Abstract
Despite the tremendous progress in research on hemoglobin (Hb) cellular and molecular responses, the current understanding of Hb's overall intrinsic toxicity is still limited. The complete mechanism of Hb-induced vasoconstriction has not yet been established, particularly the involvement of the renin-angiotensin system (RAS). Some studies emphasized that Hb may augment the vascular responsiveness to angiotensin (Ang)-II. It was also reported that Hb, as well as Ang-II, influences the synthesis of 8-iso prostaglandin F2 alpha, which has an impact on renal flow and possibly RAS. Hb in the presence of H2O2 gains enzymatic activity. Thus, it is possible that Hb directly and/or indirectly can activate RAS. In this study, we monitored the effect of ferrous- and ferryl-Hb, and H2O2 alone, on conversion of Ang-I to its active metabolites. The structural and immunological identity of the resulting products were evaluated by reversed phase C-18 HPLC and ELISA, respectively. Additionally, ACE-like activity of Hbs was measured spectrophotometrically by determining their ability to react with the ACE substrate, the synthetic tripeptide N-[3-(2-furyl)acryloyl]-L-phenylalanylglycylglycine. Results indicate that while ferrous-Hb can serve as a receptor for Ang-I, its ferryl form possesses ACE-like activity, being able to convert, within minutes, Ang-I to Ang-II, Ang-III, Ang-IV, Ang (1-7) and other unresolved fragments. H2O2 itself had a very limited hydrolyzing effect on Ang-I. Based on this study, it can be concluded that ACE-like activity of Hb with rapid formation of active angiotensins may be a contributor to the still unexplained vasoconstrictive response observed immediately after Hb administration.
INTRODUCTION
Hb-induced vasoconstriction is an early event that occurs within minutes after infusion [Citation[1-5]]. However, currently proposed mechanisms to explain this phenomenon (i.e., NO scavenging, ionized calcium accumulation, eicosanoids, isoprostanes and endothelin production, protein kinase activation, and others) cannot justify such a quick response [Citation[1-3], Citation[6-12]]. The rapid rise in the peripheral vascular resistance and blood pressure are associated with bradycardia and decreased cardiac output [Citation[1], Citation[4], Citation[5]]. It is still unknown what triggers such a fast hemodynamic response and to what extent the rise in blood pressure affects the perfusion rate of the vital organs [Citation[13]].
Although Hb-mediated hemodynamic changes look similar to those observed with Ang-II, not too many researchers and clinicians realized that Hb and Ang-II share some vasoconstrictive properties [Citation[14]]. There are only a few reports describing Ang-II effects on Hb-mediated vasoconstriction. It was reported that the Hb moiety per se does augment the vascular responsiveness to Ang-II in the isolated perfused rat kidney [Citation[15]]. The intra-arterial injection of polyoxyethylene-conjugated Hb was found to cause a similar, but weaker response [Citation[16]]. However, the ability of captopril, an ACE inhibitor, to block the vasoconstrictor action of Ang-II in rabbit aortic rings was not reduced by recombinant Hb [Citation[17]]. Losartan, an Ang-II receptor antagonist, failed to reduce the bradycardia associated with the pressor effect of diaspirin cross-linked Hb [Citation[18]]. Yet, the effect of Hb on the entire RAS was never investigated.
In the literature there are also indirect indications that Hb could activate RAS. The inhibition of NO synthesis and superoxide generation, a feature of Hb, was found to be associated with the activation of vascular ACE [Citation[19]]. It was also reported that Hb significantly increases the production of 8-iso prostaglandin F2 alpha (8-isoprostane), a potent vasoconstrictor of renal arteries [Citation[7]]. In patients with intravascular hemolysis, free Hb correlates with increased urine 8-isoprostane and a decreased glomerular filtration rate (GFR) [Citation[20]]. 8-isoprostane is known to enhance Ang-II mediated vasoconstriction [Citation[21]]. Therefore, it is possible that free Hb, via 8-isoprostane's effect on renal flow, may activate RAS. It is also probable that the never explained mechanism of sodium reabsorption [Citation[22]] and myocardial necrosis [Citation[23]] seen after administration of Hb solution may have a link with RAS through the Ang-II → Ang-III → aldosterone pathway [Citation[14], Citation[24], Citation[25]].
Approximately 60% of angiotensin is present in the blood in its unconverted, inactive, Ang-I form [Citation[26]]. The blood of healthy individuals also contains H2O2 in a µM concentration [Citation[27]]. Therefore, it is theoretically possible that the infused Hb may rapidly react with the existing H2O2, and by gaining pseudo-peroxidase activity of ferryl-Hb may mediate the ACE-independent conversion of Ang-I, thus participating in early vasoconstrictive responses. Therefore, we tested the hypothesis that free Hb, alone or activated by H2O2 (ferryl-Hb), may influence RAS by gaining ACE-like activity, and may be able to participate in the non-ACE conversion of Ang-I to its active metabolites.
This research was designed to shed more light onto the mechanism(s) of hemodynamic disturbances observed immediately after Hb transfusion.
MATERIALS AND METHODS
All procedures in the evaluation of the pseudo-ACE activity of Hb and/or H2O2 were done in accordance with the guidelines of the TTUHSC Institutional Review Board for the Protection of Human Subjects (IRB Code No: 93189) and DHHS rules.
Preparation and Characterization of Hemoglobin Solutions
Ferrous Hemoglobin
Tetrameric Hb, obtained from bovine RBC, was prepared at the Texas Tech University Health Sciences Center Blood Substitute Good Laboratory Practice Pilot Plant (Lubbock, TX). All procedures were done under sterile, pyrogen free conditions, using U.S. Pharmacopoeia grade chemicals, water, and buffer solutions. The method for preparation of ferrous-Hb tetramer was previously published [Citation[8], Citation[9]].
Ferryl Hemoglobin
The oxidative challenge following the reaction of Hb with H2O2 was evaluated as described earlier [Citation[9], Citation[28], Citation[29]]. Absorption spectra at 560, 576, 577, and 630 nm were measured by a Coleman 575 spectrophotometer. The proportions of ferrous-Hb, ferric-Hb, and ferryl-Hb were calculated by multi-component analysis using earlier reported extinction coefficients [Citation[9]].
Assessment of Pseudo-ACE Activity of Hemoglobin
Effects of Hemoglobin on ACE Synthetic Substrate N-[3-(2-furyl)acryloyl]-L-phenylalanylglycylglycine (FAPGG)
The pseudo-ACE activity of Hb was measured spectrophotometrically by comparing the ability of ferrous- and ferryl-Hb to react with the ACE substrate FAPGG that mimics Ang-I. The reaction was based on the ability of ACE to hydrolyze FAPGG to furylacryloylphenylalanine (FAP) and glycoglycine, which was associated with a decrease in absorbance at 340 nm. In brief, a reaction volume (1 mL) containing 0.066 M phosphate buffer at pH 7.4 and 150 µM of FAPGG (Trinity Biotech USA, St. Louis, MO), was reacted with: 1) 15 µM of ferrous-Hb; 2) 150 µM of H2O2; or 3) 15 µM of ferrous-Hb activated with 150 µM of H2O2, and incubated for 15 min at 37°C. Saline served as the control. The 340 nm absorbance was obtained at the beginning and the end of incubation. After corrections for Hb, ACE activity in the sample was determined by comparing the sample reaction rate to that obtained with the ACE calibrator (81 µU/mL at 37°C; Trinity Biotech USA). In this method, 1 mU of ACE represents the production of 1 nM of FAP per min at 37°C.
Effects of Hemoglobin and Hydrogen Peroxide on the Conversion of Ang-I to Ang-II and Other Active Angiotensin Metabolites
The ability of ferrous- and ferryl-Hb and H2O2 to convert Ang-I was evaluated by the reversed phase C-18 HPLC (structural/spectral identity) and the ELISA (immunological compatibility).
Human Ang-I (Sigma Chemical, St. Louis, MO) in a concentration of 77 µM in 0.066 M phosphate buffer, pH 7.4, was incubated 15 min at 37°C with: 1) 7.7 µM of ferrous Hb (1:10 molar ratio with respect to Ang-I); 2) 77 µM of H2O2 (1:1 molar ration with respect to Ang-I); and 3) 7.7 µM of ferrous-Hb activated with 77 µM of H2O2 (1:2.5 molar ratio with respect to heme; ferryl-Hb). After incubation, the reaction volume was subjected for filtration (6,000 × g) using the Nanosep® centrifugal device with 10 K molecular weight cutoff (Pall Gelman Laboratory, Ann Arbor, MI). In the control, Hbs and H2O2 were replaced with saline. Additionally, to evaluate the effect of higher concentrations of H2O2 both alone and with Hb, the 77 µM of Ang-I was reacted with: 1) 216 µM of H2O2 (2.8:1 molar ratio with respect to Ang-I); and 2) 7.7 µM of ferrous Hb activated with 216 µM of H2O2 (1:7 molar ratio with respect to heme; ferryl-Hb). The reaction conditions were the same as above. The filtrate was subjected to reversed phase HPLC analysis using the DiscoveryTM C18 column (ID: 25 cm × 4.6 mm, particle size 5 µm; Supelco, Bellefonte, PA) and Waters 990 chromatography system equipped with photodiode array detector (Waters, Milford, MA). In brief, 20 µL of filtrate was injected and a chromatogram developed using a linear gradient with solvent A (5 mM NH4HCO3 in 5 mM KH2PO4, pH 7.4) and solvent B (50% Solvent A plus 50% Acetonitrile), from A/B 70%/30% to A/B 20%/80% in 25 min. Separation was completed in 30 min at a flow rate of 1.0 mL/min. In order to correlate retention time with different forms of angiotensin, calibration of the C-18 column was performed with human Ang-I, Ang-II, Ang-III and Ang (1–7) fragment (Sigma Chemical). The retention time and spectral characteristics (200–340 nm) were used to identify different forms of angiotensin. Other angiotensin metabolites, including Ang-IV, were identified on the basis of their spectral characteristics (200–340 nm). The oxidation products from the reaction between Hb and H2O2, smaller than 10 kDa, were monitored by their spectral characteristics and retention time.
The immunological compatibility of the resulting Ang-II was evaluated using a commercially available ELISA (SPIBIO, Massy Cedex, France). The reaction condition was identical to that in the C-18 HPLC study in which 7.7 µM Hb was reacted with 77 µM of H2O2. The immunological identification test excluded the experiments that used H2O2 in a concentration higher than 77 µM. In order to measure the Ang-II within the detection range offered by the ELISA kit, the samples were diluted with phosphate buffer, pH 7.4. Then, the samples were subjected for the reaction with a specific monoclonal anti-Ang-II Ab. The trapped molecules were covalently linked to the plate by glutaraldehyde via amino groups. After washing and denaturing treatment, Ang-II was exposed to the acetylcholinesterase-labeled mAb used as tracer. After incubation and washing, the reaction was developed with Ellman's reagent. The results in pg/mL were recalculated to µM concentration by applying the proper dilution factor. The results were also expressed as a percentage of conversion of Ang-I to Ang-II, considering 77 µM of Ang-I as 100%.
Assessment of Results
The levels of different factors measured in this study were analyzed using standard statistical methods. The numerous parameters were reported as an average of at least three separate sets of observations, run in duplicate. The HPLC results from the photodiode array detector of each LC separation were analyzed using various software: Waters 990 Integrator at 215 nm, Waters 990 Spectrum Index with Max Mode 205–340 nm, Waters 990 Peak Calculation at 215 nm, and Waters 990 Spectrum Analysis/Spectrum Calculation (Phoenix Technologies, Phoenix, AZ). Analysis of variance (ANOVA) was used to determine statistical differences among and between groups at the p < 0.001, p < 0.01, and p < 0.05 levels. Statistical analyses were performed using the StatView® 5 statistical package (SAS Institute, Inc., San Francisco, CA).
RESULTS
Characteristics of Hemoglobin Solutions
Tetrameric ferrous Hb had physical-biochemical characteristics similar to those previously reported [Citation[8], Citation[9], Citation[28-30]]. Specifically, the Hb solution was free of non-heme proteins and stromal phospholipids. The viscosity was 0.80–0.83 cP, and the oncotic activity was ca. 49 mm Hg. The oxygen affinity (P50 value) was 26 mm Hg in the presence of 100 mM of chloride. The spectrophotometric analysis of the ferric heme level revealed that the oxidation of iron was minimal (2.7%). The chromatographic profile illustrated that the Hb solution was uniformly composed of 68 kDa tetramers with a pI of 6.8–7.0, as previously reported [Citation[31]].
The reaction of ferrous-Hb with the 2.5 molar excess of hydrogen peroxide (with respect to heme) resulted in the formation of ferryl- and ferric-Hb species and a drop in ferrous-Hb content. The ferryl-Hb reached its maximum level at 5 min (70.3% of the total). After 15 min of incubation, ferryl-Hb accounted for 60% of all Hb species present in the reaction volume. Ferrous- and ferric-Hb levels were 21% and 19%, respectively. The reaction of ferrous-Hb with H2O2, in a molar ratio 1:7 with respect to heme, resulted in a more rapid heme oxidation. After 5 min of incubation almost 100% of Hb was converted to its ferryl form. The level of ferryl-Hb after 15 min of incubation accounted for almost 90% and ferric-Hb for 10%. Ferrous-Hb was not present in the reaction volume. These effects of different concentrations of H2O2 on ferrous-Hb (2.5:1 and 7:1 molar ratio with respect to heme) were identical to those described previously [Citation[9], Citation[28], Citation[32]]. The Hb solutions used in these experiments were isotonic and had a physiological pH.
Evaluation of ACE-like Activity of Hbs and Hydrogen Peroxide
The effect of Hbs and H2O2 on the ACE synthetic substrate that mimics Ang-I is presented in . The extent of FAPGG hydrolysis to FAP and glycoglycine that can be translated into the ACE activity was found to be dependent on the degree of heme oxidation. While H2O2 showed no effect on the ACE synthetic substrate, ferryl-Hb represented significant ACE-like activity corresponding with 27.80 ± 4.90 mU/mL of ACE. The ACE-like activity of ferrous-Hb after 15 min of incubation showed to be only slightly elevated (4.23 ± 2.70 mU/mL).
Table 1. Pseudo-ACE activity of Hbs toward N-[3-(2-furyl)acryloyl]-L-phenylalanylglycylglycine (FAPGG), the ACE synthetic substrate that mimics Ang-I
The chromatographic analysis of angiotensin by C-18 reversed phase HPLC is a well-accepted analytical method used in the identification of the various angiotensin metabolites. In this study, the characterization of the angiotensin metabolites was done on the basis of their retention time and spectral characteristics. With this chromatographic separation method the elution time for the standards: Ang-I, Ang-II, Ang-III and Ang (1–7) were approx. 23–24, 15–16, 16–17 and 8–9 min, respectively. The spectral analysis, which was within the 200–340 nm range, of the angiotensin standards revealed characteristic differences between their spectrums. The oxidation products from the reaction between Hb and H2O2 that were present in the 10 kDa filtrate were separated at 2–4 min retention time.
shows examples of C-18 HPLC elution profiles of Ang-I: untreated (A) and treated with H2O2 (B), ferrous-Hb (C) and ferryl-Hb (D). The elution pattern, spectral and integrator analysis of untreated Ang-I, eluted at approx. 24 min, revealed that 88% remained in its native form (A). In these samples Ang-I was associated with impurities that include: (1) a less polar fraction than Ang-I that was separated between 26 and 27 min (8.5%); and (2) a more polar fraction than Ang-I that was eluted at 21 min (3.5%). These impurities, which did not interfere with this study, were present in every Ang-I preparation purchased from Sigma Chemical.
Figure 1 C-18 reversed phase chromatographic (bottom) and spectral (top) profiles of different angiotensin metabolites produced following the reaction of 77 µM Ang-I with: saline-control (A), 77 µM of hydrogen peroxide (1 : 1 molar ratio with respect to Ang-I) (B), 7.7 µM of ferrous-Hb (1:10 molar ratio with respect to Ang-I) (C), and 7.7 µM of Hb reacted with 77 µM of hydrogen peroxide (1:2.5 molar ratio in respect to heme; ferryl-Hb) (D). Chromatographic Fraction Identifiers: 1 = Angiotensin I; 2 = Angiotensin II; 3 = Angiotensin III; 4 = Angiotensin IV; 5 = Angiotensin (1–7) Fragment; 6 = Other angiotensin metabolites; 7 = Heme byproducts; 8 = Oxidative products of the reaction Between Hb and hydrogen peroxide. The reaction conditions and C-18 separation method are described in the Materials and Methods section. The integrator analysis (area %) of the obtained fractions is presented in the Results section.
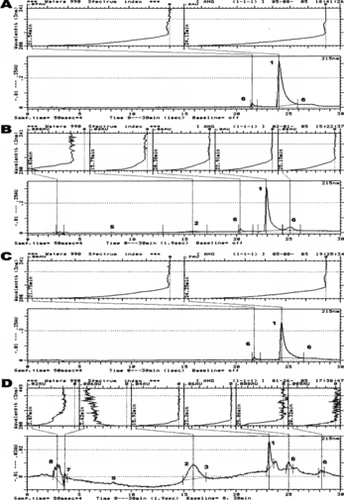
The chromatogram obtained following the reaction of Ang-I and H2O2 (molar ratio 1:1) shows that 77% of Ang-I (eluted at 23–24 min) is in its native form and approx. 5% of Ang-I was converted to Ang-II, which separated at 16 min retention time (B). After H2O2 treatment, the level of a less polar than Ang-I fraction (26 min) increased to 13.5% but the concentration of a more polar fraction (21 min) than Ang-I remained the same (3.5%). This study shows that H2O2 has an impact on Ang-I, being able to convert 6% of it into Ang-II.
The treatment of Ang-I with ferrous-Hb (molar ratio 10:1 with respect to Ang-I) resulted in a decrease in its concentration by about 18% (C). Ferrous-Hb alone failed to hydrolyze Ang-I. The observed scavenging effect of Hb toward Ang-I is perhaps related to the ability of the Hb molecule to serve as a receptor for many peptides [Citation[33], Citation[34]].
D shows that ferryl-Hb was able to hydrolyze Ang-I. A 15 min exposure of Ang-I to ferryl-Hb resulted in the formation of 35% of Ang-II/III, separated together at 15–17 min retention time, 1.8% of Ang-IV and 2% of the Ang (1–7) fragment that were eluted at 18 and 8 min, respectively. Ferryl-Hb significantly decreased the level of Ang-I (to 26%) and increased to 17% the formation of a less polar than Ang-I fraction, which was separated at 25–26 min. Ferryl-Hb was also able to produce a small amount of other unidentified angiotensin metabolites, which were eluted between 19–23 and 27–29 min. The spectral analysis confirmed that these fractions are indeed angiotensin metabolites (, top). The mixture of oxidized products formed after the incubation of ferrous-Hb (7.7 µM) and H2O2 (77 µM) eluted as an unresolved peak between 2.5 and 4 min. This experiment showed that ferryl-Hb indeed has pseudo-ACE-like activity, being able to nonspecifically hydrolyze Ang-I into Ang-II, Ang-III, Ang-IV, Ang (1–7) fragment and other unresolved angiotensin metabolites.
The immunological compatibility test revealed that while ferryl-Hb was capable of converting approx. 26% of Ang-I into Ang-II, hydrogen peroxide alone produced slightly over 5% of Ang-II (). This immunological test revealed that ferrous-Hb is unable to hydrolyze Ang-I. In the ferrous-Hb group, the observed 2.4% increase in the immunoreactive Ang-II fraction was related to some (approx. 3%) nonspecific cross-reactivity of monoclonal Ab, used in this assay, with Ang-I. The 9% difference between the ferryl-Hb rate of conversion of Ang-I into Ang-II measured with C-18 analysis (35%) and ELISA (26%) () suggests that the angiotensin fraction separated at 15–17 min contains a mixture of Ang-II and Ang-III (). This test illustrates that the pseudo-ACE-like activity of ferryl-Hb results in the synthesis of immunologically active angiotensin metabolites.
Table 2. Effects of Hbs and/or hydrogen peroxide on the conversion rate of Ang-I into Ang-II, determined on the basis of the immunological identification of Ang II with the ELISA method
A shows that 216 µM of H2O2 (2.8:1 molar ratio with respect to Ang-I) had a more detrimental effect on Ang-I than its lower concentration of 77 µM (B). The chromatographic analysis revealed that a 21% decrease in Ang-I concentration (elution time 24 min), as compared to the 77 µM group, was associated with a significant increase in Ang-II/III level (7%, 15–18 min), elevation of a less polar fraction than Ang-I (27%, 26 min) and the formation of a new unresolved Ang-I fraction (3.5%) separated at 23.5 min. The concentration of a more polar Ang-I fraction, separated at 22 min remains unchanged. The ferryl-Hb, produced by reacting ferrous-Hb with a 7 molar excess of hydrogen peroxide (with respect to heme), showed to be a more potent activator of Ang-I hydrolysis than its version synthesized with 2.5 molar excess (B). A dramatic decrease in the level of Ang-I (to 18%, 24 min) was associated with a significant increase of Ang-II (29%, 16 min), Ang-III (37%, 16.5 min) and Ang-IV (3.5%, 18.5 min). This treatment also resulted in the formation of the Ang (1–7) fragment (5%, 8 min) and other unresolved Ang-I and Ang-II metabolites separated at 22 and 25.6 min. The oxidative products formed during the incubation of ferrous-Hb (7.7 µM) with H2O2 (216 µM) were separated at 1.3–3.5 min retention time. These experiments provide additional evidence that the pseudo-ACE-like activity of Hb is driven by H2O2 concentration.
Figure 2 C-18 reversed phase chromatographic (bottom) and spectral (top) profile of different angiotensin metabolites produced following the reaction of 77 µM Ang-I with: 216 µM hydrogen peroxide (1:2.8 molar ratio with respect to Ang-I) (A) and 7.7 µM Hb reacted with 216 µM of hydrogen peroxide (1:7 molar ratio with respect to heme; ferryl Hb) (B). Chromatographic identifiers are the same as in .
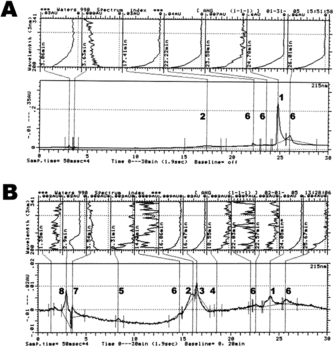
DISCUSSION
Several novel findings emerged from this study. First, ferryl-Hb has ACE-like activity and is able to convert, within minutes, Ang-I into Ang-II, Ang-III, Ang-IV, Ang (1–7) fragment and other yet unresolved angiotensin metabolites, and the extent of this event depends on H2O2/Hb molar ratio. This phenomenon might be a contributor to the vasoconstriction seen immediately after Hb infusion. And second, H2O2 in µM concentrations has a small but evident hydrolyzing effect on Ang-I, which could provide more insight into its recently reported vasoconstrictor potency [Citation[35]].
The blood and endothelial cells are first in line of exposure to free Hb. Although the effects of Hb on blood cellular components (RBC, platelets, WBC) and endothelial cells have become a central focus of research [Citation[7], Citation[32], Citation[36]], its effects on plasma still remain undetermined. In the literature there is limited information on the interaction of free Hb with the components of plasma. The only research in this area is related to the evaluation of the interaction of Hb with haptoglobin and its effects on plasma NO, complement, coagulation factors, LDL, GSH, endotoxin and drugs [Citation[3], Citation[7], Citation[28], Citation[29], Citation[32], Citation[33], Citation[37-41]].
The active species formed after the reaction of Hb with peroxides can contribute to many pathological events seen after Hb administration [Citation[6], Citation[7], Citation[28], Citation[29], Citation[32], Citation[42]]. It is well recognized that the reaction between Hb and H2O2 generates a series of steps that involves cycling of Hb between ferric- and ferryl-forms, and it is now believed that the conversion of the ferryl- to ferric-form is one of the most important steps in diminishing Hb pseudo-peroxidase activity [Citation[43]]. It was reported that this chemical conversion is H2O2 concentration dependent. In fact, after removal of residual H2O2 from the reaction mixture, the ferryl-Hb is shown to be a long living species, not converted to the ferric-form [Citation[44]]. Since the level of H2O2 in normal human plasma is in the µM range [Citation[27]], free Hb in µM to mM concentrations, similar to those seen during intravascular hemolysis or injection of Hb-based oxygen carriers (HBOC), can be associated with the formation of a long lived ferryl species. The pseudo-peroxidase activity of ferryl-Hb will solely rely on the Hb/H2O2 molar ratio in plasma. Some studies suggest that the level of H2O2 in human plasma is between 4 and 35 µM, although in the inflammatory condition could reach 100 µM [Citation[27]]. Our earlier study showed that the H2O2 plasma level is approx. 30 µM and can increase up to 75 µM, 1 hr after Hb administration [Citation[30]]. It is known that H2O2 alone, below 50 µM, has limited cytotoxicity to many cells; however, it can function as an inter- and intra-cellular signaling molecule [Citation[27]]. 10 µM of endogenous H2O2 acts as a vasoconstrictor in resistance vessels and contributes to the regulation of blood pressure [Citation[35]].
Our present study provides the evidence that H2O2 at a molar ratio of 1:1 with respect to Ang-I is able to convert at least 5% of Ang-I into Ang-II (B). H2O2 at this level also increases the formation of less polar Ang-I metabolites. The higher concentration of H2O2 (1:2.8 with respect to Ang-I) was associated with a significant increase (approx. 7%) in Ang-II/III, Ang-IV (3.5%) and other angiotensin metabolites (A). Perhaps this effect of H2O2 on Ang-II production can help explain its vasomotor effect and contribution in the development of oxidative stress-mediated hypertension [Citation[35]]. More recent studies suggest that the combination of Ang-II and H2O2 dose dependently increases endothelial apoptosis and 8-isoprostane formation, evidencing the role of RAS in oxidative stress-induced endothelial damage [Citation[45]].
This current work, which is in full agreement with our earlier studies [Citation[9], Citation[28], Citation[32]], illustrates that free Hb, when reacted with a 2.5- and 7-fold excess of H2O2 (with respect to heme), can easily be converted into a relatively long living ferryl species. The most potent ferryl-Hb was detected after the reaction of Hb with the higher concentration of H2O2. After 5 min of incubation, approx. 70% of ferrous-Hb was converted into ferryl species when reacted with a 2.5 molar excess of H2O2 and almost 100% with a ratio of 1:7 (data shown in the Results Section). The rapid formation of ferryl-Hb suggests its possible immediate impact on biological systems. Our earlier work showed that the Fenton and Haber-Weiss reactions can no longer be used to explain the mechanism of oxidative damage induced by Hb and provided evidence that ferryl-Hb indeed is responsible for these effects [Citation[28]]. The consequences of ferryl-Hb in blood are immense, from the devastating, oxidizing effect on the blood cellular components to the oxidative modification of plasma lipoproteins [Citation[6], Citation[7], Citation[9], Citation[28], Citation[29], Citation[32], Citation[42-44], Citation[46]].
Human plasma contains many metabolic, hormonal, neuronal, and cellular (from PMN and endothelial cells) factors that are prone to the oxidative reactions. While in the literature there is only one report, that the pseudo-peroxidatic activity of ferryl-Mb is responsible for the primary oxidation of adrenaline [Citation[47]], some experts are warning that the higher oxidation state of heme proteins, particularly Hb, can be a potent oxidant capable of promoting oxidative damage to most classes of biological molecules [Citation[42]]. Nonetheless, this emerging call did not accelerate enough interest in further investigation of ferryl-Hb effects on the blood plasma active factors, particularly the NO-independent mediators of the vascular tone.
Despite the large number of articles published, the current understanding of how Hb mediates vasoconstriction is not well defined [Citation[7]]. There is still no mechanism provided for rapid, within a few minutes, and sustained, for a few hours, Hb-mediated vasoconstrictor response [Citation[1], Citation[4], Citation[5]]. The pressor response to Hb is much faster [Citation[3]] than the time required for its extravasation into the subendothelial space and scavenging of NO [Citation[48]]. Even more time is needed for Hb-related activation of phospholipase C and 1,4,5-triphosphate, required to raise the intracellular ionized calcium [Citation[7], Citation[8]]. While the eicosanoids' role in Hb-induced hemodynamic disturbances has not been substantiated, isoprostane synthesis requires Hb and lipid redox cycling, which could not occur rapidly [Citation[7]]. The controversial role of Hb in endothelin-1 formation is still under investigation [Citation[9-11]]. The Hb-activated protein kinases, which are known to be contributory factors in the non-endothelium mediated vessel contraction, require lengthy signaling, transcriptional and translational changes [Citation[12]]. Therefore, there is still controversy regarding the exact mechanism by which Hb causes vasoconstriction, particularly its early phase.
The rapid elevation of blood pressure in response to Hb is always associated with increased peripheral vascular resistance, decreased cardiac output and bradycardia [Citation[1], Citation[4], Citation[5]]. These hemodynamic events are similar to those mediated by Ang II [Citation[14]]. Even though it was reported that Hb augments the vascular responsiveness to Ang-II [Citation[15]], and pyridoxylated polyoxyethylene conjugated Hb has a similar effect [Citation[16]], the full spectrum of Hb's impact on RAS was never fully investigated.
RAS is involved in the homeostasis of peripheral vascular resistance and the electrolyte composition of body fluids [Citation[14]]. Any decrease in GFR is associated with the release of renin, which acts on angiotensinogen to produce Ang-I [Citation[14]]. In blood of healthy subjects, only 40% of Ang-I is converted into Ang-II [Citation[26]]. In the human renal vascular bed, less than 10% of arterially delivered Ang-I is converted [Citation[49]]. Therefore, a large pool of inactive Ang-I is always present in the bloodstream awaiting conversion into its biologically active form, Ang-II. The angiotensin degradation products possess different biological activities. Their vasoconstriction potencies vary. Although Ang-III is 16 times less active than Ang-II and Ang-IV is approx. 2,700-fold less potent, Ang (1–7) peptide has a vasodilatory effect [Citation[50]]. Ang-II is the most potent vasoconstrictor and stimulator (along with Ang-III/IV) of aldosterone synthesis and release, with consequent sodium and water retention by the kidney [Citation[14], Citation[50-53]]. Ang-II stimulates NAD(P)H oxidase to produce the reactive oxygen species, which mediate oxidative and inflammatory responses of endothelium, causing vascular tissue injury [Citation[14], Citation[50-53]]. Recently, it was reported that Ang-II is able to activate NF-kappa B and induce IL-6, which express circulating acute phase reactants, such as CRP and angiotensinogen, the Ang-I precursor [Citation[54], Citation[55]]. In addition, Ang-II and Ang-IV may stimulate the production of plasminogen activator inhibitor-1 and cause thromboembolic events [Citation[56]]. It also was reported that aldosterone, which can be activated particularly by Ang-II and Ang-III, has a number of deleterious effects on the cardiovascular system, including myocardial necrosis [Citation[25]], similar to that observed after HBOC administration [Citation[23]].
In the present study, we demonstrated that ferrous-Hb without pseudo-peroxidase activity failed to hydrolyze Ang-I (, C, and ). This Hb, however, showed to be a receptor for Ang-I (C). Approximately 18% of Ang-I was incorporated into the Hb molecule. It is known that Hb can interact with many drugs and peptides, and even strong anion-exchange chromatography is unable to separate such complexes [Citation[34]]. Drugs and peptides seek out niches in the Hb molecule, where their stereochemistry of binding is determined by the available van der Waals space, and, within that space, by a tendency to maximize electrostatic interaction, with binding range from strong hydrogen bonds to weakly polar interactions [Citation[33]]. Therefore, ferrous-Hb can be considered as an Ang-I transporting system, however, its biologic/pharmacologic significance needs to be determined.
Yet, Hb with pseudo-peroxidase activity rapidly hydrolyzed Ang-I into its active metabolites (, D, , B). Ang-I, reacted for 15 min with ferryl-Hb, produced by the reaction of ferrous-Hb with a 2.5 molar excess of H2O2, was converted into 35% Ang-II/III, 1.8% Ang-IV and 2% Ang (1–7). Ferryl-Hb was also able to produce other unresolved angiotensin metabolites (D). The resulting Ang-II showed a high degree of immunological compatibility with human Ang-II (). The ferryl-Hb, produced by the reaction of ferrous-Hb with a 7 molar excess of H2O2, more rapidly and effectively converted Ang-I into its active metabolites (B). After 15 min of incubation Ang-I was converted into: 29% Ang-II, 37% Ang-III, 3.5% Ang-IV, 5% Ang (1–7) fragment, and other unidentified angiotensin metabolites.
The ability of ferryl-Hb to rapidly convert Ang-I into its active fragments, suggests that the formation in the bloodstream of Hb with pseudo-peroxidase activity might disrupt the vascular tone homeostasis and produce other pathological effects mediated by angiotensins. It seems highly probable that infused Hb after the reaction with H2O2, already existing in the bloodstream in µM concentrations [Citation[27], Citation[30]], and by gaining ACE-like activity to convert Ang-I into its active forms could participate in the early vasoconstrictive events observed immediately after Hb infusion.
In this study we did not focus on the pharmacological consequences of Hb-mediated synthesis of active angiotensin metabolites. We can assume, however, that if such a phenomenon occurs, we should expect an array of pathological events. Particularly, the formation of Ang-II and Ang-III, which are linked to aldosterone synthesis, may have further consequences, including myocardial necrosis [Citation[25], Citation[57]]. This effect is accelerated by removal of NO [Citation[57]]. Since Hb has well documented NO scavenging potency [Citation[3], Citation[6]] and is able to activate RAS, it is probable that the myocardial lesions observed after HBOC injection [Citation[23]] might be aldosterone-mediated. Besides our study, there is other indirect proof of the connection between Hb and aldosterone synthesis through the reported increased sodium reabsorption after HBOC administration [Citation[22]].
Although many aspects of the Hb-mediated synthesis of active angiotensin metabolites still await full elucidation, our present study suggests that the pseudo-ACE activity of Hb could be a significant contributor of the vasoconstrictive events seen within minutes after Hb administration.
In the literature there is some information about non-ACE conversion of Ang-I. Human neutrophil cathepsin G, human skin mast cell chymase and tonin from salivary gland can rapidly convert Ang-I to Ang-II [Citation[58], Citation[59]]. Multiple lines of evidence indicate that a dual enzymatic pathway exists in human arteries, mediated by ACE and serine proteases, one of which is chymase [Citation[60]]. This is the reason why ACE inhibitors incompletely block the production of Ang-II from Ang-I [Citation[61]]. Hence, another alternative Ang-II forming pathway, also ACE-independent, identified in our study carries out a reaction of physiologic importance. The pseudo-peroxidase potential of Hb provides a unique pathway for the generation of Ang-II and other angiotensin metabolites and may be of great importance for HBOC developers.
Our research documented that ferryl-Hb is capable of synthesizing many active angiotensin metabolites, therefore the “single bullet” strategy with ACE inhibitor or Ang-II receptor blocker, proposed earlier to counteract the impact of Hb on Ang-II, cannot be effective [Citation[17], Citation[18]]. After the realization that Hb's effect can be multifactorial, Texas Tech scientists are in the process of developing a comprehensive management strategy to counteract these unwanted RAS-mediated responses.
In conclusion, Hb activates RAS. While ferrous-Hb can be considered as a receptor for Ang-I, ferryl-Hb possesses ACE-like activity. Ferryl-Hb, within minutes, was able to convert Ang-I to Ang-II and other active angiotensin metabolites. This event, which is H2O2 concentration dependent, may be a contributor to the vasoconstriction observed immediately after Hb administration. Based on this study, we postulated that the pseudo-ACE activity of Hb could be a factor in the not yet fully understood Hb-mediated vasoconstriction.
REFERENCES
- Winslow, R.M. (1994). Vasoconstriction and the efficacy of hemoglobin-based blood substitutes. Transf. Clin. Biol. 1(1): 9–14.
- Kim, H.W., Greenburg, A.G. (1995). Hemoglobin mediated vasoactivity in isolated vascular rings. Art. Cells, Blood Substitutes, Immobilization Biotechnol. 23(3): 303–309.
- Kim, H.W., Greenburg, A.G. (1997). Ferrous hemoglobin scavenging of endothelium derived nitric oxide is a principle mechanism for hemoglobin mediated vasoactivities in isolated thoracic aorta. Art. Cells, Blood Substitutes, Immobilization Biotechnol. 25(1–2): 121–133.
- Kasper, S.M., Walter, M., Grune, F., Bischoff, A., Erasmi, H., Buzello, W. (1996). Effects of a hemoglobin-based oxygen carrier (HBOC-201) on hemodynamics and oxygen transport in patients undergoing preoperative hemodilution for elective abdominal aortic surgery. Anesth. Analg. 83: 921–927.
- Schubert, A., O'Hara, J.F., Przybelski, R.J., Tetzlaff, J.E., Marks, K.E., Mascha, E., Novick, A.C. (2002). Effect of diaspirin (DCLHb HemAssistTM) during high blood loss surgery on selected indices of organ function. Art. Cells, Blood Substitutes, Immobilization Biotechnol. 30(4): 259–283.
- Alayash, A.I. (2004). Oxygen therapeutics: Can we tame haemoglobin? Nat. Rev. Drug Discov. 3(2): 152–159.
- Simoni, J. (2005). Endothelial cell response to hemoglobin based oxygen carriers. Is the attenuation of pathological reaction possible?, in Artificial Oxygen Carrier. Its Front Line, K. Kobayashi, E. Tuschida, H. Horinouchi, Eds., Springer-Verlag: Tokyo, pp. 75–126.
- Simoni, J., Simoni, G., Martinez-Zaguilan, R., Wesson, D.E., Lox, C.D., Prien, S.D., Kumar, R.V. (1998). Improved blood substitute: Evaluation of its effects on human endothelial cells. ASAIO J. 454(5): M356–M367.
- Simoni, J., Simoni, G., Lox, C.D., Prien, S.D., Shires, G.T. (1995). Evidence for the direct inhibition of endothelin-1 secretion by hemoglobin in human endothelial cells. ASAIO J. 41(3): M641–M651.
- Gulati, A., Sharma, A.C., Singh, G. (1996). Role of endothelin in the cardiovascular effects of diaspirin crosslinked and stroma reduced hemoglobin. Crit. Care Med. 24(1): 137–147.
- Tai, J., Kim, H.W., Greenburg, A.G. (1997). Endothelin-1 is not involved in hemoglobin associated vasoactivities. Art. Cells, Blood Substitutes, Immobilization Biotechnol. 25(1–2): 135–140.
- Wickman, G., Lan, C., Vollrath, B. (2003). Functional roles of the rho/rho kinase pathway and protein kinase C in the regulation of cerebrovascular constriction mediated by hemoglobin: relevance to subarachnoid hemorrhage and vasospasm. Circ. Res. 92(7): 809–816.
- Cheung, A.T., Driessen, B., Jahr, J.S., Duong, P.L., Ramanujam, S., Chen, P.C., Gunther, R.A. (2004). Blood substitute resuscitation as a treatment modality for moderate hypovolemia. Art. Cells, Blood Substitutes, Immobilization Biotechnol. 32(2): 189–207.
- Peach, M.J. (1977). Renin-Angiotensin system: Biochemistry and mechanism of action. Physiol. Rev. 57(2): 313–370.
- Nishi, K., Ueda, S., Sato, D., Nishi, K. (1994). Effects of pyridoxylated hemoglobin polyoxyethylene conjugate and stroma-free hemoglobin on renal vascular responsiveness to vasoactive substances in isolated perfused rat kidney. Artif. Organs. 18(6): 429–438.
- Kida, Y., Iwata, S., Gyoutoku, Y., Aikou, A., Yamakawa, T., Nishi, K. (1991). Vascular responsiveness to various vasoactive substances after exchange transfusion with pyridoxalated hemoglobin polyoxyethylene conjugate (PHP) solution in anesthetized rats. Artif. Organs. 15(1): 5–14.
- Rioux, F., Drapeau, G., Marceau, F. (1995). Recombinant human hemoglobin (rHb1.1) selectively inhibits vasorelaxation elicited by nitric oxide donors in rabbit isolated aortic rings. J. Cardiovasc. Pharmacol. 25(4): 587–594.
- Moisan, S., Drapeau, G., Burhop, K.E., Rioux, F. (1998). Mechanism of the acute pressor effect and bradycardia elicited by diaspirin crosslinked hemoglobin in anesthetized rats. Can. J. Physiol. Pharmacol. 76(4): 434–442.
- Usui, M., Egashira, K., Kitamato, S., Koyanagi, M., Katoh, M., Kataoka, C., Shimokawa, H., Takeshita, A. (1999). Pathogenic role of oxidative stress in vascular angiotensin-converting enzyme activation in long-term blockade of nitric oxide synthesis in rats. Hypertension 34(4 Pt 1): 546–551.
- Simoni, J., Simoni, G., Griswold, J.A., Moeller, J.F., Tsikouris, Khanna, A., Roongsritong, C., Wesson, D.E. (2005). Free hemoglobin (Hb)-mediated synthesis of 8-iso prostaglandin F2 alpha and its effects on coronary artery endothelium in chronic renal failure (CRF). ASAIO J. 51(2): 62A ( Abstract).
- Badr, K.F., Abi-Antoun, T.E. (2005). Isoprostanes and the kidney. Antiox. Redox Signal. 7(1–2): 236–243.
- Baines, A.D., Christoff, B., Wicks, D., Wiffen, D., Pliura, D. (1995). Cross-linked hemoglobin increases fractional reabsorption and GFR in hypoxic isolated perfused rat kidneys. Am. J. Physiol. 269(5 Pt 2): F628–F636.
- Burchop, K., Gordon, D., Estep, T. (2004). Review of hemoglobin-induced myocardial lesion. Art. Cells, Blood Substitutes, Immobilization Biotechnol. 32(3): 353–374.
- Struthers, A.D., MacDonald, T.M. (2004). Review of aldosterone- and angiotensin II-induced target organ damage and prevention. Cardiovasc. Res. 61(4): 663–670.
- Stier, C.T., Chander, P.N., Rocha, R. (2002). Aldosterone as a mediator in cardiovascular injury. Cardiol. Rev. 10(2): 97–107.
- Campbell, D.J., Kladis, A. (1990). Simultaneous radioimmunoassay of six angiotensin peptides in arterial and venous plasma of man. J. Hypertens. 8(2): 165–172.
- Halliwell, B., Clement, M.V., Long, L.H. (2000). Hydrogen peroxide in the human body. FEBS Lett. 466(1): 10–13.
- Simoni, J., Simoni, G., Lox, C.D., Feola, M. (1994). Reaction of human endothelial cells to bovine hemoglobin solutions and tumor necrosis factor. Art. Cells, Blood Substitutes, Immobilization Biotechnol. 22(3): 777–787.
- Simoni, J., Simoni, G., Lox, C.D., Prien, S.D., Shires, G.T. (1997). Modified hemoglobin solution, with desired pharmacological properties does not activate nuclear transcriptional factor NF-kappa B in human vascular endothelial cells. Art. Cells, Blood Substitutes, Immobilization Biotechnol. 25(1–2): 193–210.
- Simoni, J., Feola, M., Canizaro, P.C. (1990). Generation of free oxygen radicals and the toxicity of hemoglobin solutions. Biomat. Art. Cells, Art. Org. 18(2): 189–202.
- Simoni, J., Simoni, G., Feola, M. (1993). Chromatographic analysis of biopolymers distribution in poly-hemoglobin, and intermolecularly crosslinked hemoglobin solution. Analyt. Chim. Acta. 279: 73–88.
- Simoni, J., Simoni, G., Wesson, D.E., Griswold, J.A., Feola, M. (2000). A novel hemoglobin-adenosine-glutathione based blood substitute: Evaluation of its effects on human blood ex vivo. ASAIO J. 46: 679–692.
- Perutz, M.F., Fermi, G., Abraham, D.J., Poyart, C., Burseaux, E. (1986). Hemoglobin as a receptor of drugs and peptides: X-ray studies of the stereochemistry of binding. J. Am. Chem. Soc. 108: 1064–1078.
- Simoni, J., Simoni, G., Feola, M., Canizaro, P.C. (1991). Evaluation of anion-exchange liquid chromatography for purification of hemoglobin from peptides and other proteins. Analyt. Chim. Acta. 249: 169–183.
- Suvorava, T., Lauer, N., Kumpf, S., Jacob, R., Meyer, W., Kojda, G. (2005). Endogenous vascular hydrogen peroxide regulates arteriolar tension in vivo. Circulation 112: 2487–2495.
- Simoni, J., Simoni, G., Lox, C.D., McGunegle, D.E., Feola, M. (1994). Cytokines and PAF release from human monocytes and macrophages: Effects of hemoglobin and contaminants. Art. Cells, Blood Substitutes, Immobilization Biotechnol. 22(3): 525–534.
- Feola, M., Simoni, J., Dobke, M., Canizaro, P.C. (1988). Complement activation and the toxicity of stroma-free hemoglobin solutions in primates. Circulatory Shock 25: 275–290.
- Tejedor, C., Lorente, J.A., Delgado, M.A., Fernandez-Segoviano, P., Se Paula, M., Tobalina, R., Alonso, M., Moscoso, A., Soto, F., Blazquez, J., Esteban, A. (2002). Interaction between hemoglobin and glutathione in the regulation of blood flow in normal and septic pigs. Crit. Care Med. 30(11): 2493–2500.
- Mandal, R., Teixeira, C., Li, X.F. (2003). Studies of cisplatin and hemoglobin interactions using nanospray mass spectrometry and liquid chromatography with inductively-coupled plasma mass spectrometry. Analyst 128(6): 629–634.
- Kaca, W., Roth, R.I., Levin, J. (1994). Hemoglobin, a newly recognized lipopolysaccharide (LPS)-binding protein that enhances LPS biological activity. J. Biol. Chem. 269(40): 25078–25084.
- Kim, H.W., Awad, M., Greenburg, A.G. (1994). Coagulation responses of human plasma after hemodilution with hemoglobin solution in vitro. Artif. Cells, Blood Substitutes, Immobilization Biotechnol. 22(3): 613–618.
- Alayash, A.I. (2001). Oxidative mechanisms of hemoglobin-based blood substitutes. Art. Cells, Blood Substitutes, Immobilization Biotechnol. 29(6): 415–425.
- Giulivi, C., Davies, K.J. (1990). A novel antioxidant role for hemoglobin. The comproportionation of ferrylhemoglobin with oxyhemoglobin. J. Biol. Chem. 265(32): 19453–19460.
- Goldman, D.W., Breyer, R.J., Yeh, D., Brockner-Ryan, B.A., Alayash, A.I. (1998). Acellular hemoglobin-mediated oxidative stress toward endothelium: A role for ferryl iron. Am. J. Physiol. 275(3 Pt 2): H1046–H1053.
- Akishita, M., Nagai, K., Xi, H., You, W., Sudoh, N., Watanabe, T., Ohara-Imaizumi, M., Nagamatsu, S., Kozaki, K., Horiuchi, M., Toba, K. (2005). Renin-angiotensin system modulates oxidative stress-induced endothelial cell apoptosis in rats. Hypertension 45(6): 1188–1193.
- Zilletti, L., Ciuffi, M., Franchi-Micheli, S., Fusi, F., Gentilini, G., Moneti, G., Valoti, M., Sgaragli, G.P. (1994). Cyclooxygenase activity of hemoglobin. Methods Enzymol. 231: 562–573.
- Giulivi, C., Cadenas, E. (1998). Oxidation of adrenaline by ferrylmyoglobin. Free Radic. Biol. Med. 25(2): 175–183.
- Smani, Y., Faivre, B., Audonnet-Blaise, S., Labrude, P., Vigneron, C. (2005). Relationship between arterial pressure evolution and free hemoglobin distribution into vascular wall in guinea pigs. J. Hypertens. 23(8): A8.
- Danser, A.H., Admiraal, P.J., Derkx, F.H., Schalekamp, M.A. (1998). Angiotensin I-to-II conversion in the human renal vascular bed. J. Hypertens. 16(12 Pt 2): 2051–2056.
- Li, Q., Feenstra, M., Pfaffendorf, M., Eijsman, L., van Zwieten, P.A. (1997). Comparative vasoconstrictor effects of angiotensin II, III, and IV in human isolated saphenous vein. J. Cardiovasc. Pharmacol. 29(4): 451–456.
- Ardaillou, R. (1997). Active fragments of angiotensin II: Enzymatic pathways of synthesis and biological effects. Curr. Opin. Nephrol. Hypertens. 6(1): 28–34.
- Ardaillou, R., Chansel, D. (1996). Angiotensin IV a new component of the renin-angiotensin system, which acts on kidney cells. Bull. Acad. Natl. Med. 180(2): 475–486.
- Chappell, M.C., Pirro, N.T., Sykes, A., Ferrario, C.M. (1998). Metabolism of angiotensin-(1–7) by angiotensin-converting enzyme. Hypertension 31(1 Pt 2): 362–367.
- Pueyo, M.E., Gonzalez, W., Nicoletti, A., Savoie, F., Arnal, J.-F., Michel, J.-B. (2000). Angiotensin II stimulates endothelial vascular cell adhesion molecule-1 via nuclear factor-kappa B activation induced by intracellular oxidative stress. Arterioscler. Thromb. Vasc. Biol. 20: 645–651.
- Brasier, A.R., Recinos, A., Eledrisi, M.S. (2002). Vascular inflammation and the renin-angiotensin system. Arterioscler. Thromb. Vasc. Biol. 22: 1257–1266.
- Ridker, P.M., Gauboury, C.L., Conlin, P.R., Seely, E.W., Williams, G.H., Vaughan, D.E. (1993). Stimulation of plasminogen activator inhibitor in vivo by infusion of angiotensin II. Evidence of a potential interaction between the renin-angiotensin system and fibrinolytic function. Circulation 87(6): 1969–1973.
- Rocha, R., Stier, C.T., Kifor, I., Ochoa-Maya, M.R., Rennke, H.G., Williams, G.H., Adler, G.K. (2000). Aldosterone: A mediator of myocardial necrosis and renal arteriopathy. Endocrinology 141(10): 3871–3878.
- Reilly, C.F., Tewksbury, D.A., Schechter, N.M., Travis, J. (1982). Rapid conversion of angiotensin I to angiotensin II by neutrophil and mast cell proteinases. J. Biol. Chem. 257(15): 8619–8622.
- Wintroub, B.U., Schechter, N.B., Lazarus, G.S., Kaempfer, C.E., Schwartz, L.B. (1984). Angiotensin I conversion by human and rat chymotryptic proteinases. J. Invest. Dermatol. 83(5): 336–339.
- Tom, B., Garrelds, I.M., Scalbert, E., Stegmann, A.P., Boomsma, F., Saxena, P.R., Danser, A.H. (2003). ACE- versus chymase-dependent angiotensin II generation in human coronary arteries. A matter of efficiency? Arterioscler. Thromb. Vasc. Biol. 23: 251–256.
- Richard, V., Hurel-Merle, S., Scalbert, E., Ferry, G., Lallemand, F., Bessou, J.P., Thuillez, C. (2001). Functional evidence for a role of vascular chymase in the production of angiotensin II in isolated human arteries. Circulation 104(7): 750–752.