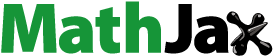
Abstract
Skin melanoma is considered the most dangerous form of skin cancer due to its association with high risk of metastasis, high mortality rate and high resistance to different treatment options. Genistein is a natural isoflavonoid with known chemotherapeutic activity. Unfortunately, it has low bioavailability due to its poor aqueous solubility and excessive metabolism. In the current study, genistein was incorporated into transferosomal hydrogel to improve its bioavailability. The prepared transferosomal formulations were characterized regarding: particle size; polydispersity index; zeta potential; encapsulation efficiency; TEM; FTIR; DSC; XRD; in vitro drug release; viscosity; pH; ex vivo anti-tumor activity on 3D skin melanoma spheroids and 1-year stability study at different storage temperatures. The optimized formulation has high encapsulation efficiency with an excellent particle size that will facilitate its penetration through the skin. The transfersomes have a spherical shape with sustained drug release profile. The anti-tumor activity evaluation of genistein transfersome revealed that genistein is a potent chemotherapeutic agent with enhanced penetration ability through the melanoma spheroids when incorporated into transfersomes. Stability study results demonstrate the high physical and chemical stability of our formulations. All these outcomes provide evidence that our genistein transferosomal hydrogel is a promising treatment option for skin melanoma.
1. Introduction
Skin melanoma is considered a prime global health challenge to researchers due to its high risk of metastasizing nature, drug resistance, amplified incidences, and concomitant mortality rate. Throughout most of the 20th century, the incidence rate has increased mostly with an anticipated annual growth of 3–7%. The imperative factors allied with the development of cancer diseases are related to exposure factors (e.g. sunlight exposure), stage of disease, genetic syndromes, number of tumors, and immunosuppression (Prajapat et al., Citation2023). Because melanoma is the most malignant invasive metastasis skin cancer that is generated by melanocytes, a localized treatment strategy becomes unproductive and tedious. Though chemotherapy, radiotherapy, and immunotherapy have stayed the cornerstone in the treatment outcomes of melanoma, major drawbacks of these options are instances of adverse toxic effects and inadequate localization of chemotherapeutic agents in addition to drug resistance to current immunosuppressive checkpoint inhibitors. The stroma cell barrier existence within the immunosuppressive microenvironment of the carcinoma is the primary trigger of drug resistance in melanoma (Mason et al., Citation2019). Similarly, the traditional therapy regimen for such malignant tumors has issues with its suboptimal therapeutic efficiency owing to off-target drug transport and inefficient internalization by target receptors on tumor cells. Thus, targeted nanomedicines that can provide site-specific delivery bypass such hurdles. Patients with melanoma are benefiting from better treatment outcomes thanks in large part to nanotechnology. Nanosized drug delivery systems are colloidal substances that include: inorganic; polymeric; lipidic; or hybrid particulate materials with nanometric dimension. Proteins, peptides, antibodies, nucleic acids, radiotherapeutics, and chemotherapeutic drugs can all be effectively transported by these particles (Meteoglu and Erdemir, Citation2021).
Potential phytonanomedicines are emerging sciences that affect cell signaling and are used as chemopreventive agents in cutaneous cancer treatment via enabled drug penetration and precise targeting interventions with mitigated adverse effects in case of topical application. In this regard, flavonoids are the most common polyphenols with broad treatment modalities such as antioxidant, antiviral, anti-inflammatory, antibacterial and anti-allergic effects. Numerous flavonoids hamper several signal transduction pathways that promote apoptosis, restrict angiogenesis and metastasis and limit cell proliferation (Meteoglu and Erdemir, Citation2021). Genistein (Gen) is the major isoflavone in human diets, profusely abundant in soybean foods. Numerous studies demonstrated that Gen offers great promise as chemotherapeutic agent that chiefly controls cellular events involving cell proliferation, migration, apoptotic cell death, metastasis and angiogenesis in different skin cancer types (Andrade et al., Citation2014). However, the poor aqueous solubility, rapid metabolism and excretion and unfavorable bioavailability are considered the main lacunas of the drug that restrict its overall therapeutic potency. Despite the high stability of genistein during storage, some literatures reported its possible degradation pathways. Genistein forms conjugates under acidic condition which followed by further degradation (Wang et al., Citation2002). Also, it forms conjugate with very high UV absorption in the presence of sugars (Ungar et al., Citation2003). Another possible degration pathway for genistein is its autodegradation at high temperature (60 °C) to form Maillard browning product (Davies et al., Citation1998).
A variety of nanosized-based drug delivery technologies—including nanocapsules (de Zampieri et al., Citation2013), lipid nanoparticles (Andrade et al., Citation2014), nanomicelles (Yin et al., Citation2015), nanoemulsions (Back et al., Citation2018), nanocrystals (Wang et al., Citation2018), metallic nanoparticles (Ghasemi Goorbandi et al., Citation2020), liposomes (Song et al., Citation2020) and gold nanoparticles (Vodnik et al., Citation2021)—have been engineered to overcome its inherent hindrances and widened the options for the treatment of several malignant diseases. Apart from their role as a nanocarrier, a novel class of flexible nanovesicles toward effectual transdermal drug delivery system such as transfersomes (Tfs) assists in their rapid penetration via the intercellular lipid pathway of the subcutaneous tissue. They consist of at least one inner aqueous compartment enclosed by a bilayer of concentric phospholipids in combination with edge activator (nonionic surfactant), which helps in solubilizing the stratum corneum (Rai et al., Citation2017; Srivastava et al., Citation2023). Additionally, it has acquired enormous demand in recent years due to well organized and depot release of low and high molecular weight drugs. Transferosomal drug delivery is the pioneer finding to encapsulate both hydrophilic and lipophilic drugs without any toxicological effect (Gayathri and Sangeetha, Citation2022). They impart numerous privileges such as evading variable GI absorption and GI incompatibility, avoiding first-pass metabolism, upgrading the bioavailability, better stability, decreasing the pace of administration and boosting patient conformity (Rai et al., Citation2017). They are metastable vesicles which build up the vesicle membrane to be extremely flexible and ultra-deformable that could be compressed to less than one-tenth of their own size without a measurable loss when applied under non-occlusive conditions (Maji et al., Citation2021). Despite these many advantages that transfersomes possessed, they have some challenges and/or limitations associated with their practical implementation. Transfersomes hydrophilic surface offered by edge activator limited its drug loading efficiency of lipophilic drugs compared to other phospholipid based nanovesicles. Also, the presence of nonionic surfactants as edge activator in transfersomes composition may result in perforation of their membrane that increases the liquification of the phospholipid bilayer (van den Bergh et al., Citation2001). Another challenge for transfersomes is the unequal hydration of different layers of stratum corneum. So, the inner parts of stratum corneum was reported to be more hydrated than the parts near the epidermis which ruined the concentration gradient that allowed continuous drug absorption. That may resulted in the development of depot effect (Jain et al., Citation2017). In addition to that, the large scale production of transfersomes is considered a very big challenge (Matharoo et al., Citation2024). Another main limitation of transfersomes is the dependency of its stability on the type of phospholipid used in their preparation. This is due to the liability of phospholipids to oxidative degradation upon exposure to air, sunlight or high temperstures (Parkash et al., Citation2018).
The present study was pointed at evolving a novel topical delivery system of genistein for treatment of skin melanoma using transfersomes as a nanocarrier. Transfersomes with various ratios of the drug, phospholipids and edge activator were prepared and characterized. The optimized transferosomal formulations—F5 and F6—were selected based on the particle size, PDI, zeta potential and encapsulation efficiency data then subjected to further characterization. Transfersome evaluations include: physicochemical characterization; transmission electron microscopy (TEM); in vitro drug release and stability studies. Furthermore, the antitumor activity of the optimized vesicular formulation was comprehensively evaluated using 3D ex vivo skin melanoma tumor spheroids through cell viability and Live/Dead cell assay. Inclusively, the developed transferosomal formulation is anticipated to reap benefits in improving the therapeutic efficacy against skin melanoma.
2. Materials and methods
2.1. Materials
Genistein (4′,5,7-trihydroxyisoflavone, C15H10O5, >95%) (Gen) was obtained as a free gift from geniVida™ TG, Germany. Span 60 (Sp60) and low molecular weight chitosan (50–190 kDa) (CS), D-α-Tocopherol polyethylene glycol 1000 succinate (TPGS) were purchased from Sigma-Aldrich, St. Louis, MO. Phospholipon 90 G (PL90G) was procured from Lipoid, Germany. Carbopol 940 (CP940) was obtained as a free gift sample from Lubrizol Advanced Materials, Inc. (Cleveland, OH). Dacarbazine, Dulbecco’s Modified Eagle Medium (high glucose) (DMEM) and 10% fetal bovine serum, were purchased from Fisher Scientific (Fair Lawn, NJ). WM164 skin melanoma cell line (RRID:CVCL number-7928) was obtained as a free gift from Dr. Lawrence Pfeffer lab, Department of Pathology, University of Tennessee Health Science Center, Memphis, TN. 3D tissue culture gel (Col-Tgel, medium stiffness) was purchased from 101Bio, Mountain View, CA. Cell-counting assay kit (CCK-8) was purchased from Dojindo Molecular Technologies, Japan. Cyto3D™ Live-Dead Assay Kit was purchased from The Well Bioscience Inc. North Brunswick Township, NJ. All other chemicals used in this study were analytical reagent grade.
The research in the current manuscript was conducted as a collaboration project between two labs; Dr. Amira Motawea lab (the first author) and Dr. Mohamed Ibrahim lab (the corresponding author) as follows: Studies done in Dr. Amira Motawea lab, Department of Pharmaceutics, Faculty of Pharmacy, Mansoura University, Mansoura, Egypt including; preparation of the original formulations, in vitro drug release, size characterization, pH, viscosity, XRD, DCS and FTIR. Studies done in Dr. Mohamed Ibrahim Lab, Department of Ophthalmology, University of Tennessee Health Science Center, Memphis, TN, USA including; preparation of the optimized formulations, size characterization, assessment of anti-tumor activity, cell viability and 1-year stability study.
2.2. Quantification of genistein
Genistein was quantified using a previously reported HPLC-UV method, with few changes. Briefly, HPLC system (KNAUER, Azura, Germany) attached to Supelco kromasil C18 column (5 μm, 100 Å, 4.0 mm × 300 mm) was used. A mixture of ammonium acetate (pH 3.37) and methanol (40:60) was used as mobile phase at a flow rate of 1.0 mL/min. The column was kept at 25 °C and the drug was detected after 6.5 min at a λmax 254 nm using photodiode array detector (Jandera et al., Citation2005; Kumar et al., Citation2016).
2.3. Preparation of genistein transfersomes
Genistein transfersomes (Gen Tfs) were fabricated by thin film hydration method with some modification (Sun et al., Citation2023). Preliminary studies were accomplished to optimize our formulation. Concisely, different drug quantity (6, 8 and 10 mg Gen) was weighed and dissolved in 5 mL absolute ethyl alcohol using an ultrasonic bath (Sonix IV, USA). TPGS as the edge activator (EA), phospholipon 90 G (PL90G) and Sp60 with different molar ratios were dissolved in 5 mL chloroform followed by sonication. Subsequently, both solutions were mixed in a rounded bottom flask. The solvents were evaporated at 60 °C under reduced pressure for 10 min at 120 rpm using a rotary evaporator (Rotavapor R-300, BUCHI, Germany) to form a uniform thin lipid film. The deposited film was hydrated with 10 mL deionized water containing glycerin (2.25%w/v) for 15 min at 60 °C at 120 rpm. The obtained lipid vesicles were left to harden for 2 h at 25 °C. The colloidal dispersion was sonicated using a probe sonicator (Sonics, Newtown, CT) with amplitude of 30% (10 sec on/off cycle) for 2 min in ice bath. Gen Tfs were kept at 4 °C until further analysis. The effect of PL90G:Sp60 molar ratio and drug concentration are the critical formulation parameters that were systematically optimized at different levels for the preparation of the optimized Gen Tfs. The blank formulation was prepared using the same method without the addition of the drug.
2.4. Determination of entrapment efficiency
The entrapment efficiency of Gen in the vesicular formulations was determined by the centrifugal ultrafiltration technique as previously reported (Mittal et al., Citation2019; Rassu et al., Citation2018). The vesicular formulations were placed in Amicon ultra-centrifugal filter of 10 kDa molecular weight cutoff and centrifuged (Acculab cooling centrifuge, USA) at 13,000 rpm for 1 h then analyzed for drug content by the HPLC-UV method mentioned before. The following equation was used to calculate the entrapment efficiency:
2.5. Particle size, polydispersity index (PDI) and zeta potential determination
Particle size and PDI of the selected vesicular formulations were analyzed by dynamic light scattering technique using a Zetasizer Pro (Malvern Instruments Ltd., UK). Samples were diluted 100-fold by Milli-Q water before the measurement. Three replicate size measurements were performed, and the average size value was calculated. For zeta potential (ZP) measurements, the same instrument was used utilizing laser Doppler Electrophoresis technique after 1000-fold dilution by Milli-Q water. Three series of five consecutive measurements were performed and an average ZP value was obtained. All determinations were performed in triplicate at 25 °C (Ferrado et al., Citation2023).
2.6. Transmission electron microscopy
The morphology of Gen and blank Tfs were examined using transmission electron microscopy (TEM) (JEOL JEM1200EX II electron microscope). Briefly, Tfs formulations were diluted 1:100 with Milli-Q water. On 400 mesh copper grids covered with Formvar film (Electron Microscopy Sciences EMS, Hatfield, PA), two microliters of the diluted Tfs were placed. The grids were allowed to dry for 2 h in a desiccator followed by negative staining with Uranyless EM stain (Electron Microscopy Sciences EMS, Hatfield, PA) before examination by TEM (Ibrahim et al., Citation2019).
2.7. Physicochemical characterization of Gen Tfs
Compatibility between Gen and various transfersomes components was determined by Fourier transform infrared (FTIR) in the transmission mode. FTIR spectra were recorded in KBr diffuse reflectance mode utilizing Nicolet iS10™ spectrometer (Thermo Fisher Scientific, Madison, Wisconsin). FTIR analysis for Gen, PL90G, Sp60, TPGS, the lyophilized blank and medicated F5 and F6 was performed by homogenously ground with KBr, manually compressed into disks and scanned over a frequency range of 4000 to 400 cm−1. A blank KBr disk was used to execute a background scan. Subsequently, FTIR software for data processing (OMNIC version 8) was used to record, visualize and interpret the corresponding peaks. The average of triplicate sample measurements of the IR distinctive peaks spectra was attained (Abd El Hady et al., Citation2023).
For DSC analysis, differential scanning calorimeter (Pyris 6 DSC, Perkin Elmer, USA) was used. Accurately weighed samples including Gen; PL90G; Sp60; TPGS; the lyophilized blank and medicated F5 and F6 were hermetically sealed in a crimped aluminum pan. The samples were scanned in the temperature range 30–400 °C with a heating rate of 10 °C/min. Our data analysis curves were automatically generated via Calisto treatment software. Before every run, the baseline was optimized (Abd El Hady et al., Citation2023).
X-ray diffraction (XRD) patterns of Gen, PL90G, Sp60, TPGS, lyophilized blank and their corresponding formulations (F5 and F6) were recorded utilizing X-ray diffractometer (Bruker, Germany) equipped with Cu-Kα x-ray radiation. The following conditions were employed to carry out the assessment, scanning range at 2-Theta 6–50° diffraction angle, the voltage at 45 kV current at 40 mA (Ibrahim et al., Citation2019).
2.8. Preparation of genistein-loaded vesicular formulation hydrogels
The optimized Gen Tfs formulations (F5 and F6) were incorporated into preprepared hydrogel for topical application. The prepared hydrogels were 2% low molecular weight chitosan (CS) or 0.5% Carbopol 940 (CP940). CS hydrogel was prepared in 1% glacial acetic acid followed by pH adjustment to 5.5, while CP940 was prepared in Milli-Q water. The concentration of chitosan and Carbopol 940 polymers was selected based on a pre-formulation study. The hydrogels were then homogenously mixed with the selected Gen Tfs formulations in a 1:1 ratio to produce Gen Tf gels. For CP940 Gen Tf hydrogel, triethanolamine was added dropwise to achieve the desired viscosity and pH of the formulation. The prepared Gen Tf hydrogels were packed in air-tight containers and stored at 4 °C for further characterization.
2.9. Homogeneity, viscosity and pH evaluations
The homogeneity was observed visually for any clumps or aggregation in the prepared hydrogels. Additionally, all hydrogels were hard-pressed between the index and the thumb fingers to interpret reliability as homogeneous or non-homogeneous (Abdellatif et al., Citation2018; Insaf et al., Citation2023). The viscosity of our formulations was assessed on Discovery Hybrid Rheometer DHR-3 (Waters TA Instruments, New Castle, DE) at 25 °C using the flow rheological technique. The viscosity was determined using cone and plate attachment at shear rate 10 s−1 (n = 3) (Maji et al., Citation2021).
For determination of pH, 1 gm of the prepared Gen- and Gen Tf hydrogels was diluted to 20 gm by Milli-Q water and the pH was measured at 25 °C by pH meter (Beckman Fullerton, Germany). All measurements were performed in triplicate to confirm the accuracy and consistency and the results were expressed as mean ± SD.
2.10. Determination of the percentage drug content
To ensure the homogeneity of drug our Tfs, distribution in the hydrogels, the drug content of the hydrogels was analyzed by accurately weighing 0.1 g of each hydrogel into a 10 mL volumetric flask. The gel formulations were dissolved in 1 mL DMSO and the mixtures were vortexed using vortex mixer (Fisher Scientific, Fair Lawn, NJ), then the volume was completed to 10 mL by Milli-Q water. After filtering the solution using a 0.22 µm Millipore filter, samples were assayed for the drug concentration using HPLC-UV method that was mentioned above.
2.11. In vitro drug release and kinetics studies
A dialysis bag of molecular weight cutoff 10,000–12,000 Da was adopted to perform the in vitro drug release of the prepared vesicular formulations. The hydrogels of the optimized transferosomal formulation F5 and F6, the optimized vesicular formulation dispersions (F5 and F6) and Gen aqueous suspension (control) were evaluated in this study. One hundred milliliters of phosphate buffer saline pH 7.4 (PBS) containing 1% polysorbate 20, was used as a release medium at a temperature of 37° ± 0.5 °C and stirred at 50 rpm. A sample aliquot (2 mL) was withdrawn at predetermined time points and immediately replaced with fresh two milliliters PBS for 48 h (Raval et al., Citation2018). For getting insights into the mechanism of in vitro release kinetics, release data were plotted according to different release kinetics models including zero order; first order; Higuchi (Higuchi, Citation1963); Korsmeyer–Peppas (Korsmeyer et al., Citation1983) and Weibull. Each experiment was performed in triplicate and the results were calculated as mean ± SD.
2.12. 3D Cell culture ex vivo skin melanoma simulation for evaluation of the optimized Gen Tfs formulations antitumor activity
2.12.1. Formation of 3D tumor spheroids
To form the 3D skin melanoma tumor spheroids, WM164 skin melanoma cell line was first allowed to grow in traditional 2D culture design in high glucose DMEM medium containing 10% fetal bovine serum and 1% penicillin/streptomycin and incubated at humidified atmosphere of 5% CO2 at 37 °C. The cells were allowed to grow in these 2D conditions to produce the required number of cells that needed to form the 3D tumor spheroids. After reaching the required cell count, WM164 cells were then dispersed in the gelatin solution (component A of the Col-Tgel) at a concentration of 2 × 106 cell/mL of gelatin solution. Transglutaminase crosslinker (component B of the Col-Tgel) was mixed with the cell dispersion by repeated gentle pipetting. In a 48-well plate, 20 µL of this mixture was dropped in the middle of the well. The plate was incubated for 45 min at humidified atmosphere of 5% CO2 at 37 °C without the addition of any culturing medium to allow the Col-Tgel to reach the required consistency. After the gel hardening and the formation of the 3D tumor spheroids, 0.5 mL of high glucose DMEM medium was added. This medium was added to cover the gel and prevent its dryness. The 3D tumor spheroids were allowed to grow for three days with daily medium changing before drug treatment (Fang et al., Citation2016; 101Bio, Citation2024).
2.12.2. Evaluation of Gen Tfs antitumor activity
After three days of incubation is required for the 3D tumor spheroids growth (per the manufacturer’s protocol), treatment was started for five days (the recommended treatment period for our positive control). Different formulations were evaluated including Gen solution; Gen Tfs; blank Tfs; Dacarbazine solution (positive control)—a well-known chemotherapeutic drug that is recommended for melanoma skin cancer—as well as untreated tumor spheroids (negative control). All formulations were sterilized and used at a concentration of 0.8 mg/mL. All formulations were diluted with the culture medium and replaced two times with freshly diluted formulations during the experiment to ensure that cells got the required nutrition during the whole evaluation period. After five days of incubation with different treatments, the antitumor activity was evaluated using both cell viability and live/dead cell assays.
2.12.2.1. Cell viability test
After 5 days of incubation with different treatments, cell-counting assay kit-8 (CCK-8) that contains WST-8 [2-(2-methoxy-4-nitrophenyl)-3-(4-nitrophenyl)-5-(2,4-disulfophenyl)-2H-tetrazolium, monosodium salt], was used to quantify the cell viability in the tumor spheroids due to the ability of the live cells to reduce the WST-8 tetrazolium salt to a yellow colored water soluble formazan dye. The intensity of the yellow color is directly proportional to the number of living cells. According to the manufacturer’s protocol, medium in each well was replaced by 500 µL of fresh medium containing 50 µL of stock CCK-8 reagent (Kumari et al., Citation2022). The plate was incubated for 4 h in humidified atmosphere of 5% CO2 at 37 °C. After 4 h, 100 µL of medium was transferred into a 96-well plate and the absorbance was measured at 450 nm by a µ-Quant universal microplate spectrophotometer (Bio-Tek Instruments, Inc. Winooski, VT). Medium containing the CCK-8 reagent was served as baseline control. Cell viability was calculated as a percentage compared to the negative control (untreated cells). According to the protocol, cell viability evaluation using CCK-8 assay kit depends mainly on the ability of the live cells on the surface of the tumor spheroids to reduce the tetrazolium salt to its orange formazan derivative followed by measuring the orange color intensity in the growth medium outside the tumor spheroids. Therefore, we can deduce that CCK-8 assay kit is specifically designed to evaluate the number of viable cells on the surface of the tumor spheroids (i.e. superficial evaluation) (Fang et al., Citation2014; Laboratories, Citation2023).
2.12.2.2. Live/dead cell assay
After 5 days of application for all treatments, a live/dead cell assay was performed using Cyto3D™ Live-Dead Assay Kit. The kit contains acridine orange (cell permeable) and propidium iodide (cell non permeable) dyes. Briefly, 2 µL of Cyto3D reagent was added to each 100 µL of the total liquid volume in the well (hydrogel and medium). The plate was incubated in humidified atmosphere of 5% CO2 at 37 °C for 30 min. The medium containing the Cyto3D reagent was removed, and tumor spheroids were washed three times with sterile PBS (Cyto3D™ Live-Dead assay kit pamphlet, Citation2023). Tumor spheroids were then subjected to imaging using confocal microscope (Zeiss LSM 980, Germany). In contrast to the cell viability assay, confocal microscopy in addition to its superficial evaluation, it demonstrates the cell viability in deeper layers inside the tumor spheroids. The dead cells images obtained from confocal microscope were quantified using ImageJ software and the percentage cell death was calculated for each tested formulation compared to the positive control (i.e. Dacarbazine solution). Results were statistically analyzed by one way ANOVA followed by Tukey’s multiple comparisons test using GraphPad Prime 10 software and expressed as (mean ± SD) (GraphPad Software Inc., San Diego, CA).
2.13. Stability studies of the optimized Gen Tf formulations
Stability studies of our optimized Gen Tf formulation (F6) were tested by storage at two different temperatures (5° ±1 °C and 25° ±1 °C) for 12 months. Four formulations were evaluated including F6 Gen Tf dispersion; F6 Gen Tf CP940 hydrogel; blank F6 Tfs dispersion and blank F6 Tfs CP940 hydrogel. Three independently prepared batches of each formulation were evaluated. All Tf formulations were packed in air-tight glass vials and protected from light by wrapping with aluminum foil. Tf dispersion formulations were used to test aggregation, physical appearance, particle size, PDI and zeta potential changes. Tf hydrogel formulations were used to evaluate the changes in the pH, consistency and drug contents at various time points of storage. Our Tf formulations were evaluated initially and at specified time intervals—1, 2, 3, 6, 9 and 12 months. All experiments were performed in triplicate and the results were expressed as mean ± SD. Results were statistically analyzed by one way ANOVA followed by Tukey’s multiple comparisons test using GraphPad Prime 10 software (GraphPad Software Inc., San Diego, CA).
3. Results and discussion
Lipid-based nanocarriers and new generations of phospholipid vesicles (e.g. transfersomes) have a great influence on the following characteristics: better encapsulation of lipophilic drugs; improved safety; penetration through biological barriers; site specific targeting to tumor and hence their therapeutic performance. In the present study, the choice of lipid-based nanovesicles—transfersomes—with their penetration enhancement and structure flexibility due to the edge activators, is very promising as a topical drug delivery system (Abd-Allah et al., Citation2023). Advantageously, genistein-loaded transfersomes could permeate across the stratum corneum and drive their payload more efficiently into the deeper layers of the skin, augmenting their therapeutic efficacy owing to their ultra-deformable flexible structure (Maji et al., Citation2021). Transfersomes lipid-based nanocarriers were selected as a dosage form for genistein topical delivery due to the many advantages they possessed. Among these advantages; their flexibility and ultradeformable structure that allow them to pass through smaller pore size (1/10 of its original size) that resulted in better skin penetration enhancement (Jiang et al., Citation2018; Demartis et al., Citation2021). They could be used to incorporate a variety of drug molecules that include; hydrophilic or lipophilic drugs, small or large molecules. They are very safe as they usually prepared from biocompatible materials (Gayathri and Sangeetha, Citation2022). TPGS was selected as an edge activator in our Tfs due to its biocompatibility, its hydrophilic characteristics, its antioxidant properties that could increase the stability of the Tfs and its FDA approval (Alhakamy et al., Citation2019). Another reason for the use of TPGS in our Tfs is its P-glycoprotein inhibition ability which help in decrease drug resistance following transdermal delivery (Skazik et al., Citation2011). The developed modified transfersomes were evaluated according to their PS, PDI and ZP values, which will affect their pharmacokinetics behavior such as absorption and distribution and also their toxicity and targeting capabilities (Akl et al., Citation2023).
3.1. Entrapment efficiency, particle size, PDI and zeta potential of Gen Tfs
To optimize our transferosomal formulations, the effect of the PL90G:EA (TPGS) molar ratio and Gen concentrations were investigated. Preliminary studies were performed to attain the optimal formulation by testing different molar ratios of the drug, Sp60, PL90G, and different organic solvent ratios. The effect of these factors on the PS, PDI, ZP and % EE were evaluated. The criteria of selecting our optimal Tf formulation include: the smallest PS and PDI values and the highest ZP and % EE values. represents the PS and ZP values of our optimized F6 Tfs formulation. As revealed in , all particle sizes of the prepared transfersomes are in the range of 150.40 − 266.90 nm with a small PDI value (0.19–0.33) which is indicative of a narrow particle size distribution pattern. PDI values (˂0.5) depict better distribution and are referred to as unimodal NP dispersion which is imperative for warranting the stability of a colloidal dosage form upon storage (Danaei et al., Citation2018). Here, the average ZP values of all the prepared Tf formulations are in the range of −24.93 ± 0.15 to −48.41 ± 1.25 mV. It is worth remembering that ZP values above 30 mV (either negative or positive) are requisite for the provision of very good stability in the dispersion medium and colloidal systems because the nanoparticles had enough repulsion and became less prone to form aggregates (Kamble et al., Citation2022; Maji et al., Citation2021). In addition to the stability, the high negative charge carried by our Tfs offers more biocompatibility and less biological toxicity as previously reported that negatively charged particles are less toxic than positive particles due to their lower ability to induce the production of reactive oxygen species (Lockman et al., Citation2004; Ortiz et al., Citation2019). Negative ZP of our Tfs was conferred to the use of negatively charged lipid molecules such as PL90G which carry a negatively charged phosphate groups (Allam et al., Citation2022). In addition to the colloidal system stability conferred by electric repulsion between particles, this stability was improved by the steric stability accorded by the steric shielding influence of the PEG moiety of TPGS (Luiz et al., Citation2021; Yang et al., Citation2018).
Figure 1. (A,B) The average particle size and zeta potential of the optimized Tf formulation (F6); (C,D) TEM images of blank and medicated Tf formulations that reveal its spherical outlines and uniform size distribution.
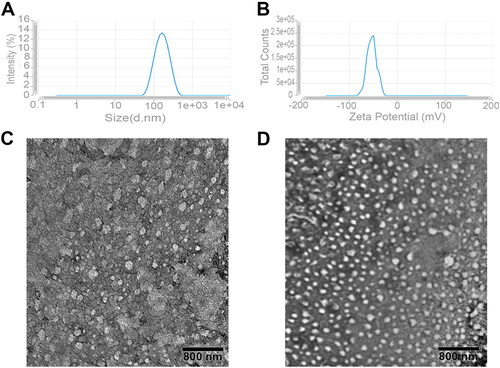
Table 1. Characterization of genistein transfersome formulations.
As presented in , the % EE of the prepared Tf formulations vary from 85.73 ± 0.26% to 96.65 ± 0.27%. The drug entrapment efficiency increases with the increase of Gen amount from 6 to 10 mg which indicates that maximum drug encapsulation is achieved at Gen amount of 10 mg, after which excess drug is precipitated, leading to reduced %EE (data not shown). Highly lipophilic drugs have the propensity to be completely packed within the well-packed tight bilayer structure formed by Spans due to their lipophilic nature and the low hydrophilic-lipophilic balance value (HLB) of Spans (Ramkanth et al., Citation2021). Our results demonstrate that the particle size of F5 and F6 is below 170 nm with low PDI and high ZP values compared to the other formulations, manifesting the feasibility of these systems for considerable localized Gen delivery through the skin (). Furthermore, TEM analyses () of blank and medicated Tfs (F6) reveal the spherical outlines of Tfs with uniform size distribution and lack of aggregations that confirm the particle size results obtained from the zeta sizer.
3.2. Physicochemical characterization of Gen Tfs
Physicochemical evaluations including FTIR; DSC and XRD were performed on the lyophilized optimized blank and medicated transfersome formulations (F5 and F6). All these evaluations will assess the possible interaction of Gen with other transfersome excipients and evaluate the crystallinity status of the entrapped Gen inside the Tfs to confirm their suitability for better topical application.
3.2.1. FTIR study
FTIR spectroscopy is a widely nondestructive technique used for studying the possible interaction of distinctive functional groups between drugs and excipients by perceiving the changes in the characteristic wavenumber of each molecule (Malviya et al., Citation2023). As elucidated in (i), Gen shows its principal Infrared peaks at 3412 cm−1 (–O–H stretching), 3105 cm−1 (–C–H stretching), 1653 cm−1 (–C=O stretching), 1612–1424 cm−1 (C–C stretching), 1308–1253 cm−1 (–O–H bending), 1198–1043 cm−1 (C–O–C stretching) and 884–817 cm−1 (–C–H bending). (ii), illustrates the spectrum of PL90G which reveals characteristic stretching bands (C–H) at 2926 and 2855 cm−1, a stretching band of the ester carbonyl group (C=O) at 1739 cm−1 and an ester stretching band (C–O) at 1241 cm−1 (Salama et al., Citation2019). As displayed in (iii), Sp60 presents broad band at 3420 cm−1, sharp broad band at 2919 cm−1, and a characteristic peak at 1739 cm−1 (Sabry et al., Citation2021). As shown in (iv), the absorption terminal –O–H group, –CH stretching, the characteristic C=O and C–O stretching bands of TPGS appear at 3448, 2891, 1742, and 1113 cm−1, respectively (Sharma and Chauhan, Citation2020). As is evident from , the characteristic peaks of Gen are slightly shifted after drug encapsulation in Gen Tfs. Also, the FTIR spectra of both medicated and blank transfersomes () retain the characteristic peaks of all the excipients without any major shifts which pursue the stability of our prepared transfersomes and the absence of any chemical interaction between its components (Hady et al., Citation2022).
Figure 2. (A) Fourier transform infrared spectra and (B) Differential scanning calorimetry of (i) gen, (ii) PL90G, (iii) Sp60, (iv) TPGS, (v) blank F5, (vi) medicated F5, (vii) blank F6 and (viii) medicated F6. The FTIR spectra demonstrate that the characteristic peaks of gen are slightly shifted after drug encapsulation in Tfs. Both medicated and blank Tfs also retain the characteristic peaks of all the excipients without any major shifts which is indicative of the absence of any chemical interaction between different components. The DSC demonstrates the reduced lipid crystallinity after incorporation of gen in the lipid bilayer of Tfs. In addition, the similarity of blank and medicated Tfs confirm the physical involvement of gen in the Tf structure, molecular dispersion and solubilization.
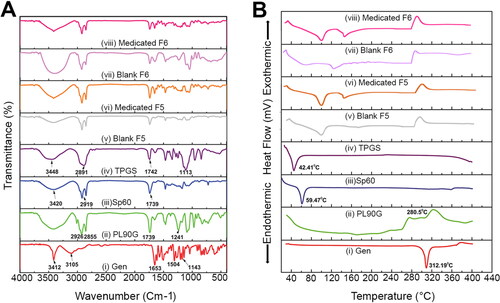
3.2.2. DSC study
DSC thermograms were recorded for Gen, PL90G, Sp60, TPGS, blank and medicated selected Tfs (F5 and F6) () to provide data about the state of drug dispersion in the nanosystems. Furthermore, DSC helps to determine if there is a chemical interaction between the drug and transfersome components (Luiz et al., Citation2021). The thermogram of Gen demonstrates a single, well defined endothermic peak at 312.19 °C with an onset at 283.14 °C, pinpointing the melting point of this crystalline substance ((i)) (Jangid et al., Citation2022; Komeil et al., Citation2022). The thermogram of PL90G ((ii)) presents three broad peaks at 170.3, 220.3 and 280.5 °C, consistent with the thermal behavior of amorphous substances (Salama et al., Citation2019). The thermogram of Sp60 demonstrates an endothermic peak at 59.47 °C while TPGS shows an endothermic peak at 42.41 °C (), respectively which agrees previously reported results (Elsaied et al., Citation2016; Lee et al., Citation2015). Compared to the bulk lipid, in transfersomes, either Gen-free or Gen-loaded, the melting peak corresponding to PL90G is shifted toward reduced temperatures at 272 °C (). This thermal behavior demonstrates the reduced lipid crystallinity boosting the incorporation of Gen in the lipid bilayer of the formed vesicles and ascertain the interlinkage between transfersome components and hence their cohesion within the lipid bilayer. Furthermore, in medicated Tfs—F5 and F6—the characteristic peak of SP60 and TPGS are shifted to higher temperature with reduced intensity (). These results coincide with previously reported data of other authors (Akl et al., Citation2023; Tsakiri et al., Citation2024). Moreover, the lack of any peak close to Gen melting point in Gen loaded Tfs that had a similar spectrum with the unloaded transfersomes suggests its physical involvement in transfersomes structure, molecular dispersion and solubilization (Jangid et al., Citation2022).
3.2.3. XRD study
The crystallinity of Gen was confirmed by the XRD pattern analysis. The pure Gen () displays sharp peaks at 7.45°, 12.68°, 15.94°, 17.99°, 26.29°, 27.35°, 28.64°, 29.36°, 33.54°, 35.91° and 40.56° which confirms its crystalline structure. A single diffraction peak was observed at 2θ = 7.55° and 21.56° for PL90G and Sp60, respectively (). In addition, TPGS reveals two characteristic crystalline diffraction peaks at 19.28° and 23.53° () (Lee et al., Citation2015). As is evident from , the pure Gen specific peaks are not perceived in our prepared transfersomes which confirms the amorphousness of the drug in the Tf formulation. The XRD results confirm the obtained results of encapsulation efficiency and DSC, indicating that transfersomes encapsulated a high amount of Gen that entrapped in a non-crystalline state inside the transfersomes.
Figure 3. (A) X-Ray diffraction of (i) gen, (ii) PL90G, (iii) Sp60, (iv) TPGS, (v) blank F5, (vi) medicated F5, (vii) blank F6 and (viii) medicated F6. The XRD spectra illustrate that gen is entrapped within tfs in a non-crystalline or amorphous state. (B) In vitro drug release profiles of different gen formulations illustrate the sustained release behavior of our tfs (both dispersion and hydrogel) compared to the free drug.
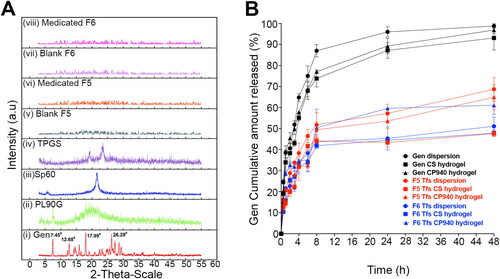
3.3. Homogeneity, viscosity, pH and % drug content evaluations of optimized gen Tf hydrogels
All the prepared hydrogels possess good homogeneity with no observed clumps or aggregations. The viscosity of our prepared Tf CS hydrogels of medicated F5 and F6 is 27.90 ± 1.21 and 28.64 ± 1.33 Pa.s, respectively. In addition, the viscosity of our Tf CP940 hydrogels is 31.14 ± 2.03 and 33.23 ± 0.89 Pa.s for medicated F5 and F6, respectively. These data reveal that all our prepared hydrogels demonstrate an optimum viscosity with suitable texture and consistency required for an excellent skin retention (Ramkanth et al., Citation2021). The pH of the topical gel should ideally be close to the skin pH to not cause any skin irritation. The medicated F5 and F6-based CS hydrogels have pH values of 6.6 ± 0.2 and 6.7 ± 0.3, respectively, while, that of CP940 hydrogels have pH values of 5.76 ± 0.54 and 5.58 ± 0.91 for medicated F5 and F6 hydrogels, respectively. According to the European Group of efficacy measurement and evaluation of cosmetics (EEMCO) guidance, the ideal pH for topical skin formulations should be in the range of 4.5 − 7.0 to be tolerable by the skin without any irritation (Parra et al., Citation2003). In addition, the percentage drug content of our prepared Tf hydrogels—F5 and F6—is 95.4 ± 1.4% and 97.9 ± 2.3%, respectively. These data are indicative of the homogeneous Gen distribution in the prepared hydrogels which confirms its suitability for topical administration.
3.4. In vitro drug release and kinetics studies of optimized Gen Tf formulations
The release profiles of Gen from its aqueous dispersion (control) and the selected optimized Tfs formulations (F5 and F6) were evaluated and the results are presented in . A rapid diffusion rate is observed for Gen dispersion, with more than 50% drug released within the first 3 h and ∼−85% after 8 h. In sharp contrast, a much slower release profile of our optimized Tf formulations—F5 and F6—is observed during the whole in vitro release process. Biphasic release patterns are observed for Gen Tfs with an early burst release of ∼30% of the drug load at 3 h followed by a sustained release for more than 48 h. The early burst release is mainly assigned to the drug adsorbed on the surface and poorly entrapped inside the Tfs core, which is easily diffused into the release medium (Manickavasagam et al., Citation2018). Another reason for this rapid onset release is the small particle size of the Tfs that resulted in larger surface to volume ratio and higher release rate for drug located near to Tfs surface (Danaei et al., Citation2018). However, the following sustained release phase is principally ascribed to the erosion of the transfersomes and then the diffusion of the well-encapsulated Gen (Xiao et al., Citation2020). The solid matrix of the Tfs is also responsible for drug immobilization which supports the slow release rate of Gen. Impressively, the biphasic drug release pattern is expected to enhance the therapeutic proficiency of Gen. Because the poorly entrapped drug on the Tf surface will be ready to be absorbed and then will produce immediate response upon application. However, the well-encapsulated Gen portion will preserve the continuous drug response for an extended time period (i.e. rapid onset and long duration of action) (Malviya et al., Citation2023). As illustrated in , the drug release profiles of both the free Gen and our prepared Tf dispersion were best fitted with the Weibull model, which has the highest correlation coefficient values of 0.958, 0.936 and 0.868 for the free Gen, F5 and F6 dispersions, respectively. In Weibull model’s, β value is used to describe the drug release mechanism (Maji et al., Citation2021). Fickian diffusion is anticipated if the β value is ≤ 0.75 while 0.75 < β < 1 is indicative of a combined Fickian diffusion and Case II transport mechanism. Nonetheless, for β > 1, the drug release follows a complex release mechanism. Our data show β values of 0.624, 0.421 and 0.337 for the free Gen, F5 and F6 dispersions, respectively which demonstrates that Gen release follows a Fickian diffusion mechanism (Corsaro et al., Citation2021).
Table 2. Drug release kinetics of genistein in different formulations.
Drug release profiles of the selected transferosomal formulations F5 and F6 in either CS or CP940 hydrogels compared to the corresponding Gen gel are presented in . For chitosan gels, as is evident from Gen-loaded CS gel shows faster release rate with 93.10 ± 3.78% drug release after 48 h compared to F5 and F6 Tf CS hydrogels, which show 47.6 ± 0.9% and 47.45 ± 1.38% drug release, respectively after 48 h. Similar results are observed with CP940-based hydrogels. Gen-loaded CP940 gel illustrates rapid Gen release of 96.9 ± 3.66% after 48 h compared to F5 and F6 Tf CP940 hydrogels which present 64.9 ± 3.59% and 61.1 ± 0.66% drug release, respectively after 48 h (). These results demonstrate that the hydrogel-based transferosomal formulations exhibit highly controlled drug release rate compared to the formulations that lack Tfs. This observation is likely attributed to two main reasons: the first is the solid matrix of the transferosomal gel which is responsible for drug immobilization and subsequently slower drug release than the Gen hydrogel (Maji et al., Citation2021). The second reason for that sustained drug release of Gen Tf hydrogels, is the extra step included in the drug release mechanism. To be released, Gen has to first diffuse from the Tfs to get out of its vesicular structure then it has to diffuse through the viscous gel matrices to reach the release membrane. This extra step greatly extends its release from Tf hydrogels.
The release kinetics of Gen from different formulations were evaluated in vitro to determine the efficacy of the transfersomes as a prospective topical drug delivery system. The drug release data obtained from the optimized Tf formulations was evaluated using various release kinetics models such as: zero order; first order; Higuchi; Weibull and Korsmeyer-Peppas and the results were listed in . In case of chitosan hydrogels, relying on the determination coefficients values, Weibull model is determined to be the best fit for the release data. The calculated β values are ≤ 0.75 (0.624, 0.421 and 0.337 for the Gen-loaded CS hydrogel, Gen-loaded F5 and F6 Tf CS hydrogels, respectively), demonstrating a Fickian diffusion-controlled drug release mechanism (Sharma et al., Citation2022). Similar results are observed for CP940 formulations where the best fit model is Weibull model except for F6 Tf CP940 hydrogel which determined to follow the Korsmeyer-Peppas model with n value of 0.214 that presents a Fickian (≤0.5, non-steady) diffusion-controlled release mechanism (Hady et al., Citation2022).
3.5. 3D Cell culture ex vivo simulation of skin melanoma for evaluation of the optimized Gen Tf formulations antitumor activity
WM164 skin melanoma cells were allowed to grow in 3D spheroids designed to mimic the complex skin tumor microenvironment using medium stiffness Col-Tgel. Previous studies demonstrated that Col-Tgel is appropriate for in vitro tumor spheroids bioengineering because it resembles the complexity of the tumor matrix. The degree of stiffness of the Col-Tgel depends mainly on the type of the studied tumor (Fang et al., Citation2016). In the current study, medium stiffness Col-Tgel was selected to match the stiffness of skin melanoma (101Bio, Citation2024). After seeding the Col-Tgel with WM164 cells, the spheroids were allowed to grow for 3 days before any treatment because of the expected prolonged doubling time and the slower metabolic rate within the tumor spheroids microenvironment (Fang et al., Citation2016).
After the formation of well-developed WM164 tumor spheroids, Gen antitumor activity was evaluated using two different techniques (cell viability assay and live/dead cell assay). Cell viability assay is used to evaluate the cell viability on the tumor surface because reagent color change is only measured in the medium surrounding the tumor spheroids. However, live/dead cell assay is used mainly to evaluate the antitumor activity inside the tumor mass and determine the ability of the formulation to penetrate into deeper area inside the solid tumor.
3.5.1. Cell viability
describes the percentage cell viability quantification results of the tested formulations using CCK-8 assay kit. The results show that the blank F6 Tfs has a comparable percentage cell viability result (100.79 ± 4.90%) to that of the untreated tumor spheroids (medium only, 100% cell viability) with no significant statistically difference (p = .9997). These results confirm the safety and biocompatibility of our transfersome ingredients. On the other hand, comparing the percentage cell viability of Gen F6 Tfs (13.33 ± 1.044%), Dacarbazine solution (5.79 ± 0.025%) and Gen solution (5.65 ± 0.115%) with the untreated tumor spheroids (100 ± 4.71%), our results show very high significant differences (p < .0001), which demonstrate the antitumor activities of these formulations. Our results also illustrate that there is no statistically significant difference in the percentage cell viability between Dacarbazine and Gen solutions (p = .9999). These data demonstrate that Gen has a comparable antitumor activity to that of the positive control. In contrast, there are significantly lower percentage cell viability was detected upon comparing Gen Tfs with Dacarbazine solution (p = .0018) and Gen solution (p = .0011). The lower efficacy of Gen Tfs compared to Dacarbazine and Gen solutions is expected to be mainly due to two factors: the first is the direct effect of the drug available in solution on the cells located superficially on the surface of the tumor spheroids which is higher in case of drug solutions than in case of transfersomes. The second factor is the encapsulation of Gen inside the lipid structure of the transfersomes that requires longer time to be released and exert its antitumor effect. Depending on these two factors, we can deduce that, because the cell viability study is designed to evaluate the number of viable cells on the surface of the tumor spheroids, we expect that the drug solutions will have higher antitumor activity than transfersomes because of the availability of high amount of free drug molecules in the solutions.
Figure 4. (A) The percentage cell viability assay using CCK-8 assay kit, which illustrates that gen has a comparable antitumor effect with the dacarbazine (the positive control). (B) Confocal microscope images of the edges of the 3D spheroids of WM164 skin melanoma cells with live/dead assay showing dead cells (red), total cells (green) and the merged images. (C) The quantitative estimation of the dead cells images using ImageJ software that illustrates the superiority of the antitumor activity of gen tfs due to their ability to penetrate into deeper layers inside the tumor spheroids.
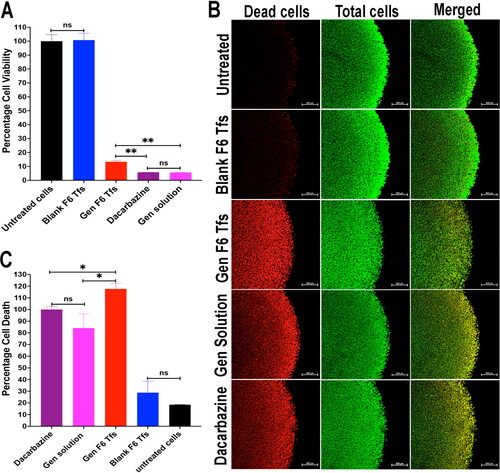
3.5.2. Live/dead cell assay
illustrates the confocal microscope images for the tumor spheroids treated with different formulations with the dead cell column (stained red) and the total cell column (stained green). demonstrates the quantitative evaluation of the percentage cell death (antitumor effect) of different formulations. The results show that the blank F6 Tfs has a comparable percentage cell death count to that of the untreated tumor spheroids with no significant statistical difference (p = .849). These results confirm the safety and biocompatibility of our transfersome ingredients and agreed with the percentage cell viability results. On the other hand, comparing the percentage cell death of Gen F6 Tfs (117.6 ± 4.78%), Dacarbazine solution (100 ± 2.46%) and Gen solution (84.1 ± 12.12%) with the untreated tumor spheroids show high significant differences (p < .001), which demonstrate the antitumor activities of these formulations. Also, the results show that there is no statistically significant difference in the cell death percentage between Dacarbazine and Gen solutions which confirms that Gen has a comparable antitumor activity compared to the positive control (p = .589). In contrast to the cell viability test results, the results of the cell death present a significantly higher antitumor effect of Gen Tfs compared to both Dacarbazine and Gen solutions (p < .05). This higher efficacy of Gen Tfs compared to the drug solution is likely due to the synergetic effect of the Tfs components and the drug. Among these components is the edge activator (TPGS) which provides Tfs with its hydrophilic, ultra-deformable and flexible structure that provides it with high penetration ability through deeper area inside the tumor spheroid core. Other Tfs components like the phospholipid (Hmingthansanga et al., Citation2022) and span 60 (Mady et al., Citation2019) are known to be permeability enhancers which also could provide a synergetic effect. The higher antitumor efficacy of Gen Tfs may be also attributed to the small particle size of the Tfs that enables for higher penetration through the tumor and longer retension inside it, which resulted in better efficacy of the chemotherapeutic agent (Maeda, Citation2015; Caracciolo, Citation2018). Another reason for the higher efficacy of Gen Tfs, is its negative zeta potential value that enables better penetration and entrapment into the tumor tissues (Miatmoko et al., Citation2022). Several previous researches proved the penetration enhancing ability of transfersomes through skin melanoma tissue due to its flexible and ultra-deformable structure which enable them to compress themselves to less than one-tenth of their own size to easily pass though the tissues (Jiang et al., Citation2018; Demartis et al., Citation2021). The TPGS incorporated in our transfersomes acts as an edge activator that provided Tfs with these flexible and ultra-deformable behavior that allows them to easily penetrate into deeper area in the tumor spheriods. In conclusion, our data demonstrate that Gen Tfs is a promising drug delivery system for the treatment of skin melanoma (Maji et al., Citation2021).
3.6. Stability studies of the optimized Gen Tf formulations
Regarding the physical stability of Tfs dispersion after storage for 12 months at different temperatures, there were no observable signs of physical changes in both medicated and blank Tfs dispersions such as precipitation or aggregation. The improved physical stability of transfersomes drug delivery system may be due to the the increased elasticity and surface hydrophilicity as a result of the presence of edge activator. This enhanced elasticity and surface hydrophilicity prevent agglomeration and coalescence due to osmotic stress. This unique characteristic of transfersomes make them more advantageous than the traditional liposomes (Cevc, Citation2004; Matharoo et al., Citation2024). The particle size data of both medicated and blank Tfs are presented in and , respectively. The results demonstrate that our Tf formulations possess an excellent particle size stability with no significant changes in the particle size of the medicated Tfs after 1 year of storage at different temperatures (p > .05). The average particle size of the fresh Gen Tfs was 168.867 ± 9.774 nm and after 12 months, the particle size was 164.278 ± 4.110 nm and 199.811 ± 14.539 nm for medicated formulations at 5° and 25 °C, respectively (). Regarding the blank Tfs, the average particle size of the fresh sample was 160.392 ± 2.710 nm with no significant changes after 1 year of storage at 5 °C (p > .05). In addition, at 25 °C there were no significant changes in the particle size of the blank Tfs during the 1st 6 months (p > .05). However, after 6 months of storage there was a significant increase in the blank Tfs particle size (p = .0090), which is likely due to particle aggregation as a result of long standing at higher temperature (Omar et al., Citation2019) as illustrated in . Despite the significant increase in the particle size of the blank Tfs after 6 months of storage at 25 °C, the particle size is still in the acceptable range for efficient delivery of the encapsulated drug into deeper layers of the skin (Verma et al., Citation2003). Also, it is reported that a particle size below 600 nm is essential to deliver the encapsulated drug into different layers of skin tumor (Danaei et al., Citation2018). Regarding the PDI values of Tfs demonstrated in and , although there were significant changes especially for the medicated Tfs at some time points, all the measured PDI values are still in the acceptable range for phospholipid-based vesicles (< 0.4) (Putri et al., Citation2017). It is known that PDI is an indicator of the quality of particle size distribution. It is clearly observed from our results ( and ) that our Tfs has a uniform and narrow particle size distribution which is indicative of a good physical stability and perfect performance at the tumor application site (Danaei et al., Citation2018).
Figure 5. Stability evaluations of medicated Tfs after storage for 12 months at 5° and 25 °C. (A) Particle size, (B) Polydispersity index, (C) Zeta potential, (D) pH and (E) Drug content. The stability results demonstrate the high physical and chemical stability of our Gen Tfs.
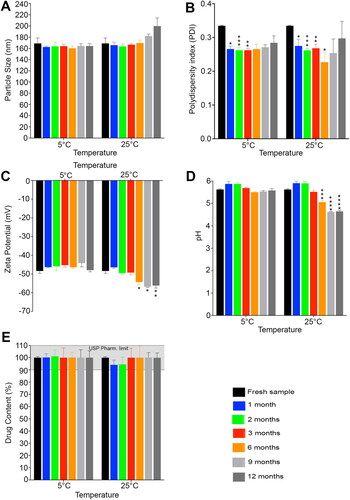
Figure 6. Stability evaluation of the blank tfs after storage for 12 months at 5° and 25 °C. (A) Particle size, (B) Polydispersity index, (C) Zeta potential and (D) pH. The stability results demonstrate the high physical stability of the blank tfs.
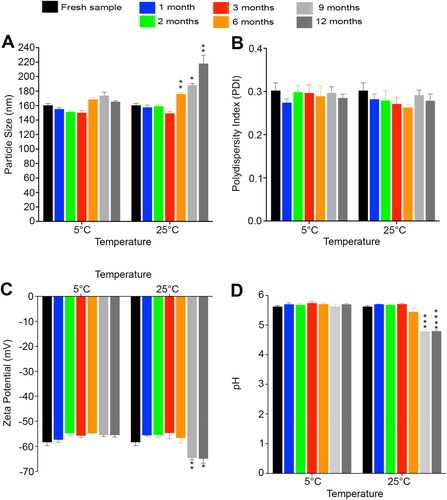
Concerning the zeta potential values of the prepared Gen Tfs that illustrated in and , the fresh Gen Tfs and blank Tfs samples possess high negative zeta potential values of −48.408 ± 1.253 mV and −58.356 ± 1.363 mV, respectively. After 12 months of storage at 5 °C, there were no significant changes in the measured zeta potential values of both medicated (−47.953 ± 0.903 mV) and blank Tfs (−55.547 ± 0.750 mV) (p > .05). Similarly at 25 °C, there were no significant changes in the measured zeta potential values for the first 3 months for medicated Tfs and for the first 6 months for blank Tfs (p > .05). However, there was an observed increase in the measured zeta potential negativity values at 25 °C after 6 months for medicated Tfs (p = .0213) and after 9 months (p = .0081) for blank Tfs as demonstrated in and , respectively. This observed increase in the negativity of the Tfs stored at 25 °C is likely due to the hydrolysis of the lipid contents of the prepared Tfs with the exposure of the negatively charged free carboxylic acid groups at higher temperature (Tamilvanan et al., Citation2010). Advantageously, this increase in the measured zeta potential negativity will provide more physical stability to the Tf formulations. This increase in the zeta potential values will also help to keep a smaller particle size and narrow particle size distribution (PDI) due to the increased and continuous repulsion between adjacent particles in the dispersion (Izak-Nau et al., Citation2015).
Regarding the physical stability of Tf CP940 hydrogels after 12 months of storage at different temperatures, there were no observed changes in the hydrogel physical appearance or consistency at 5 °C for both medicated and blank Tf hydrogels. Similarly, there were no observed changes in the hydrogel physical appearance or consistency at 25 °C for both medicated and blank Tf hydrogels during the 1st nine months of the stability study. After nine months, there was an observed decrease in the hydrogel consistency for both medicated and blank Tf hydrogels. This change in consistency is likely due to the hydrolysis of the lipid contents of the prepared Tfs with the liberation of free fatty acids at higher temperature. These free fatty acids resulted in a decrease in the pH value of the CP940 hydrogels below its pKa value of about 5.5 that resulted in its conversion from gel to sol state as a pH triggered in situ gelling polymer (Gupta and Vyas, Citation2010). This change in the pH values of the hydrogels during the long term storage at 25 °C could be mitigated by preparing the hydrogels in a suitable biocompatible buffering system.
The changes in the pH values of the prepared Tf hydrogels during storage for 12 months at different temperatures were illustrated in and . Initially, the pH values were 5.622 ± 0.036 and 5.608 ± 0.021 for medicated and blank Tf hydrogels, respectively. No significant changes were observed in the pH values of both medicated (5.578 ± 0.083) and blank (5.702 ± 0.031) formulations after storage for 12 months at 5 °C (p > .05). Similarly, at 25 °C, there were no significant changes in the pH values for up to 3 months of storage for the medicated hydrogel and for up to 6 months for the blank hydrogel (p > .05). In contrast, at the end of the study, there was an observed decrease in the pH values of the formulations stored at 25 °C for medicated (5.057 ± 0.077, p = .006) and blank (4.796 ± 0.039, p = .0002) hydrogels, respectively ( and ). This observed decrease in the pH values at elevated temperature is likely due to the release of free fatty acids as a result of hydrolysis and/or oxidative degradation of the transfersomes phospholipid components at higher temperature (Tamilvanan et al., Citation2010). Although there was a decrease in the measured pH values of the formulations stored at 25 °C for 1 year, according to the EEMCO guidelines, the formulations still have ideal pH values for semi-solid formulation intended for topical use (pH 4.5–7) (Novi et al., Citation2023; Parra et al., Citation2003; Lukić et al., Citation2021).
The chemical stability of the medicated CP940 Tf hydrogel, expressed as drug content, is illustrated in . As is evident from our results, there were no significant changes in the formulations drug contents at any time point during the 1 year of storage regardless the storage temperature (p > .05). This improved chemical stability of genistein may be due to its incorporation into this lipid based drug delivery system which provide additional protection for the incorporated active ingredient against possible degradation by oxidation, light and temperature (Matharoo et al., Citation2024). This data obviously indicated the very high chemical stability of genistein in the tested formulations at temperatures up to 25 °C, which recommends its use as a promising topical drug delivery system for the treatment of skin melanoma that could be stored in ambient temperature without any specific storage conditions. But as a more precautionary measure, we recommended the storage of the Tf hydrogels under refrigeration conditions to preserve the integrity of its lipids components and mitigate pH changes at ambient conditions.
4. Conclusion
Melanoma is the most dangerous form of skin cancer. Several reasons are making it more dangerous than other forms of skin cancer including high incidence; high chance of metastasis; high mortality rate; resistance to the available treatment options; and the possible side effects of these treatment options. All these factors make drug market in unmet urgent need for safe and effective treatment for skin melanoma. In the current study we succeeded to prepare, optimize and characterize a topical drug delivery system that meets that urgent need. Genistein, a well-known natural chemotherapeutic agent, was incorporated in a topical transfersome hydrogel to provide a safe and effective antitumor topical drug delivery system for treatment of skin melanoma. Transfersomes were selected as nanocarriers for that purpose because of its ultra-deformable structure that allows for penetration of more drug into deeper layers of the tumor with higher antitumor activity. Our optimized formulations possessed perfect characteristics that enable them to provide excellent antitumor properties such as tiny particle size with narrow particle size distribution, spherical shape and sustained release behavior. Evaluation of the antitumor activity of genistein transfersomes on 3D melanoma spheroids model proves that genistein is a potent chemotherapeutic agent. The antitumor activity of genistein was also improved upon incorporation into transfersomes because of the synergetic effect exerted by the Tfs components that enhanced penetration ability of the drug into the different layers of melanoma spheroids. In addition to the enhanced chemotherapeutic activity, our formulations possess a good shelf-life physical and chemical stability. In conclusion, our genistein transfersome hydrogel could serve as a promising topical drug delivery system for treatment of skin melanoma. In order to support this proposal, further future studies are required for our formulation to be ready for in vivo and/or clinical evaluation. Examples of these future studies is to test the transfersomes safety, efficacy and integrity upon long term storage. Tfs integrity could be tested by evaluating drug encapsulation and drug release behavior of aged formulation.
Authors contributions
Amira Motawea: conception and design, drafting of the paper, final approval of the version to be published, agree to be accountable for all aspects of the work. Sara N. Maria: analysis and interpretation of the data, drafting of the paper, final approval of the version to be published, agree to be accountable for all aspects of the work. Doaa N. Maria: analysis and interpretation of the data, revising the paper critically for intellectual content, final approval of the version to be published, agree to be accountable for all aspects of the work. Monica M. Jablonski: conception and design, revising the paper critically for intellectual content, final approval of the version to be published, agree to be accountable for all aspects of the work. Mohamed Moustafa Ibrahim: conception and design, revising the paper critically for intellectual content, final approval of the version to be published, agree to be accountable for all aspects of the work. Our team has excellent experience in preparation and evaluation of topical pharmaceutical formulations for melanoma treatment.
Acknowledgments
The authors thank Dr. Lawrence Pfeffer (Department of Pathology, University of Tennessee Health Science Center) for providing us with free gift of WM164 skin melanoma cells. Also, the authors thank Dr. TJ Hollingsworth (Department of Ophthalmology, University of Tennessee Health Science Center) for the assistance with the confocal microscope images. The authors thank geniVida™ TG for the free gift of genistein and also the authors thank Lubrizol Advanced Materials for the free gift of Carbopol 940.
Disclosure statement
Dr. Amira Motawea was gifted genistein from geniVida™ TG. Dr. Mohamed Moustafa Ibrahim was gifted Carbopol 940 from Lubrizol Advanced Materials. Dr. Monica Jablonski was gifted WM164 cell line from Dr. Lawrence Pfeffer lab, Department of Pathology, UTHSC. The authors declare no competing interests. Also, the authors declare no competing interests with the organizations or the people providing the free gifted samples of genistein, Carbopol 940 and WM164 cell line. They declare that there is no relationship between the commercial parties (geniVidaTM TG and Lubrizol Advanced Materials) and the author(s) who received the gift free samples mentioned above. Also, the authors declare that the commercial parties (geniVidaTM TG and Lubrizol Advanced Materials) have no role in the experiments.
Data availability statement
The authors confirm that the data supporting the findings of this study are available within the article [and/or] its supplementary materials.
Additional information
Funding
References
- 101Bio. (2024). 3D cell culture gel - Col-Tgel. https://www.101bio.com/P720_3D_cell_culture_gel.php
- Abd El Hady WE, El-Emam GA, Saleh NE, et al. (2023). The idiosyncratic efficacy of spironolactone-loaded PLGA nanoparticles against murine intestinal schistosomiasis. Int J Nanomedicine 18:1–18. doi: 10.2147/IJN.S389449.
- Abd-Allah H, Ragaie MH, Elmowafy E. (2023). Unraveling the pharmaceutical and clinical relevance of the influence of syringic acid loaded linoleic acid transferosomes on acne. Int J Pharm 639:122940. doi: 10.1016/j.ijpharm.2023.122940.
- Abdellatif AAH, El-Telbany DFA, Zayed G, Al-Sawahli MM. (2018). Hydrogel containing PEG-coated fluconazole nanoparticles with enhanced solubility and antifungal activity. J Pharm Innov 14:112–22. doi: 10.1007/s12247-018-9335-z.
- Akl MA, Ryad S, Ibrahim MF, Kassem AA. (2023). Formulation, and optimization of transdermal atorvastatin calcium-loaded ultra-flexible vesicles; ameliorates poloxamer 407-caused dyslipidemia. Int J Pharm 638:122917. doi: 10.1016/j.ijpharm.2023.122917.
- Alhakamy NA, Fahmy UA, Ahmed OAA. (2019). Vitamin E TPGS based transferosomes augmented TAT as a promising delivery system for improved transdermal delivery of raloxifene. PLoS One 14:e0226639. doi: 10.1371/journal.pone.0226639.
- Allam AA, Fathalla D, Safwat MA, Soliman GM. (2022). Transferosomes versus transethosomes for the dermal delivery for minoxidil: preparation and in vitro/ex vivo appraisal. J Drug Delivery Sci Technol 76:103790. doi: 10.1016/j.jddst.2022.103790.
- Andrade LM, de Fátima Reis C, Maione-Silva L, et al. (2014). Impact of lipid dynamic behavior on physical stability, in vitro release and skin permeation of genistein-loaded lipid nanoparticles. Eur J Pharm Biopharm 88:40–7. doi: 10.1016/j.ejpb.2014.04.015.
- Back PI, Furtado LR, Nemitz MC, et al. (2018). Skin permeation and oxidative protection effect of soybean isoflavones from topical nanoemulsions—a comparative study of extracts and pure compounds. AAPS PharmSciTech 19:3029–39. doi: 10.1208/s12249-018-1133-x.
- Caracciolo G. (2018). Clinically approved liposomal nanomedicines: lessons learned from the biomolecular corona. Nanoscale 10:4167–72. doi: 10.1039/c7nr07450f.
- Cevc G. (2004). Lipid vesicles and other colloids as drug carriers on the skin. Adv Drug Deliv Rev 56:675–711. doi: 10.1016/j.addr.2003.10.028.
- Corsaro C, Neri G, Mezzasalma AM, Fazio E. (2021). Weibull modeling of controlled drug release from Ag-PMA nanosystems. Polymers 13:2897. doi: 10.3390/polym13172897.
- Cyto3D™ Live-Dead assay kit pamphlet. (2023). The Well Bioscience Inc.
- Danaei M, Dehghankhold M, Ataei S, et al. (2018). Impact of particle size and polydispersity index on the clinical applications of lipidic nanocarrier systems. Pharmaceutics 10:57. doi: 10.3390/pharmaceutics10020057.
- Davies CGA, Netto FM, Glassenap N, et al. (1998). Indication of the maillard reaction during storage of protein isolates. J Agric Food Chem 46:2485–9. doi: 10.1021/jf9800864.
- de Zampieri ALTC, Ferreira FS, Resende ÉC, et al. (2013). Biodegradable polymeric nanocapsules based on poly (DL-lactide) for genistein topical delivery: obtention, characterization and skin permeation studies. J Biomed Nanotechnol 9:527–34. doi: 10.1166/jbn.2013.1555.
- Demartis S, Rassu G, Murgia S, et al. (2021). Improving dermal delivery of rose Bengal by deformable lipid nanovesicles for topical treatment of melanoma. Mol Pharm 18:4046–57. doi: 10.1021/acs.molpharmaceut.1c00468.
- Elsaied EH, Dawaba HM, Ibrahim EA, Afouna MI. (2016). Investigation of proniosomes gel as a promising carrier for transdermal delivery of Glimepiride. UJPR 1:1–18. doi: 10.22270/ujpr.v1i2.R1.
- Fang JY, Tan SJ, Wu YC, et al. (2016). From competency to dormancy: a 3D model to study cancer cells and drug responsiveness. J Transl Med 14:38. doi: 10.1186/s12967-016-0798-8.
- Fang JY, Tan SJ, Yang Z, et al. (2014). Tumor bioengineering using a transglutaminase crosslinked hydrogel. PLoS One 9:e105616. doi: 10.1371/journal.pone.0105616.
- Ferrado JB, Perez AA, Menegon M, et al. (2023). PEGylation of genistein-loaded bovine serum albumin nanoparticles and its effect on in vitro cell viability and genotoxicity properties. Colloids Surf B Biointerfaces 222:113082. doi: 10.1016/j.colsurfb.2022.113082.
- Gayathri H, Sangeetha S. (2022). Pharmaceutical development of methotrexate loaded transferosomal gel for skin cancer by doe approach. J Pharm Negat Results 13:2456–68.
- Ghasemi Goorbandi R, Mohammadi MR, Malekzadeh K. (2020). Synthesizing efficacious genistein in conjugation with superparamagnetic Fe3O4 decorated with bio-compatible carboxymethylated chitosan against acute leukemia lymphoma. Biomater Res 24:9. doi: 10.1186/s40824-020-00187-2.
- Gupta S, Vyas SP. (2010). Carbopol/chitosan based pH triggered in situ gelling system for ocular delivery of timolol maleate. Sci Pharm 78:959–76. doi: 10.3797/scipharm.1001-06.
- Hady MA, Darwish AB, Abdel-Aziz MS, Sayed OM. (2022). Design of transfersomal nanocarriers of nystatin for combating vulvovaginal candidiasis: a different prospective. Colloids Surf B Biointerfaces 211:112304. doi: 10.1016/j.colsurfb.2021.112304.
- Higuchi T. (1963). Mechanism of sustained‐action medication. Theoretical analysis of rate of release of solid drugs dispersed in solid matrices. J Pharm Sci 52:1145–9.
- Hmingthansanga V, Singh N, Banerjee S, et al. (2022). Improved topical drug delivery: role of permeation enhancers and advanced approaches. Pharmaceutics 14:2818. doi: 10.3390/pharmaceutics14122818.
- Ibrahim MM, Maria DN, Mishra SR, et al. (2019). Once daily pregabalin eye drops for management of glaucoma. ACS Nano 13:13728–44. doi: 10.1021/acsnano.9b07214.
- Insaf A, Parveen R, Srivastava V, et al. (2023). TLC-MS-bioautographic identification of antityrosinase compounds and preparation of a topical gel formulation from a bioactive fraction of an RSM-optimized alcoholic extract of Rubia cordifolia L. stem. J AOAC Int 106:1598–607. doi: 10.1093/jaoacint/qsad076.
- Izak-Nau E, Huk A, Reidy B, et al. (2015). Impact of storage conditions and storage time on silver nanoparticles’ physicochemical properties and implications for their biological effects. RSC Adv 5:84172–85. doi: 10.1039/C5RA10187E.
- Jain S, Patel N, Shah MK, et al. (2017). Recent advances in lipid-based vesicles and particulate carriers for topical and transdermal application. J Pharm Sci 106:423–45. doi: 10.1016/j.xphs.2016.10.001.
- Jandera P, Skeifíková V, Rehová L, et al. (2005). RP-HPLC analysis of phenolic compounds and flavonoids in beverages and plant extracts using a CoulArray detector. J Sep Sci 28:1005–22. doi: 10.1002/jssc.200500003.
- Jangid AK, Solanki R, Patel S, et al. (2022). Genistein encapsulated inulin-stearic acid bioconjugate nanoparticles: formulation development, characterization and anticancer activity. Int J Biol Macromol 206:213–21. doi: 10.1016/j.ijbiomac.2022.02.031.
- Jiang T, Wang T, Li T, et al. (2018). Enhanced transdermal drug delivery by transfersome-embedded oligopeptide hydrogel for topical chemotherapy of melanoma. ACS Nano 12:9693–701. doi: 10.1021/acsnano.8b03800.
- Kamble S, Agrawal S, Cherumukkil S, et al. (2022). Revisiting zeta potential, the key feature of interfacial phenomena, with applications and recent advancements. ChemistrySelect 7:e202103084. doi: 10.1002/slct.202103084.
- Komeil IA, Abdallah OY, El-Refaie WM. (2022). Surface modified genistein phytosome for breast cancer treatment: in-vitro appraisal, pharmacokinetics, and in-vivo antitumor efficacy. Eur J Pharm Sci 179:106297. doi: 10.1016/j.ejps.2022.106297.
- Korsmeyer RW, Gurny R, Doelker E, et al. (1983). Mechanisms of solute release from porous hydrophilic polymers. Int J Pharm 15:25–35. doi: 10.1016/0378-5173(83)90064-9.
- Kumar M, Vinukonda V, Giri A. (2016). A validated RP-HPLC method for simultaneous determination of daidzein, genistein, formononetin and biochanin A from in vitro cultured cells of Trifolium pratense L. (Fabaceae). Der Pharma Chemica 8:16–21.
- Kumari J, Wagener F, Kouwer PHJ. (2022). Novel synthetic polymer-based 3D Contraction assay: a versatile preclinical research platform for fibrosis. ACS Appl Mater Interfaces 14:19212–25. doi: 10.1021/acsami.2c02549.
- Laboratories D. (2023). Cell Counting Kit-8 technical manual. https://www.dojindo.eu.com/TechnicalManual/Manual_CK04.pdf
- Lee J-Y, Kang W-S, Piao J, et al. (2015). Soluplus®/TPGS-based solid dispersions prepared by hot-melt extrusion equipped with twin-screw systems for enhancing oral bioavailability of valsartan. Drug Des Devel Ther 9:2745–56. doi: 10.2147/DDDT.S84070.
- Lockman PR, Koziara JM, Mumper RJ, Allen DD. (2004). Nanoparticle surface charges alter blood-brain barrier integrity and permeability. J Drug Target 12:635–41. doi: 10.1080/10611860400015936.
- Luiz MT, Viegas JSR, Abriata JP, et al. (2021). Docetaxel-loaded folate-modified TPGS-transfersomes for glioblastoma multiforme treatment. Mater Sci Eng C Mater Biol Appl 124:112033. doi: 10.1016/j.msec.2021.112033.
- Lukić M, Pantelić I, Savić SD. (2021). Towards optimal pH of the skin and topical formulations: from the current state of the art to tailored products. Cosmetics 8:69. doi: 10.3390/cosmetics8030069.
- Mady OY, Donia AA, Al-Shoubki AA, Qasim W. (2019). Paracellular pathway enhancement of metformin hydrochloride via molecular dispersion in Span 60 microparticles. Front Pharmacol 10:713. doi: 10.3389/fphar.2019.00713.
- Maeda H. (2015). Toward a full understanding of the EPR effect in primary and metastatic tumors as well as issues related to its heterogeneity. Adv Drug Deliv Rev 91:3–6. doi: 10.1016/j.addr.2015.01.002.
- Maji R, Omolo CA, Jaglal Y, et al. (2021). A transferosome-loaded bigel for enhanced transdermal delivery and antibacterial activity of vancomycin hydrochloride. Int J Pharm 607:120990. doi: 10.1016/j.ijpharm.2021.120990.
- Malviya N, Prabakaran A, Alexander A. (2023). Comparative study on ethosomes and transferosomes for enhancing skin permeability of sinapic acid. J Mol Liq 383:122098. doi: 10.1016/j.molliq.2023.122098.
- Manickavasagam D, Novak K, Oyewumi MO. (2018). Therapeutic delivery of simvastatin loaded in PLA-PEG polymersomes resulted in amplification of anti-inflammatory effects in activated microglia. Aaps J 20:18. doi: 10.1208/s12248-017-0176-3.
- Mason R, Au L, Ingles Garces A, Larkin J. (2019). Current and emerging systemic therapies for cutaneous metastatic melanoma. Expert Opin Pharmacother 20:1135–52. doi: 10.1080/14656566.2019.1601700.
- Matharoo N, Mohd H, Michniak-Kohn B. (2024). Transferosomes as a transdermal drug delivery system: dermal kinetics and recent developments. Wiley Interdiscip Rev Nanomed Nanobiotechnol 16:e1918. doi: 10.1002/wnan.1918.
- Meteoglu I, Erdemir A. (2021). Genistein and Temozolomide-loaded polymeric nanoparticles: a synergistic approach for improved anti-tumor efficacy against glioblastoma. Process Biochem 110:9–18. doi: 10.1016/j.procbio.2021.07.015.
- Miatmoko A, Marufah NA, Nada Q, et al. (2022). The effect of surfactant type on characteristics, skin penetration and anti-aging effectiveness of transfersomes containing amniotic mesenchymal stem cells metabolite products in UV-aging induced mice. Drug Deliv 29:3443–53. doi: 10.1080/10717544.2022.2149895.
- Mittal P, Vrdhan H, Ajmal G, et al. (2019). Formulation and characterization of genistein-loaded nanostructured lipid carriers: pharmacokinetic, biodistribution and in vitro cytotoxicity studies. Curr Drug Deliv 16:215–25. doi: 10.2174/1567201816666181120170137.
- Novi L, Iskandarsyah A, Aulia J. (2023). Stability of high concentration capsaicin in transfersome carriers in gel dosage form. MUDIMA 3:153–9. doi: 10.55927/mudima.v3i1.2316.
- Omar MM, Hasan OA, El Sisi AM. (2019). Preparation and optimization of lidocaine transferosomal gel containing permeation enhancers: a promising approach for enhancement of skin permeation. Int J Nanomedicine 14:1551–62. doi: 10.2147/IJN.S201356.
- Ortiz R, Cabeza L, Perazzoli G, et al. (2019). Nanoformulations for glioblastoma multiforme: a new hope for treatment. Future Med Chem 11:2459–80. doi: 10.4155/fmc-2018-0521.
- Parkash V, Maan S, Chaudhary V, et al. (2018). Implementation of design of experiments in development and optimization of transfersomal carrier system of tacrolimus for the dermal management of psoriasis in albino wistar rat. J Bioequiv Availab 10:99–106. doi: 10.4172/0975-0851.1000385.
- Parra JL, Paye M, Group E. (2003). EEMCO guidance for the in vivo assessment of skin surface pH. Skin Pharmacol Appl Skin Physiol 16:188–202. doi: 10.1159/000069756.
- Prajapat VM, Mahajan S, Paul PG, et al. (2023). Nanomedicine: a pragmatic approach for tackling melanoma skin cancer. J Drug Delivery Sci Technol 83:104394. doi: 10.1016/j.jddst.2023.104394.
- Putri DCA, Dwiastuti R, Marchaban M, Nugroho AK. (2017). Optimization of mixing temperature and sonication duration in liposome preparation. JPharmSciCommunity 14:79–85. doi: 10.24071/jpsc.142728.
- Rai S, Pandey V, Rai G. (2017). Transfersomes as versatile and flexible nano-vesicular carriers in skin cancer therapy: the state of the art. Nano Rev Exp 8:1325708. doi: 10.1080/20022727.2017.1325708.
- Ramkanth S, Anitha P, Gayathri R, et al. (2021). Formulation and design optimization of nano-transferosomes using pioglitazone and eprosartan mesylate for concomitant therapy against diabetes and hypertension. Eur J Pharm Sci 162:105811. doi: 10.1016/j.ejps.2021.105811.
- Rassu G, Porcu EP, Fancello S, et al. (2018). intranasal delivery of genistein-loaded nanoparticles as a potential preventive system against neurodegenerative disorders. Pharmaceutics 11:8. doi: 10.3390/pharmaceutics11010008.
- Raval A, Bahadur P, Raval A. (2018). Effect of nonionic surfactants in release media on accelerated in-vitro release profile of sirolimus eluting stents with biodegradable polymeric coating. J Pharm Anal 8:45–54. doi: 10.1016/j.jpha.2017.06.002.
- Sabry S, El Hakim Ramadan A, Abd Elghany M, et al. (2021). Formulation, characterization, and evaluation of the anti-tumor activity of nanosized galangin loaded niosomes on chemically induced hepatocellular carcinoma in rats. J Drug Delivery Sci Technol 61:102163. doi: 10.1016/j.jddst.2020.102163.
- Salama A, Badran M, Elmowafy M, Soliman GM. (2019). Spironolactone-loaded leciplexes as potential topical delivery systems for female acne: in vitro appraisal and ex vivo skin permeability studies. Pharmaceutics 12:25. doi: 10.3390/pharmaceutics12010025.
- Sharma M, Malik G, Gulati D, et al. (2022). Formulation and evaluation of fusidic acid based transferosome for burn wound infection. Mater Today: Proc 68:836–41. doi: 10.1016/j.matpr.2022.06.260.
- Sharma PK, Chauhan MK. (2020). Optimization and evaluation of encapsulated brimonidine tartrate-loaded nanoparticles incorporation in situ gel for efficient intraocular pressure reduction. J Sol-Gel Sci Technol 95:190–201. doi: 10.1007/s10971-020-05305-z.
- Skazik C, Wenzel J, Marquardt Y, et al. (2011). P-glycoprotein (ABCB1) expression in human skin is mainly restricted to dermal components. Exp Dermatol 20:450–2. doi: 10.1111/j.1600-0625.2010.01237.x.
- Song Y-y, Yuan Y, Shi X, Che Y-y. (2020). Improved drug delivery and anti-tumor efficacy of combinatorial liposomal formulation of genistein and plumbagin by targeting Glut1 and Akt3 proteins in mice bearing prostate tumor. Colloids Surf B Biointerfaces 190:110966. doi: 10.1016/j.colsurfb.2020.110966.
- Srivastava V, Singh V, Khatri DK, Mehra NK. (2023). Recent trends and updates on ultradeformable and elastic vesicles in ocular drug delivery. Drug Discov Today 28:103647. doi: 10.1016/j.drudis.2023.103647.
- Sun Y, Zhang Y, Liu X, et al. (2023). The preparation of high minoxidil loaded transfersomes and its gel for effective topical treatment of alopecia. J Drug Delivery Sci Technol 84:104458. doi: 10.1016/j.jddst.2023.104458.
- Tamilvanan S, Kumar BA, Senthilkumar SR, et al. (2010). Stability assessment of injectable castor oil-based nano-sized emulsion containing cationic droplets stabilized by poloxamer-chitosan emulsifier films. AAPS PharmSciTech 11:904–9. doi: 10.1208/s12249-010-9455-3.
- Tsakiri M, Ghanizadeh Tabriz A, Naziris N, et al. (2024). Exosome-like genistein-loaded nanoparticles developed by thin-film hydration and 3D-printed Tesla microfluidic chip: a comparative study. Int J Pharm 651:123788. doi: 10.1016/j.ijpharm.2024.123788.
- Ungar Y, Osundahunsi OF, Shimoni E. (2003). Thermal stability of genistein and daidzein and its effect on their antioxidant activity. J Agric Food Chem 51:4394–9. doi: 10.1021/jf034021z.
- van den Bergh BA, Wertz PW, Junginger HE, Bouwstra JA. (2001). Elasticity of vesicles assessed by electron spin resonance, electron microscopy and extrusion measurements. Int J Pharm 217:13–24. doi: 10.1016/s0378-5173(01)00576-2.
- Verma DD, Verma S, Blume G, Fahr A. (2003). Particle size of liposomes influences dermal delivery of substances into skin. Int J Pharm 258:141–51. doi: 10.1016/s0378-5173(03)00183-2.
- Vodnik VV, Mojić M, Stamenović U, et al. (2021). Development of genistein-loaded gold nanoparticles and their antitumor potential against prostate cancer cell lines. Mater Sci Eng C Mater Biol Appl 124:112078. doi: 10.1016/j.msec.2021.112078.
- Wang G, Kuan SS, Francis OJ, et al. (2002). A simplified HPLC method for the determination of phytoestrogens in soybean and its processed products. J Agric Food Chem 38:185–90. doi: 10.1021/jf00091a041.
- Wang Y, Wang Y, Liu Y, et al. (2018). Preparation, characterization, and antioxidant activities of cellulose nanocrystals/genistein nanocomposites. BioRes 14:336–48. doi: 10.15376/biores.14.1.336-348.
- Xiao Y, Ho C-T, Chen Y, et al. (2020). Synthesis, characterization, and evaluation of genistein-loaded zein/carboxymethyl chitosan nanoparticles with improved water dispersibility, enhanced antioxidant activity, and controlled release property. Foods 9:1604. doi: 10.3390/foods9111604.
- Yang C, Wu T, Qi Y, Zhang Z. (2018). Recent advances in the application of vitamin E TPGS for drug delivery. Theranostics 8:464–85. doi: 10.7150/thno.22711.
- Yin L, Chen Y, Zhang Z, et al. (2015). Biodegradable micelles capable of mannose‐mediated targeted drug delivery to cancer cells. Macromol Rapid Commun 36:483–9. doi: 10.1002/marc.201400650.