Abstract
We studied the peroxidase activity of ferrylhemoglobin radical (Hb(Fe4+ = O*)) generated by the reaction of metHb (Hb(Fe3+)) with hydrogen peroxide (H2O2). To clarify the behaviors of ferrylHb radical, it was isolated from the reaction mixture of metHb and H2O2 by GPC at 4°C. The radical species underwent rapid autoreduction to metHb at 37°C accompanied with denaturation; however, it was stable for several minutes at 4°C. In ESR measurements, the signal of the ferrylHb radical immediately disappeared in the presence of l-Tyrosine (l-Tyr), and simultaneously, the signal of the ferric heme increased. This suggested that the ferrylHb radical immediately converted to metHb by l-Tyr even at 4°C. Furthermore, dimerized l-Tyr was detected in the reaction mixture. This showed that the ferrylHb radical was reduced to metHb by electron donation from l-Tyr. The enzymatic reaction using l-Tyr as the substrate resulted in the elimination of H2O2 in this system.
INTRODUCTION
Heme-containing proteins show high redox activities in the presence of reactive oxygen species (ROS). The reaction mechanism has been studied by many investigators invarious fields. It is well known that methemoglobin (metHb, Hb(Fe3+)), which has a ferric heme iron, is oxidized to ferrylHb radical (Hb(Fe4+ = O*)) by reaction with hydrogen peroxide (H2O2). Malencik et al. reported that horseradish peroxidase (HRP), which is also a kind of heme-protein, produced an L-Tyr dimer (diTyr) by coupling the ortho-positions of two L-Tyr molecules as substrate utilizing H2O2 [Citation[1]]. This reaction was based on the generation of the ferryl radical of HRP (HRP(Fe4+ = O*) (Compound I) by the oxidation of the ferric state (HRP(Fe3+)) by H2O2 followed by reduction of the radical state to regenerate the ferric state by the oxidative coupling of L-Tyr to diTyr. This is the enzymatic cycle of HRP.
The ferryl radical states (Compound I) of Hb and myoglobin (Mb), which are mainly induced by the reaction with H2O2, are known to be strong oxidants for several biomolecules such as tocopherol (and its analog Trolox C) [Citation[2], Citation[3]] and ascorbic acid [Citation[4], Citation[5]], and are reduced to the respective ferric states (metHb and metMb) by the acceptance of two electrons from these biomolecules. DiTyr and its related products were detected in human erythrocytes and were thought to be produced by the proteolysis of the ferrylHb radical generated by H2O2 [Citation[6]]. Thus, the ferryl radicals of Hb or Mb are unstable due to their high reactivity. Nagababu et al. reported a reaction intermediate generated from the oxoferryl state of Hb (Hb(Fe4+ = O)) in the process of heme degradation and the proteolysis of Hb by H2O2, and named it “rhombic heme” [Citation[7]].
We have developed Hb vesicles that encapsulate highly concentrated and purified Hb solution (Hb purification; > 99.9%, concentration; 36 g/dl) within a phospholipid bilayer membrane as an artificial oxygen carrier [Citation[8-12]]. Ferrous Hb (Hb(Fe2+)) molecules in the Hb vesicles gradually lose their oxygen binding ability because they react with ROS such as H2O2 [Citation[13], Citation[14]] and nitrogen monoxide (NO*) [Citation[15]] to become nonfunctional ferric Hb, in addition to the autoxidation of oxyHb itself. The concentration of metHb in the human erythrocyte is normally kept at less than 1% by reduction systems such as NADH-cytochrome b5, NADPH-flavin, and reductants such as glutathione and ascorbic acid. Furthermore, ROS are eliminated by SOD, catalase, or peroxidase to suppress metHb formation [Citation[16], Citation[17]]. In contrast, metHb molecules generated in the Hb vesicle are not reduced to the ferrous state due to the absence of the reduction systems, because the constitutive enzymes and reductants necessary for metHb reduction in the human erythrocyte are removed during Hb purification process [Citation[18]]. Furthermore, ROS elimination enzymes are also completely removed in the process. For these reasons, the resulting Hb vesicles are not effective against ROS, in particular, H2O2, which permeates the phospholipid bilayer membrane of the Hb vesicles. We are in the process of constructing an H2O2 elimination system using Hb vesicles in order to prolong the oxygen carrying ability of the vesicles. Coencapsulation of catalase in the Hb vesicle is an effective method; however, it has some practical problems such as the stability of catalase itself, the source and amount of catalase, and loss of the preferred long-term (two years) preservation at room temperature. The preservation period of donated blood is three weeks at 4°C; therefore, the long-term preservation of Hb vesicles is desirable for pharmaceutical and medical reasons. Because of these reasons, catalase is not used in Hb vesicles. Recently, we succeeded in the suppression of the conversion of oxyHb to metHb in the Hb vesicle by coencapsulation of metHb and L-Tyr in vesicles ((metHb/L-Tyr) Hb vesicles) and showed that this was effective in vitro and in vivo [Citation[19]]. In the Hb vesicle, during the reaction of metHb with H2O2, metHb itself is oxidized to ferrylHb radical. FerrylHb radical returns to the ferric state (metHb) by electron donation from two L-Tyr molecules. This reaction is regarded as the peroxidase activity of metHb. In the practical application of the method to the Hb vesicle, we incorporated an H2O2 elimination system in the Hb vesicle. This is a unique idea in the sense that “metHb is utilized for the suppression of metHb formation in the Hb vesicle.” Moreover, L-Tyr is a stable amino acid and is possible to encapsulate in the Hb vesicle. We consider the (metHb/L-Tyr) Hb vesicles as “the second generation of Hb vesicles.” However, the reduction process of ferrylHb radical to metHb by L-Tyr and its radical behavior during the reduction are still unknown. In addition, the chemical properties of the ferrylHb radicals are not well known. In this study, we analyzed the radical behavior of ferrylHb radical and its reduction process by electron spin resonance (ESR) and UV-vis spectrometry.
MATERIALS AND METHODS
Human Hb solution (Hb homogeneity > 99.9%) purified from human donated blood was a gift from Oxygenix Co., Ltd. (Tokyo, Japan). All reagents were used without further purification. Potassium ferricyanide, hydrogen peroxide (ultra pure grade), acetonitrile, and trifluoroacetic acid were purchased from Kanto Chemical Co., Inc. (Tokyo, Japan). Catalase (from bovine liver, 11500 unit/mg, lyophilized powder) was purchased from Wako Pure Chemical Industries, Ltd. (Osaka, Japan). L-Tyr was purchased from Tokyo Chemical Industry Co., Ltd. (Tokyo, Japan). Phosphate buffered saline tablets (10 mM PBS (pH7.4)) were purchased from Takara Bio Inc. (Otsu, Japan). Sephadex G-25 was purchased from Pharmacia AB (Uppsala, Sweden).
Preparations of metHb and ferrylHb Radical
MetHb was prepared by the reaction of HbCO (10 g/dl) with 2.5-fold excess potassium ferricyanide in the heme base. The unreacted potassium ferricyanide and ferrocyanide were removed by gel permeation chromatography (GPC, Sephadex-G25) developed by PBS (pH7.4). FerrylHb radical was prepared by the reaction of metHb with H2O2. H2O2 (4.5 mM) was added to the metHb (420 µM) solution and stirred for 1 min at 25 °C. After the reaction, the mixture was immediately cooled to 4°C and the remaining H2O2 was removed by GPC (Sephadex-G25) at 4°C. MetHb and ferrylHb were identified with a UV-vis spectrometry (V-570, Jasco, Tokyo, Japan) at 4°C and an ESR apparatus (9.059 GHz, 3.00 mW, JES-TE200, JEOL Ltd., Tokyo, Japan) at 4 K and 25 K controlled by a thermocontroller (MODEL 9650, Scientific Instrument Inc., Tokyo, Japan). The specification of the ESR tube was 5 mm ϕ and 270 mm L (JEOL Ltd.).
Temperature Stability of ferrylHb Radical
Temperature stability of the ferrylHb radical (2 µM) in PBS (pH7.4) was assessed with UV-visible spectrometry by repetitive scanning in the visible region from 300 nm to 700 nm at 2-minute intervals. The measurement was performed under strictly controlled temperature conditions at 4°C and 37°C. The percentages of the changed ferrylHb radical (the conversion of metHb) were calculated from the absorbance at 405 nm (λmax of metHb).
Reduction of ferrylHb Radical
Reduction of the ferrylHb radical (2 µM) by an excess amount of L-Tyr (500 µM) was assessed with the UV-vis spectrometry by repetitive scanning in the visible region from 300 nm to 700 nm at 2-minute intervals at 4°C. The percentage of the reduced ferrylHb radical (the conversion of metHb) was calculated from the absorbance at 405 nm (λmax of metHb). The reduction process of the ferrylHb radical was analyzed by ESR method. Immediately after preparation of a ferrylHb radical solution (110 µM), it was mixed with a solution of L-Tyr (1.8 mM) and stirred at 4°C. ESR samples were taken from the reaction mixture at 0, 1, 3, and 5 min, and each sample was immediately injected into the ESR tube (5 mm ϕ, 270 mm L, JEOL Ltd., Tokyo, Japan) and frozen at 196 K (liquid nitrogen).
Analysis of L-Tyr
During the reaction of the metHb ([heme] = 20 µM) with H2O2 (200 µM, [heme]/[H2O2] = 1/10) in PBS (pH7.4, at 37°C), the reaction mixture was periodically sampled out, and 200 µL catalase (5000 unit/mL) was added to eliminate H2O2. L-Tyr was analyzed with an HPLC (LC solution system, Shimadzu Co., Kyoto, Japan) equipped with an ODS column (TSK-GEL ODS-80Ts, 4.6 mm I.D., 250 mm L, Tosoh Co., Tokyo, Japan) after being separated from the Hb samples by centrifugal filtration (cutoff 5 kDa, Ultrafree-MC, Millipore Co., MA, USA). The separated solutions were injected into the column and developed with a mixed solvent (PBS/acetonitrile = 4/1, v/v) containing 0.3 vol% trifluoroacetic acid at a flow rate of 0.5 mL/min. The detection of solutes was performed at 240 nm.
RESULTS
Preparation of metHb and Isolation of ferrylHb Radical
A shows the UV-vis spectra of the prepared metHb and the isolated ferrylHb after the reaction of metHb with H2O2 and the subsequent removal of H2O2. The λmax of metHb in the Soret band at 405 nm shifted to 417 nm attributed to the λmax of ferrylHb. Moreover, the inset of A was the magnification of the Q band of the ferrylHb, showing disappearance of the peak at 630 nm, which was the specific absorption of metHb. These results indicated that metHb was completely converted to ferryl state of Hb by reaction with H2O2. The ESR results are shown in B. The signal of the isolated ferrylHb showed only a signal at g = 6 attributed the high spin state (S = 5/2) of ferric heme under the 4 K condition with liquid He; however, we could not confirm the signal at g = 2.006 attributed to the radical in the ferrylHb. On the other hand, both signals at g = 6 and g = 2.006 were detected at 25 K. ESR results at 25 K showed the metHb signal at g = 6 and g = 1.99 attributed to the ferric iron of the heme (C). In the case of the isolated ferrylHb, we confirmed the appearance of the signal at g = 2.006, which was attributed to the signal of the ferryl radical of Hb, and the decrease of the signal intensity at g = 6. From the results of UV-vis. and ESR measurements, the isolated ferrylHb possessed a radical in the molecule; therefore, it could be regarded as “ferrylHb radical” generally shown as Hb(Fe4+ = O*). Because this method produced the ferrylHb radical, we defined and represented the radical state of Hb as ferrylHb radical. We also confirmed that the ferrylHb radical before the separation of H2O2 showed almost the same signal as after removal of H2O2, suggesting that the ferrylHb radical can be isolated without any change of the radical state.
Figure 1 (A) UV-vis spectra of 2 µM metHb and 2 µM ferrylHb just after isolation by GPC at 4°C. Inset is the migration (x4) of Q band region. (B) Difference of ESR spectra of the isolated ferrylHb in PBS (pH7.4) at 4 K and 25 K. (C) ESR chart of the isolated ferrylHb radical and ferrylHb radical in the presence of H2O2 remaining in the reaction mixture in PBS (pH7.4) at 25 K.
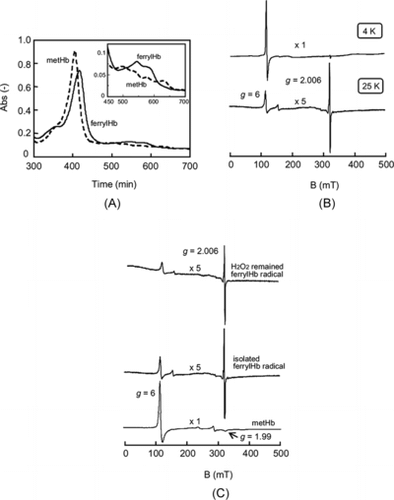
Temperature Stability of the ferrylHb Radical
The conversion of the ferrylHb radical to metHb was dependent on the temperature (A). At 4°C, the metHb conversion increased proportionally with time and finally reached 25% after 60 min. Conversely, at 37°C the conversion proceeded rapidly to 25% within 6 min, and finally reached a maximum of 70%. Therefore, the ferrylHb radical is more amenable to manipulation at 4°C.
Reduction of the ferrylHb Radical
The conversion of the ferrylHb radical to metHb in the presence of L-Tyr at 4°C is shown in B. The ferrylHb radical was more rapidly converted to metHb in the presence of L-Tyr compared with the control (the ferrylHb radical solution without L-Tyr). The conversion gradually increased and finally reached 65% after 60 min, whereas the conversion in the control solution was 25% after 60 min. shows the ESR signals during these reactions (in the presence and absence of L-Tyr) at 0, 1, 3, and 5 minute time points. The signal of the control solution at g = 2.006 (A), which was attributed to the ferryl radical of Hb, did not change in spite of the reaction time, whereas the rapid radical disappearance and the metHb conversion were confirmed in the L-Tyr-containing ferrylHb radical solution. The signal at g = 6 gradually increased, followed by the disappearance of the signal at g = 2.006.
Analysis of L-Tyr
The conversion of L-Tyr to the L-Tyr dimer (diTyr) during the reduction of the ferrylHb radical to metHb was studied using high pressure liquid chromatography (HPLC) (). The peak attributed to L-Tyr gradually decreased and simultaneously a new peak appeared between the retention times of 4 and 5 min. The new peak was identified as diTyr by the comparison with the elution time of diTyr synthesized by the method of Malencik et al. [Citation[1]]. This clearly showed that L-Tyr reacted with the ferrylHb radical to produce the diTyr.
DISCUSSION
Ferryl radical generation due to the reaction of heme protein with H2O2 is a well known phenomenon, and many researchers have investigated the behaviors and characteristics of the radical using recombinant Mb [Citation[20]]. FerrylHb radical and ferrylMb radical are known to be unstable intermediates of Hb and Mb known as Compound I. Ferryl radical spontaneously converts to the ferric state, known as autoreduction, and two tyrosines (Y103 and Y151) within the ferrylMb radical participate in the autoreduction [Citation[21]]. In other studies, the globin radicals in Hb or Mb generated by radical transfer from heme to the peptide chains were analyzed in detail using recombinant Mb [Citation[22], Citation[23]]. In particular, the two tyrosine residues (Y103 and Y151) and/or a tryptophan residue (W14) of Mb were shown to be involved in the generation of the globin radical [Citation[24-26]]. Although such theoretical studies regarding the autoreduction mechanism have been reported, the application to utilize the ferrylHb radical itself (e.g., enzymatic assay method, enzymatic synthesis) has not been reported. This is because the ferryl radical is unstable for handling and the high reactivity comes from the highly oxidative state of Compound I of heme-containing proteins. Therefore, we studied the chemistry of the ferrylHb radical in order to develop an application for this radical in the H2O2 elimination system.
First, we attempted to isolate the ferrylHb radical, which is generally considered to be an unstable intermediate. Alayash et al. reported the isolation of ferrylMb (Mb(Fe4+ = O). They reported the kinetics of the ferrylMb generation using recombinant Mb [Citation[27]]. However, they reported only the isolation of oxoferryl Mb, not the ferrylMb radical. We used their method of isolation of ferrylMb to isolate the ferrylHb radical. We could completely convert metHb to ferrylHb radical by reaction with H2O2 for 1 min at 25°C. As shown in A, we succeeded in the isolation of ferrylHb by cooling the solution down to 4°C after reaction at 25°C and the removal of H2O2 with Sephadex G-25 by keeping the temperature at 4°C. The radical of ferrylHb could not detect photometrically. However, radical in the ferrylHb was detected by the ESR measurements as described below; we represented the isolated ferrylHb produced by this method as “ferrylHb radical.” The appropriate amount of H2O2 for the reaction with metHb was determined to be a 2.5-fold molar excess relative to the heme of the metHb. This was because the ferrylHb radical conversion did not proceed to completion by adding a 1:1 molar equivalent of H2O2 to heme, in spite of the prolongation of the reaction time to 10 min. Furthermore, Hb was denaturated in a 10-fold molar excess of H2O2. In addition, it was technically impossible to remove such a large amount of H2O2 completely and rapidly from the reaction mixture with GPC. From these considerations, we finally applied a 2.5-fold molar excess of H2O2 to the heme to prepare the ferrylHb radical. ESR measurements at 25 K (C) confirmed the disappearance of the signal at g = 6 attributed to the high spin state (S = 5/2) of the ferric heme and the appearance of a new signal at g = 2.006 attributed to the ferrylHb radical, after the reaction of metHb with H2O2. The ESR spectrum of the isolated ferrylHb radical was almost the same as that of ferrylHb radical before the removal of H2O2, indicating that the H2O2 removal process with GPC at 4°C did not alter the molecule. In the ESR measurements shown in B, it is interesting that the isolated ferrylHb radical showed only a signal at g = 6 attributed to ferric heme at 4 K; the signal at g = 2.006 could not be detected. We could detect both signals at g = 6 and g = 2.006 when the temperature was raised to 25 K by liquid He.
The thermostability of the isolated ferrylHb radical measured using UV-vis spectrometry. The absorbance at 405 nm (λmax of metHb in the Soret band) of the isolated ferrylHb radical was regarded as the baseline absorption of metHb (0%), and the absorbance at 405 nm of the same concentration of pure metHb was regarded as 100% metHb. The percentage of the absorbance increase from baseline was plotted with time at 4°C and 37°C. Hence we regarded this increase as the conversion of the ferrylHb radical to metHb, namely, the autoreduction of the ferrylHb radical. The autoreduction mechanism of ferrylMb has already been reported [Citation[20], Citation[21], Citation[28]]. In the autoreduction process of sperm whale ferrylMb, it is thought that Y103 and Y151 residues are involved in the reduction of the ferryl radical by two electrons donation. Interestingly, Y146 does not contribute to the autoreduction even though it is closer to the heme iron than either Y151 or Y103. The autoreduction mechanism of the ferrylHb radical in this case is thought to be similar to the mechanism of ferrylMb, which involves intramolecular electron transfer to heme from the globin chain amino acid components such as tyrosine residues (Y103 and Y151) and a tryptophan residue (W14) [Citation[24-26]]. The rate of autoreduction was influenced by the temperature. Autoreduction was slower at 4°C than at 37°C. Aggregation was confirmed in the reaction mixture after UV-vis measurement at 37°C. Such instability of ferrylHb or its radical is well known to be related to both autoreduction and protein denaturation accompanying the release of heme or Fe ion [Citation[7], Citation[13]]. The isolated ferrylHb radical itself was comparatively stable under low temperature conditions (1–4°C) compared with at 37°C; therefore, we could stably handle it without significant autoreduction or denaturation by keeping low temperatures. We hypothesized that the ferrylHb radical could be utilized as a stable H2O2 elimination system by a enzymatic cycle.
We confirmed that the conversion of ferrylHb radical to metHb was enhanced in the presence of L-Tyr. We hypothesized that the ferrylHb radical could accept two electrons from two L-Tyr molecules, and that these L-Tyr molecules could form a bond at their ortho-positions to produce dimerized L-Tyr (DiTyr). Actually, DiTyr was detected in the reaction mixture of the ferrylHb radical and H2O2 in the presence of L-Tyr (). In considering the application of this system to the Hb vesicle in vivo, the temperature of interest is 37°C; however, the experiments of reduction of ferrylHb radical by L-Tyr were performed at 4°C in order to prevent autoreduction of ferrylHb radical itself. It should be kept in mind that the reduction of the ferrylHb radical by L-Tyr occurs more rapidly at 37°C than at 4°C. The mechanism of autoreduction is very complex, and is known to involve intra- and intermolecular transfer of globin radicals. Lardinois et al. reported that the cross-linked Mb dimmers were produced by coupling of the two Y151 tyrosine residues of two sperm whale Mb molecules as a result of electron transfer from the Y151 residue to the ferryl radical [Citation[29]]. In our study, the redox mechanisms involving the amino acid residues taking part in the autoreduction of the ferrylHb radical are still unclear. However, the direct reduction of highly oxidized heme by exogenous L-Tyr was shown to occur as evidenced by the generation of diTyr as shown in . Conversely, the autoreduced Hb molecules in our experiments might obey the rule of intra- and intermolecular transfers of globin radical in spite of L-Tyr presence. It is possible that the added L-Tyr was only involved in the direct reaction. Since metHb converted to ferrylHb radical by the process of H2O2 elimination and the generated ferrylHb radical was directly reduced to metHb by added L-Tyr, it was defined as an enzymatic reaction, and L-Tyr could be defined as a substrate in this enzymatic reaction.
In the case of the ferrylHb radical alone (A), the ESR signals after 1–5 minutes were almost the same as that of the sample at zero time. This indicates the stability of the ferrylHb radial at 4°C and agrees with the UV-vis measurement at 4°C as shown in A. This result also supports the notion that the autoreduction of the ferrylHb radical was dependent on the temperature. In contrast, in the case of the ferrylHb radical in the presence of L-Tyr (B), the signal at g = 2.006 attributed to the ferrylHb radical almost disappeared within 1 min after the addition of L-Tyr at 4°C. After 5 min, although the signal at g = 2.006 changed little compared with 1 min and 3 min, the signal at g = 6 attributed to the high spin state of ferric iron (metHb) increased. Since the autoreduction can be considered to be negligible under this reaction condition at 4°C, the result reflects the reduction process of the ferrylHb radical to metHb by L-Tyr.
We previously reported the H2O2 elimination ability of metHb in the presence of L-Tyr [Citation[19]]. H2O2 (200 µM) injected into a metHb solution (5 µM) was completely eliminated within 15 min in the presence of 1 mM L-Tyr, and Hb itself was not denaturated for 30 min. By contrast, a portion of the injected H2O2 remained after 30 minutes in the control reaction mixture and the denaturation products of Hb were found in the reaction mixture. The results proved that the cycle of H2O2 elimination was feasible.
As mentioned in the introduction section, we reported the application of this H2O2 elimination cycle to the Hb vesicles without detailed knowledge and theoretical discussion [Citation[19]]. We prepared Hb vesicles containing oxyHb (36 g/dl), metHb (4 g/dl), and L-Tyr (8.4 mM) for in vivo measurements using Wister rats (240–260 g). We determined the administration volume at 20 ml/kg as described in previous reports [Citation[10], Citation[12], Citation[14], Citation[19]].
In vivo measurements showed that the metHb percentages of the conventional Hb vesicles and metHb/L-Tyr containing Hb vesicles after 4 hr were 22% and 18%, respectively, and the times taken for the percentage of metHb to reach 50% in vivo were 14 hr and 44 hr, respectively. Furthermore, the Wistar rats were all alive until sacrifice after the measurement at 72 hr. These results confirmed the suppression of metHb formation in the metHb/L-Tyr containing Hb vesicles in vivo. This is important because the enzymatic eliminationof H2O2 shown in this report also worked in the Hb vesicles.
The authors thank Dr. T. Shibue at Material Characterization Center Laboratory of Waseda University for the technical advise and useful discussion about ESR measurement. This work was supported in part by a Health and Labor Science Research Grant from Ministry of Health, Labor and Welfare, Japan, a Research Grant from Ministry of Education, Science, Sports and Culture, Japan (MEXT, 18500368), and 21-COE “Practical Nano-Chemistry” from MEXT.
REFERENCES
- Malencik, D.A., Sprouse, J.F., Swanson, C.A., Anderson, S.R. (1996). Anal. Biochem. 242: 202–213.
- Giulivi, C., Romero, J.F., Cadenas, E. (1992). Arch. Biochem. Biophys. 299: 302–312.
- Giulivi, C., Cadenas, E. (1993). Arch. Biochem. Biophys. 303: 152–158.
- Giulivi, C., Cadenas, E. (1993). FEBS Lett. 332: 287–290.
- Galaris, D., Cadenas, E., Hochstein, P. (1989). Arch. Biochem. Biophys. 273: 497–504.
- Giulivi, C., Davies, J.K. (2001). J. Biol. Chem. 276: 24129–24136.
- Nagababu, E., Ramasamy, S., Rifkind, J.M., Jia, Y., Alaysh, A.I. (2002). Biochemistry. 41: 7407–7415.
- Sakai, H., Cabrales, P., Tsai, A.G., Tsuchida, E., Intaglietta, M. (2005). Am. J. Physiol. 288: H2897–H2903.
- Cabrales, P., Sakai, H., Tsai, A.G., Takeoka, S., Tsuchida, E., Intaglietta, M. (2005). Am. J. Physiol. 288: H1885–H1892.
- Sakai, H., Masada, Y., Horinouchi, H., Ikeda, E., Sou, K., Takeoka, S., Suematsu, M., Takaori, M., Kobayashi, K., Tsuchida, E. (2004). J. Pharmacol. Exp. Ther. 311: 874–884.
- Yoshizu, A., Izumi, Y., Park, S.I., Sakai, H., Takeoka, S., Horinouchi, H., Ikeda, E., Tsuchida, E., Kobayashi, K. (2004). ASAIO J. 50: 458–463.
- Sakai, H., Takeoka, S., Park, S.I., Kose, T., Hamada, K., Izumi, Y., Yoshizu, A., Nishide, H., Kobayashi, K., and Tsuchida, E. (1997). Bioconjugate Chem. 8: 23–30.
- Takeoka, S., Teramura, Y., Atoji, T., Tsuchida, E. (2002). Bioconjugate Chem. 13: 1302–1308.
- Teramura, Y., Kanazawa, H., Sakai, H., Takeoka, S., Tsuchida, E. (2003). Bioconjugate Chem. 14: 1171–1176.
- Herold, S., Rock, G. (2003). J. Biol. Chem. 278: 6623–6634.
- Shikama, K. (1984). Biochem. J. 223: 279–280.
- Tomoda, A., Yoneyama, T., Tsuji, A. (1981). Biochem. J. 195: 485–492.
- Naito, Y., Fukutomi, I., Masada, Y., Sakai, H., Takeoka, S., Tsuchida, E., Abe, H., Hirayama, J., Ikebuchi, K., Ikeda, H. (2002). J. Artif. Organs. 5: 141–145.
- Atoji, T., Aihara, M., Sakai, H., Tsuchida, E., Takeoka, S. (2006). Bioconjugate Chem. 17: 1241–1245.
- Svistunenko, D. A., Dunne, J., Fryer, M., Nicholls, P., Reeder, B.J., Wilson, M.T., Bigotti, M.G., Cutruzzola, F., Cooper, C.E. (2002). Biophys. J. 83: 2845–2855.
- Lardinois, O. M., Ortiz de Montellano, P.R. (2002). Biochemistry. 43: 4601–4610.
- Allentoff, A.J., Bolton, J.L., Wilks, A., Thompson, J.A., Ortiz de Montellano, P.R. (1992). J. Am. Chem. Soc. 114: 9744–9749.
- Wittig, P.K., Mauk, A.G., Lay, P.A. (2002). Biochemistry. 41: 11495–11503.
- Tew, D., Ortiz de Montellano, P.R. (1988). J. Biol. Chem. 263: 17880–17886.
- Davies M.J. (1991). Biochem. Biophys. Acta. 1077: 86–90.
- DeGray, J.A., Gunthur, M.R., Tschirrt-Gurh, R., Ortiz de Montellano, P. R., Mason, R.P. (1997). J. Biol. Chem. 272: 2359–2362.
- Alayash, A.I., Ryan, B.A.B., Eich, R.F., Olson, J.S., Cachon, R.E. (1999). J. Biol. Chem. 274: 2029–2037.
- Reeder, B.J., Wilson, M.T. (2001). Free Rad. Biol. Med. 30: 1311–1318.
- Lardinois, O.M., Ortiz de Montellano, P.R. (2003). J Biol. Chem. 278: 36214–36226.