Abstract
It has earlier been hypothesized that intravascular microbubbles, derived from a dodecafluoropentane (DDFP) emulsion, can transport physiologically significant amounts of oxygen in the animal body. To test this notion, anesthetized oxygen breathing rats were rendered severely anemic by bleeding and volume replacement. Rats treated with 0.014 ml/kg of DDFP in a 2% emulsion had normal circulatory parameters and behaved normally when waking up from anesthesia while controls died during anesthesia. Oxygen-breathing intact rats given 0.01 ml/kg of DDFP had muscle oxygen tensions which, for about 2.5 hours, exceeded those of controls by 50–100%. It was further verified in vitro that DDFP-derived microbubbles can exchange oxygen with a surrounding aqueous medium. Extrapolation from these experiments indicates that less than 1 ml of DDFP, in emulsion-form, could provide for the resting oxygen consumption of an adult person. This suggests various therapeutic uses of the emulsion.
INTRODUCTION
The presence of gas bubbles in the circulation is generally considered medical anathema because it may cause embolic manifestations such as encountered, for instance, in transfusion accidents and divers' bends (decompression sickness). However, based on theoretical modeling, it has been proposed that intravascular bubbles that are subcapillary in size can transport physiologically significant amounts of oxygen [Citation[1]]. It has further been proposed that such bubbles preferably should be generated with a poorly soluble gas, such as dodecafluoropentane (DDFP), [Citation[1]]. The function of the poorly soluble gas is to stabilize the volume of microbubbles which, without this gas component, would rapidly disappear into solution as dictated by Laplace's Law.
Intravascular microbubbles volume-stabilized by DDFP gas are formed when a DDFP-emulsion is injected into the circulation of homeothermic animals and humans. The DDFP has a boiling point of ∼29°C at atmospheric pressure and the particles in this emulsion undergo phase shift and form bubbles when warmed in the bloodstream [Citation[2]]. Initial bubble size depends on the size of the emulsion particles but, theoretically, the bubbles undergo size variations as they exchange oxygen, nitrogen and carbon dioxide by diffusion in the lungs and the tissues [Citation[1], Citation[3]]. However, the bubbles are small enough to pass the pulmonary and systemic capillary beds, which is conditional for the preparation's demonstrated usefulness as an ultrasound contrast medium [Citation[2], Citation[4]]. In the present study such an emulsion was injected into the circulation of rats to test if it could enhance oxygen transport.
The present experiments demonstrated how, without clinical or histological signs of embolism, microbubbles formed by the i.v. infusion of very small amounts of DDFP in a 2% emulsion, combined with oxygen breathing, provided very protective oxygenation in a condition of potentially lethal generalized hypoxia caused by anemia induced by isovolumetric hemodilution. That the preparation can boost local muscle oxygen tension was also shown in intact rats. Additionally, an in vitro experiment was performed to verify that bubbles formed from DDFP-emulsion could absorb oxygen from a surrounding aqueous medium.
METHODS
The preparation used in the present experiments consists of an aqueous nano-emulsion of DDFP. This intravenous injectable contains 0.3% weight/volume (w/v), PEG telomer-B, 0.3% w/v Pluronic P123 and 30% w/v sucrose in addition to 2% DDFP w/v. The mean particle size (intensity weighted) is 292 nm in diameter. Furthermore, 99% of the particles in the formulation are smaller than 626 nm. Thus, the formulation manufactured and stored under pressure does not contain a significant number of particles in the micrometer range [Citation[5]].
The experimental protocol was approved by the University at Buffalo's Institutional Animal Care and Use Committee. Male Wistar rats weighing 350–400 g were used.
Four series of experiments were performed:
Treatment of potentially fatal anemia in rats so as to preserve vital circulatory function in acute experiments.
Treatment of potentially fatal anemia in rats for long term survival.
Boosting of muscle oxygen tension by treating (top loading) intact rats (acute experiments).
Measurement of changes in oxygen content in water baths at temperatures of 20°C and 37°C, respectively, in response to injections of DDFP-emulsion and emulsion vehicle into the water.
Series I. The anesthetized (pentobarbital, 50 mg/kg i.p.) rats were instrumented for recording of arterial and central venous blood pressures and rectal temperature. The latter was controlled at 37±0.5°C by keeping the supine rat on an electric heating pad. Two syringes on an infusion pump were connected, one to a carotid artery for withdrawal of blood and one to the abdominal caval vein for infusion of a plasma expander (7% albumin in lactated Ringer's solution) and either a 2% DDFP-emulsion or emulsion vehicle (Sonus Pharmaceuticals, Inc., Bothell, WA). Arterial pressure was recorded in a tail artery with a pressure transducer (Model CDX III, Cobe Laboratories, Inc., Lakewood, CO). Through an incision in the skin, a combination electrode (Kontron Instruments, Zürich, Switzerland) for recording tissue oxygen and carbon dioxide tensions was placed on an abdominal muscle. All data were continuously monitored using a multi-channel recorder. The spontaneously breathing rat was supplied with 70% oxygen (balance nitrogen) via a mask. Blood was withdrawn and plasma expander infused simultaneously at a rate of 0.4 ml/min. Arterial blood samples were drawn every 15 min during the hemodilution period for measurement of hemoglobin (Hb) concentrations. When the Hb had declined to about 50%, i.v. infusion, at 0.05 ml/min, of emulsion vehicle was started in the controls (n = 8) and 2% DDFP-emulsion in treatment animals (n = 8). The total dose of DDFP-emulsion was 0.7 ml/kg body weight (equivalent to 0.014 ml of DDFP/kg). The infusion lasted 60 min or less in the animals that died (controls only). Despite having Hb-levels about half of those which were fatal in the controls the treatment animals did not die spontaneously, and they were euthanized after being monitored for 2–3 hrs. Postmortem tissue samples were taken from the brain, heart, lungs, kidneys, adrenals, stomach, spleen, liver and testicles. The samples were fixed in 4% buffered formaldehyde solution, stained with hematoxylin-eosin and examined with light microscopy.
Series II. Preparation and procedures on these animals (eight controls and eight treated) were the same as in Series I, with some exceptions dictated by the intention to let them wake up from anesthesia. Thus, they were anesthetized with fentanyl 0.16 mg/kg i.m. and midazolam hydrochloride 1 mg/kg i.m. while the 70% oxygen gas mixture was provided via mask during the hemodilution and treatment phases. All control animals died during anesthesia. For awakening, the treatment animals were placed in an acrylic cage flushed with 100% oxygen. The invasive procedures consisted of introducing a catheter in the caval vein for infusions, one catheter in a carotid artery for blood removal and another catheter, in the tail artery, for blood pressure recordings. These three catheters were routed under the skin to an incision on the neck and, after surgery, out through an opening on top of the acrylic cage. Having awakened at about the time when the hemodilution and infusion of DDFP-emulsion were complete, the treatment animals were observed for approximately 130 min. They were then transfused with part of their shed blood so as to restore roughly 50% of their normal Hb concentration while avoiding severe hypervolemia. With the catheters secured in place, the animals were returned to air-vented cages. Blood pressure was measured daily for eight days. Twenty-one days after the hemodilution process, euthanasia was performed and tissue samples prepared and examined as in Series I.
Series III. The pentobarbital anesthetized rats were instrumented for arterial blood pressure recording and venous infusions, as well as for recording of oxygen and carbon dioxide tensions on the surface of an abdominal muscle. Breathing spontaneously through a mask, the animals were switched from air to oxygen breathing. Infusion of Ringer's solution (1.0 ml/kg/min) was begun 15 min later and, after an additional 15 min, i.v. infusions of either the 2% DDFP-emulsion or emulsion vehicle were started. A total dose of 0.5 ml/kg of DDFP-emulsion (equivalent to 0.010 ml DDFP) was given over 30 min in treatment (top loaded) animals (n = 4) as was a corresponding dose of preparation vehicle in control animals (n = 4). The Ringer infusion was terminated at the same time as the emulsion and vehicle infusions. Recordings were carried out for a total of 3 hrs and 20 min after which the animals were euthanized with pentobarbital.
Series IV. These in vitro experiments were made as a qualitative verification that, as microbubbles are formed by warming a sample of the 2% DDFP solution, they are able to take up oxygen previously physically dissolved in a surrounding aqueous medium.
Distilled water was filled into two 250 ml glass beakers and gently agitated by a magnetic stirrer (so as to avoid imbibement of air bubbles from the surface). One beaker was allowed to equilibrate with room air at 20°C and another beaker with room air while the water temperature was kept at 37°C by means of a electrical warming jacket controlled by a thermostat. The measuring probe of an Oxan meter (Model 600, Engineered Systems & Designs, Inc., Newark, DE) for recording oxygen content was inserted into the water in the beakers and readings taken every 5 min until several stable readings indicated that oxygen exchange equilibrium between ambient air and the water had been reached. A paraffin film disc was floated on the water so as to shield the water interface from further gas exchange with the ambient air. A notch in the perimeter of the disc accommodated the cable to the submersed meter probe and allowed injections into the water of the test samples. In one set of experiments the water in the beakers was injected with 5 ml of the 2% DDFP-emulsion and readings of physically dissolved oxygen content taken every 5 min for up to about 2 hrs. In another set of experiments the same protocol was followed with the exception that, instead of the DDFP-emulsion, 5 ml of preparation vehicle was injected into the water. Each type of experiment was repeated six times.
Statistics: The data were tested for normal distribution by Anova analysis, and statistical analysis was performed by student's t-test, with p ≤ 0.05 considered significant. All values are given as mean±SE (standard error of the mean).
RESULTS
The reductions in Hb concentrations resulting from the hemodilution procedure in Series I and II were identical in controls and treatment animals (). The control animals (in both Series I and II) died 90–115 min after the start of the hemodilution process. At death, their Hb concentrations averaged 2.8 ± 0.1 g/100 ml. The hemodilution in the two treatment groups (n = 16) was intentionally made more pronounced and, when it was terminated, the Hb levels, at 1.3 ± 0.1 g/100 ml (Series I) and 1.6 ± 0.2 g/100 ml (Series II), were significantly lower than in the controls (p < 0.001).
Figure 1 Series I and II. Hemoglobin concentration versus time (all data points are mean values + or − SE) during isovolumetric hemodilution (bleeding and volume replacement) in control rats (square symbols; n = 16) receiving vehicle i.v. and rats treated with 0.7 ml/kg i.v. of a 2% dodecafluoropentane emulsion (DDFPe); treatment animals kept anesthetized until euthanasia (triangles; n = 8) and treatment animals allowed to wake up (circles; n = 8). All animals breathed high concentrations of oxygen per schedule at top of .
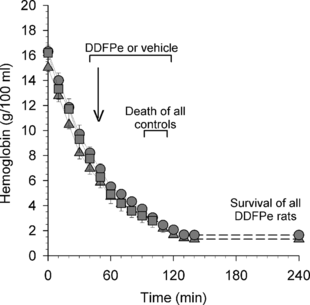
The mean arterial pressures of the two control groups combined and of the two treatment groups are shown in . After a brief increase in blood pressure, coincident with the start of breathing 70% oxygen, the pressure in the controls declined continuously from the beginning of the hemodilution until death. By contrast, again after a brief peak, the blood pressure in all the treatment animals in Series I remained at a normal level throughout the 125 min hemodilution period and the subsequent 125 min observation period, which was terminated with euthanasia. In the treatment animals allowed to wake up (Series II), a similar pattern was recorded except that, during the last two thirds of the awake period in the oxygen-flushed cage, there was a tendency for a slight increase in blood pressure. An additional, more marked, increase was seen in response to the retransfusion with whole blood. The retransfusion generated an Hb level of 7.2 ± 0.2 g/100 ml. During the subsequent eight days, the blood pressure and Hb concentration became normal.
Figure 2 Series I and II. Mean arterial pressure vs. time in rats made anemic by hemodilution (same animals as ; all data points are mean values + or − SE). Control rats (squares; n = 16) received vehicle i.v. and treatment animals received 0.7 ml/kg i.v. of 2% dodecafluoropentane emulsion (DDFPe); treatment animals kept anesthetized until euthanasia (triangles, n = 8) and treatment animals allowed to wake up (circles, n = 8). All animals breathed high concentrations of oxygen per schedule at top of figure.
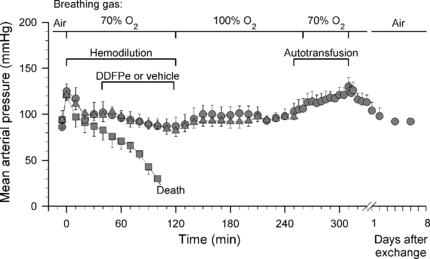
The central venous pressures in the eight control animals and eight treated animals in Series I are shown in . There was an increase in pressure in both groups but no difference between them that can be ascribed to the infusion of the DDFP-emulsion.
Figure 3 Central venous pressure (mean, + or − SE) vs. time in control rats (squares; n = 8) and treated rats of Series I (triangles; n = 8). Each data point represents the maximum value read at end-expiration.
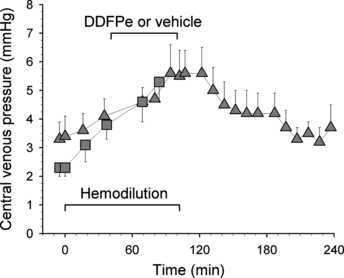
The oxygen tensions in the abdominal muscles of the permanently anesthetized control (n = 8) and treatment animals (n = 8) in Series I are shown in . During air breathing the oxygen tension was 61 ± 5 mm Hg (both groups shown together), but with administration of 70% oxygen the muscle oxygen tension rose sharply to about 135 mm Hg, after which it declined identically in the two groups until treatment began 40 min into the hemodilution period. From that point the muscle oxygen tensions in the controls fell steadily until death. By contrast, the muscle oxygen tension in treatment animals, after a slow decline, stabilized at about 36 mm Hg some 30 min after completion of treatment. This level was only slightly lower than the level recorded during air breathing before hemodilution.
Figure 4 Series I and II. Oxygen tensions in abdominal muscle vs. time in rats made anemic by hemodilution (same animals as in and ; all data points are mean values + or − SE). Control rats (squares; n = 16) received vehicle and permanently anesthetized rats (triangles; n = 8) received 0.7 ml/kg i.v. of 2% dodecafluoropentane emulsion (DDFPe). The animals breathed high oxygen concentrations per schedule at top of .
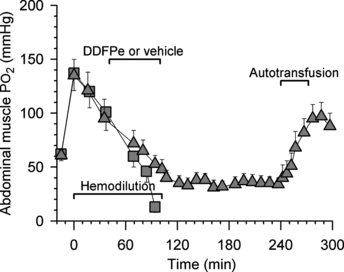
Muscle carbon dioxide tension in controls and treatment animals, before hemodilution, was 62 ± 3 mm Hg and after hemodilution it stabilized at 57 ± 1 mm Hg. Following DDFP treatment the muscle carbon dioxide fell gradually and leveled off at between 50 and 55 mm Hg during the last 1.5 hours of observation.
Between 30 and 45 min after the first righting response, all the treatment animals in Series II appeared fully awake and normal (except for having white eyes). They moved about in the cage, responded to sound and light, groomed, ate and drank.
Other than low intravascular concentrations of erythrocytes in Series I, no histological abnormalities were seen in the tissue specimens taken after euthanasia in treatment animals in Series I and II. The controls had some interstitial pulmonary edema.
The muscle oxygen tensions in the animals that were top-loaded with DDFP-emulsion and in the controls of Series III are shown in . Oxygen tensions during air breathing were 50 ± 5 mm Hg in control animals, and 49 ± 8 mm Hg in treatment animals. During the first 25 min of oxygen breathing, the readings stabilized at 120 ± 11 and 121 ± 3 mm Hg, respectively. From then on the oxygen tension remained essentially stable in the controls. In the treatment animals, the infusion of DDFP-emulsion caused a rapid rise in the muscle oxygen tension reaching levels 40–100% higher than in the controls. About 2 hrs after the start of the infusion (1.5 hr after its completion), the muscle oxygen tension began to decline and about 50 min later it had reached the control level.
Figure 5 Series III. Oxygen tensions (filled symbols) and carbon dioxide tensions (open symbols) vs. time in abdominal muscle of hematologically normal rats breathing oxygen (all data points are mean values + or − SE). Control rats (squares; n = 4) received vehicle plus Ringer's solution i.v. and treatment rats (circles; n = 4) received 0.5 ml/kg i.v. of a 2% dodecafluoropentane emulsion (DDFPe) plus Ringer's solution.
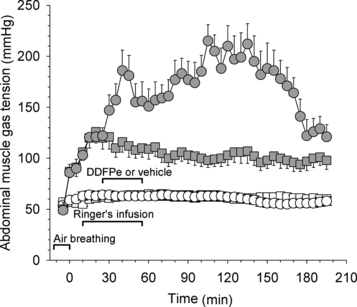
In the in vitro experiments in Series IV the absolute values for oxygen content in the equilibrated water was 5.66 ± 0.13 ppm (mean ± SE; 35 data points) at 20°C and 4.20 ± 0.17 ppm (21 data points) at 37°C. The changes relative to these control readings that were caused by the addition of DDFP-emulsion and vehicle solution, respectively, are shown in . The drop in the amount of physically dissolved oxygen in the water as some of this oxygen diffuses into the bubbles was clearly more pronounced than in any of the other conditions, not conducive to bubble formation. Note that in the curve representing the results after injection of DDFP-emulsion there is a tendency for the oxygen content in the water to increase after about 70 min.
Figure 6 Upper panel: Change in content of physically dissolved oxygen in 250 ml of distilled water at 20°C (upper panel) or 37°C, versus time, when injected with 5 ml of a 2% DDFP-emulsion at time 0 (circles; all data points are mean values + or − SE; n = 3) or 5 ml of preparation vehicle (triangles; n = 3); before time 0 water only (diamonds; n = 3). For further details see text.
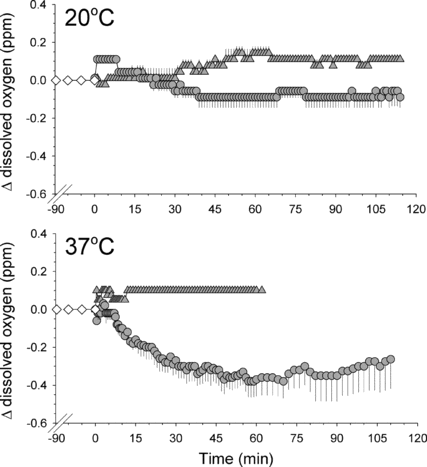
DISCUSSION AND CONCLUSIONS
The fundamental concept that intravascular microbubbles can significantly enhance circulatory oxygen transport to the tissues was supported by this study. This notion has furthermore been strengthened by other researchers who have reported that, in rats breathing carbogen (95% O2, 5% CO2), an i.v. dose of 0.75 ml/kg of the DDFP-emulsion completely reversed the hypoxic radio-resistance of hepatoma cells [Citation[6]]. That dose is essentially the same as the presently used 0.7 ml/kg.
The ability of the DDFP derived microbubbles to exchange oxygen with a surrounding aqueous medium was demonstrated by the in vitro experiments (Series IV), which showed a decline in the physically dissolved oxygen in the water at 37°C upon injection of the emulsion, the bubbles receiving oxygen from the water by diffusion. Predictably, the injection of the vehicle had no such effect. Moreover, the tendency for an increase in the oxygen content of the water during the last 70 min of observation in the former experiments may have been due to retrograde movement of oxygen as the poorly soluble DDFP gas, and with it the oxygen, slowly diffused out of the bubbles into the water. In water at 20°C, which is well below the phase-transition temperature of 29°C for DDFP, no bubble formation would be expected and no significant effects on oxygen content in the water were seen after injection of the emulsion.
As mentioned earlier, the bubbles pass through the pulmonary and systemic capillaries. Thus, it stands to reason that the bubbles receive oxygen by diffusion from the alveolar space, via the plasma, and deliver the oxygen by the same process to the tissues. The universality of this principle of gas transport has recently been demonstrated using the same preparation to enhance nitrogen transport from the tissues to the lungs in oxygen breathing pigs [Citation[7]]. The notion that the nitrogen transfer was boosted by nitrogen transport in the microbubbles, and not by circulatory changes, is strongly supported by the observation that the cardiac output, an important determinant in circulatory transport of poorly soluble gases such as nitrogen [Citation[8]], was unaffected by the administration of the DDFP preparation [Citation[7]].
The circulatory derangement, tissue hypoxia and 100% mortality caused by severe acute anemia in oxygen-breathing rats were eliminated by i.v. infusion of microbubbles derived from very small doses of DDFP in a 2% emulsion. Indeed, since it can be calculated that about 30% of the DDFP dose was lost with the shed blood, the effective dose in Series I and II was about 0.01 ml/kg. However, allowance should be made for the oxygen-carrying capacity of the Hb (∼ 10% of normal) remaining in the circulation after the hemodilution, i.e. the DDFP made up for 90% of the oxygen capacity lost with the shed erythrocytes. Given these considerations, and the fact that the relative metabolic rate (per unit of weight) is 10 times higher in rats than in humans [Citation[9]], one arrives at the striking prediction that the resting oxygen consumption of an oxygen-breathing 75 kg person could be supported by a total dose of DDFP of 0.8 ml in emulsion form. This supports the notion that, for equal amounts of fluorocarbon, DDFP (in bubble form) is several hundred times more efficient as an oxygen transporter than the liquid fluorocarbons (such as perfluorochtylbromide) in “conventional” oxygen carrying emulsions in which the oxygen is kept in physical solution [Citation[1]]. It seems that the steady-state oxygen consumption of rats could be supported by even smaller volumes of circulating DDFP gas than the total volume first generated by the current DDFP doses. This is so because the amount of circulating DDFP must have declined over the observation periods (≈ 2 hours) due to elimination from the blood stream. Such elimination occurs via expired gases [Citation[2]] as well as by some short-lasting redistribution to various tissue compartments [Citation[10]]. However, because of the high volatility of DDFP, it should be eliminated relatively rapidly. This contrasts with the “conventional” fluorocarbon-based blood substitutes from which residues of fluorocarbon have been demonstrated both in reticuloendothelial cells in the liver and in lung alveoli [Citation[10]].
As for deleterious effects of gas embolism, none were observed during the autopsies or in the histological specimens, neither in the measurements of muscle oxygen tensions or central venous pressure, which would be expected to be abnormally high if significant pulmonary embolization had occurred. Emulsion doses as large as 2.0 ml/kg distributed to dogs in 4 injections over a 1.5 hr period have been used for myocardial contrast echocardiography without any significant deterioration in myocardial micro circulation or hemodynamic parameters or arterial oxygen saturation [Citation[11]].
The present study did not attempt to directly measure the longevity of the intravascular bubbles. However, it stands to reason that as long as a positive physiological effect could be observed, enough bubbles were still present so as to satisfy basic oxygen needs. This lasted at least two hours in Series I and II in which the observation periods were intentionally limited and the increased muscle oxygen tensions lasted about 2.5 hrs in the top-loaded intact rats in Series III. Predictions of bubble life span in the circulation based on the time that useful ultrasound images can be seen after injection of the preparation are quite varied, e.g. 5 min [Citation[13]] to 30 min [Citation[14]]. However, such predictions may underestimate what the bubble lifespan might be in the absence of ultrasound since ultrasound may relatively rapidly cause break-up and elimination of the bubbles [Citation[15]]. Nonetheless, practical use of this new method of oxygenation would often call either for increased doses or repeated dosing.
A multitude of conditions in which circulatory oxygen transport is inadequate offer themselves to be treated according to the principles demonstrated in the present experiments. Moreover, enhancement of blood oxygen transport above normal levels by top loading (as described in Series III) oxygen breathing patients with an emulsion of microbubbles may benefit conditions that are currently subject to the relatively cumbersome administration of hyperbaric oxygen in pressure chambers. Furthermore, preservation of organs, intended for transplantation, may be helped by perfusion with an oxygenated emulsion of microbubbles.
Among the advantages of the microbubble preparation, as an erythrocyte replacement, are that it does not require blood typing, it eliminates the sterility problems of whole blood, it exposes the recipient to very small amounts of foreign substance, and it has an extremely low bulk, which is important in military medicine and medical disaster scenarios.
Relevant to the potential use of the preparation in humans is that in 1997 the preparation had been injected in bolus doses of up to 0.35 ml/kg in 743 patients/volunteers without significant adverse effects [Citation[12]] and the apparent safety was further confirmed in subsequent studies using bolus doses of between 0.05 and 0.12 ml/kg on an additional more than 1200 subjects [Citation[5]].
The authors thank Drs. Dillip Worah and Hugh Van Liew for useful and stimulating discussions and Dr. Peter Nickerson for histological examination of tissue samples. Skilled technical assistance by Ms. Kimberly Grant and Mr. Curtis J. Senf is gratefully acknowledged. This research was partially supported by the Department of Physiology, School of Medicine, University of Bergen, Norway, by the Research Council of Norway, Division of Medicine and Health, by Sonus Pharmaceuticals Inc., Bothell, Washington, USA, and by U.S. Army Medical Research Acquisition Activity Grant “Volume Stabilized Intravascular Microbubbles for Circulatory Transport of Oxygen and Carbon Dioxide: A Field-Usable Concept” (ID: DAMD 170010514).
REFERENCES
- Burkard, M.E., Van Liew, H.D. (1994). J. Appl. Physiol. 77: 2874–2878. [INFOTRIEVE], [CSA]
- Correas, J.-M., Quay, S.D. (1996). Clinical Radiology. 51(Suppl. 1): 11–14. [INFOTRIEVE], [CSA]
- Van Liew, H.D., Burkard, M.E. (1996). J. Appl. Physiol. 81: 500–508. [INFOTRIEVE], [CSA]
- Lopez-Ben, R., Robin, M.L., Weber, T.M., Smith, J.K., Needleman, L., Berland, L.L. (1999). J. Ultrasound Med. 18: 109–1169. [INFOTRIEVE], [CSA]
- Low, M. Sonus Pharmaceuticals, Inc., Bothell, Washington. Personal communication.
- Koch, J.C., Oprysko, P.R., Shuman, A.L., Jenkins, W.T., Brandt, G., Evans, S.M. (2002). Cancer Research 62: 3626–3629. [INFOTRIEVE], [CSA]
- Lundgren, C., Bergoe, G., Olszowka, A., Tyssebotn, I. (2005). Undersea and Hyperbaric Medicine 32: 215–226. [INFOTRIEVE], [CSA]
- Farhi, L. (1967). Resp. Physiol. 3: 1–11. [CSA], [CROSSREF]
- Spector William, S. (Ed.). (1961). Handbook of Biological Data, Division of Biology and Agriculture, The National Academy of Sciences, The National Research Council, W.B. Saunders Company: Philadelphia.
- Zuck, T.F., Riess, J.G. (1994). Critical Reviews in Clinical Laboratory Sciences 31: 295–324. [INFOTRIEVE], [CSA]
- Grayburn, P.A., Erickson, J.M., Escobar, J., Womack, L., Velasco, C.E. (1995). J. Am. Coll. Cardiol. 26: 1340–1347. [INFOTRIEVE], [CSA], [CROSSREF]
- Quay, S.C., Eisenfeld, A.J. (1997). Clin. Cardiol. 20(Suppl. I): I-19–I-26. [CSA]
- Robbin, M.L., Eisenfeld, A.J. (1998). Radiology 207: 717–722. [INFOTRIEVE], [CSA]
- Gong, Z., Giraud, G., Kenny, A., Sahn, D.J. (1994). Circulation 90: No., Part 2, abstract No. 2994. [CSA]
- Walker, K.W., Pantely, G.A., Sahn, D.J. (1997). Investigative Radiol. 32: 728–734. [CSA], [CROSSREF]