (). These data showed that TPL@mPLGA had potent anti-tumor activity without obvious toxicity. The camouflaged Huh-7 cell membrane made more TPL@mPLGA accumulated at the tumor site with higher anti-tumor activity and lower toxicity.
Conclusions
To sum up, we developed TPL@mPLGA with the homologous targeting property for the treatment of HCC. The Huh-7 cell membrane was successfully camouflaged onto the TPL@PLGA, thus endowing the TPL@mPLGA with slower drug release behavior, enhanced accumulation at tumor site, and better anti-tumor activity in vitro and in vivo when compared with TPL or TPL@PLGA. This newly developed tumor targeted drug delivery system provides a promising approach for the treatment of HCC.
Author contributions
Y.D. and T.Z. designed and supervised the experiments. Z.L., J.L., and Y.W. conducted the experiments, analyzed the experimental data, and wrote the original draft. All authors have read and agreed to the published version of the manuscript and agreed to be accountable for all aspects of the work.
Ethical approval
The experiment was approved by the Animal Ethics Committee of Shanghai University of Traditional Chinese Medicine before the research. The number of the ethics approval was PZSHUTCM22091901. The animal experiments were conducted following ARRIVE guidelines. The 23 mice were used to study the in vivo biodistribution and anti-tumor effect of the preparations, which was essential for studying the characteristics of the preparation. All mice were housed in a specific pathogen free environment (ventilated room, 24 ± 2 °C, 55–65% humidity, 12 h light/dark cycle, and free access to water and food). At the end of study, the animals were sacrificed with cervical dislocation method following anesthesia with 1.5% isoflurane.
Consent form
Not applicable.
Disclosure statement
No potential conflict of interest was reported by the author(s).
Data availability statement
The data that support the findings of this study are available on request from the corresponding author.
Additional information
Funding
References
- Chen Z, Zhao P, Luo Z, et al. (2016). Cancer cell membrane-biomimetic nanoparticles for homologous-targeting dual-modal imaging and photothermal therapy. ACS Nano 10:10049–57. doi: 10.1021/acsnano.6b04695.
- Fang RH, Gao W, Zhang L. (2023). Targeting drugs to tumours using cell membrane-coated nanoparticles. Nat Rev Clin Oncol 20:33–48. doi: 10.1038/s41571-022-00699-x.
- Fang RH, Kroll AV, Gao W, et al. (2018). Cell membrane coating nanotechnology. Adv Mater 30:e1706759.
- Feng J, Dai W, Mao Y, et al. (2020). Simvastatin re-sensitizes hepatocellular carcinoma cells to sorafenib by inhibiting HIF-1alpha/PPAR-gamma/PKM2-mediated glycolysis. J Exp Clin Cancer Res 39:24. doi: 10.1186/s13046-020-1528-x.
- Forner A, Reig M, Bruix J. (2018). Hepatocellular carcinoma. Lancet 391:1301–14.
- Ganesan P, Kulik LM. (2023). Hepatocellular carcinoma: new developments. Clin Liver Dis 27:85–102. doi: 10.1016/j.cld.2022.08.004.
- Jin J, Krishnamachary B, Barnett JD, et al. (2019). Human cancer cell membrane-coated biomimetic nanoparticles reduce fibroblast-mediated invasion and metastasis and induce T-cells. ACS Appl Mater Interfaces 11:7850–61.
- Lara-Cruz C, Jimenez-Salazar JE, Arteaga M, et al. (2019). Gold nanoparticle uptake is enhanced by estradiol in MCF-7 breast cancer cells. Int J Nanomedicine 14:2705–18.
- Liang C, Dong Z, Cai X, et al. (2020). Hypoxia induces sorafenib resistance mediated by autophagy via activating FOXO3a in hepatocellular carcinoma. Cell Death Dis 11:1017. doi: 10.1038/s41419-020-03233-y.
- Ling D, Xia H, Park W, et al. (2014). pH-sensitive nanoformulated triptolide as a targeted therapeutic strategy for hepatocellular carcinoma. ACS Nano 8:8027–39. doi: 10.1021/nn502074x.
- Liu M, Song W, Du X, et al. (2018). NQO1-selective activated prodrug of triptolide: synthesis and antihepatocellular carcinoma activity evaluation. ACS Med Chem Lett 9:1253–7.
- Operti MC, Bernhardt A, Grimm S, et al. (2021). PLGA-based nanomedicines manufacturing: technologies overview and challenges in industrial scale-up. Int J Pharm 605:120807. doi: 10.1016/j.ijpharm.2021.120807.
- Soprano E, Polo E, Pelaz B, et al. (2022). Biomimetic cell-derived nanocarriers in cancer research. J Nanobiotechnol 20:538. doi: 10.1186/s12951-022-01748-4.
- Su J, Liu G, Lian Y, et al. (2018). Preparation and characterization of erythrocyte membrane cloaked PLGA/arsenic trioxide nanoparticles and evaluation of their in vitro anti-tumor effect. RSC Adv 8:20068–76.
- Sun YY, Xiao L, Wang D, et al. (2017). Triptolide inhibits viability and induces apoptosis in liver cancer cells through activation of the tumor suppressor gene p53. Int J Oncol 50:847–52. doi: 10.3892/ijo.2017.3850.
- Wang H, Wu J, Williams GR, et al. (2019). Platelet-membrane-biomimetic nanoparticles for targeted antitumor drug delivery. J Nanobiotechnol 17:60. doi: 10.1186/s12951-019-0494-y.
- Wang S, Jiang H, Wang J, et al. (2021). Superior in vitro anticancer effect of biomimetic paclitaxel and triptolide co-delivery system in gastric cancer. J Biomed Res 35:327–38. doi: 10.7555/JBR.35.20210102.
- Wu X, Li Y, Raza F, et al. (2021). Red blood cell membrane-camouflaged tedizolid phosphate-loaded PLGA nanoparticles for bacterial-infection therapy. Pharmaceutics 13:99. doi: 10.3390/pharmaceutics13010099.
- Xiong J, Wu M, Chen J, et al. (2021). Cancer-erythrocyte hybrid membrane-camouflaged magnetic nanoparticles with enhanced photothermal-immunotherapy for ovarian cancer. ACS Nano 15:19756–70. doi: 10.1021/acsnano.1c07180.
- Yalikong A, Li XQ, Zhou PH, et al. (2021). A triptolide loaded HER2-targeted nano-drug delivery system significantly suppressed the proliferation of HER2-positive and BRAF mutant colon cancer. Int J Nanomedicine 16:2323–35.
- Yu L, Wang Z, Mo Z, et al. (2021). Synergetic delivery of triptolide and Ce6 with light-activatable liposomes for efficient hepatocellular carcinoma therapy. Acta Pharm Sin B 11:2004–15.
- Yuan H, Xia P, Sun X, et al. (2022). Photothermal nanozymatic nanoparticles induce ferroptosis and apoptosis through tumor microenvironment manipulation for cancer therapy. Small 18:e2202161. doi: 10.1002/smll.202202161.
- Zhang Y, He Z, Li Y, et al. (2021). Tumor cell membrane-derived nano-Trojan horses encapsulating phototherapy and chemotherapy are accepted by homologous tumor cells. Mater Sci Eng C Mater Biol Appl 120:111670.
- Zhao X, Liu X, Zhang P, et al. (2019). Injectable peptide hydrogel as intraperitoneal triptolide depot for the treatment of orthotopic hepatocellular carcinoma. Acta Pharm Sin B 9:1050–60.
- Zheng W, Wang C, Ding R, et al. (2019). Triptolide-loaded nanoparticles targeting breast cancer in vivo with reduced toxicity. Int J Pharm 572:118721. doi: 10.1016/j.ijpharm.2019.118721.
- Zheng Y, Kong F, Liu S, et al. (2021). Membrane protein-chimeric liposome-mediated delivery of triptolide for targeted hepatocellular carcinoma therapy. Drug Deliv 28:2033–43.
- Zhu D, Zhang Q, Chen Y, et al. (2022). Mechanochemical preparation of triptolide-loaded self-micelle solid dispersion with enhanced oral bioavailability and improved anti-tumor activity. Drug Deliv 29:1398–408.
- Zhu L, Kalimuthu S, Gangadaran P, et al. (2017). Exosomes derived from natural killer cells exert therapeutic effect in melanoma. Theranostics 7:2732–45. doi: 10.7150/thno.18752.