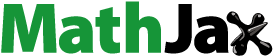
Abstract
Objectives. It is prescribed to determine blue-light hazard (BLH) weighted radiances, , for an assessment of spotlights with an angular subtense
. The BLH weighted irradiance,
, can be used alternatively for smaller sources. Appropriate instruments are not common among persons commissioned with risk assessment (RA), and especially
measurements may be challenging. Therefore, a practical BLH RA approach is proposed that is based on illuminance,
, pre-calculated blackbody BLH efficacies of luminous radiation,
, and solid angle considerations. Methods. The practicality of this method was examined and compared against other RA approaches. Results. To ensure comparability of the applied instruments, measurements were performed close to a radiance standard, showing deviations within the lamp’s expanded uncertainties (
), whereas the deviations were
for longer distances. Focusing on a complex light-emitting diode (LED) spotlight, all detected values could be converted to
by means of the RA methods within
. Two field tests with several spotlights yielded maximum permissible exposure durations (MPED) obtained from the different RA approaches that agreed among each other within uncertainties largely below
. Conclusion. The general practicality of the proposed
method can be concluded for a workplace BLH RA of white-light sources.
1. Introduction
The human eye plays a key role in the perception of our world because about of the surrounding’s information is gathered by the visual sense. It is therefore not surprising that artificial light is omnipresent at workplaces day and night, e.g., at the office in the form of screens (laptops, tablets, smartphones) or lighting. Such light sources do not pose any risk for photochemically induced acute retinal damage [Citation1], typically referred to as blue-light hazard (BLH). General lighting based on light-emitting diodes (LEDs) can also be regarded as BLH safe [Citation2–4].
In contrast to the aforementioned low-power light sources, very bright spotlights are necessary for structural and civil engineering, for large storage areas or in theaters, news studios and on stages, which might pose a BLH risk for workers [Citation5,Citation6]. If it is not undoubtedly clear whether the BLH exposure limits (EL) are either well exceeded or complied with at the workplace, the emitted light must be assessed metrologically. The International Commission on Non-Ionizing Radiation Protection (ICNIRP) published recommendations for EL in their ‘Guidelines on limits of exposure to incoherent visible and infrared radiation’ [Citation7]. Therein, it is specified under which conditions depending on the angular subtense of the source, , a risk assessment (RA) can be based on the BLH weighted irradiance,
, or requires knowledge about the BLH weighted radiance,
.
The determination of may be challenging, and appropriate devices are expensive. Radiance meters are error-prone because of their high field-of-view (FOV) sensitivity, so experienced operators are needed to achieve reliable and reproducible results. Fiorentin and Scroccaro [Citation8] write that ‘it requires pretty sophisticated instruments to measure the involved quantities’ (792); thus, alternative and practical methods are preferable to lower the hurdles for a BLH RA. A common way to circumvent radiance measurements is the conversion of
to
by means of the source’s solid angle, Ω, seen at the position of the detector. This procedure is standardized in ‘The Lamp Safety Standard’ IEC 62471:2006 [Citation9] and will be described in more detail later.
Several research groups also attempted to find appropriate solutions. By relating to the illuminance,
, of about 100 lamps, Schulmeister et al. [Citation10] derived a transformation factor in order to express the BLH EL in terms of illuminance. The permitted
values were presented as a function of correlated color temperature (CCT). They concluded that at the ‘
distance’ the BLH EL according to the ICNIRP [Citation7], the International Electrotechnical Commission (IEC) [Citation9] and the American Conference of Governmental Industrial Hygienists (ACGIH) [Citation11] are not exceeded, except for very high CCTs.
Shibuya et al. [Citation12,Citation13] applied a hyperspectral camera to examine the effect of non-uniform luminance, , distributions on BLH risk levels. They assessed the light emissions of LED luminaires, incandescent and fluorescent lamps at the standardized ‘
distance’ [Citation9], demonstrating the usefulness of two-dimensional light profiles. Liu et al. [Citation14] pursued a similar method combining the measured relative spectral power distribution with the maximum
value determined from a camera for LEDs and compact fluorescent lamps. However, such imaging luminance meters are even more expensive than radiance instruments, their handling is also demanding and they cannot be used at workplace-typical large distances.
Other RA approaches applied the BLH efficacy of luminous radiation, , i.e., the ratio of
to
or
to
. Fantozzi et al. [Citation15] describe a simplified BLH RA method according to IEC TR 62778:2014 [Citation16] based on CCT-dependent photometric threshold curves between risk group (RG) 1 and RG 2. By means of
, the
and
values measured at a distance of
can be applied for a quick RG check: if both values are smaller than the threshold curves, the lamp is classified as RG 1 at most, thus posing no photobiological risk. Otherwise, two different quantities must be determined. After the present study had been completed, the new revision of IEC 62471-7:2023 was published [Citation17] and is currently discussed to include the threshold curves from IEC TR 62778:2014 as an informative appendix.
A mere analytical approach parametrized the light emission spectra of phosphor-converting white LEDs (pc-wLEDs) via a linear combination of two Gaussian functions [Citation18]. A comparison of calculated with experimentally determined values revealed percentage deviations better than
. Although this finding demonstrates its general applicability, it remains unclear how to transfer results derived from normalized single pc-wLEDs to an actual workplace situation. The so-called ‘Planck Approximation’ [Citation19] is another RA method using
. It is based on a CCT-dependent pre-calculated
value for a blackbody radiator,
, in conjunction with a single
measurement at the exposure site. Considering
(assuming a circular extent of the source), the intended FOV and the BLH EL, three simple equations for the maximum permissible exposure duration (MPED; the exposure duration resulting in an exposure level equal to the EL) were derived.
The aim of this work is to evaluate the uncertainty and practicality of the Planck Approximation with regard to other RA approaches within the Partnership for European Research in Occupational Safety and Health (PEROSH) project ‘High-Power Spotlights Risk Assessment’ (HiPoSisAs). For a thorough analysis, the inter-comparability of the HiPoSisAs partners’ instruments was tested by means of a calibrated radiance standard. The applied RA approaches were evaluated by more demanding measurement conditions for a multi-chip RGBW spotlight. Field tests completed this evaluation.
2. Materials and methods
2.1. Basic equations for BLH risk assessment
Most photobiological effects are wavelength dependent and can be described by an action spectrum that is used for spectral weighting. Regarding photochemically induced retinal damage, is determined via the convolution of the source’s spectral irradiance,
, with the BLH action spectrum,
, in the wavelength range
[Citation7]:
(1)
(1) The convolution of
with the photopic luminous efficiency function,
, that reflects the brightness perception of the human eye [Citation20], results in
:
(2)
(2) The constant
represents the maximum spectral luminous efficacy. Replacing
by
in Equations (1) and (2) yields
and
, given as
(watt per square meter per steradian) and as
(lumen per steradian per square meter = candela per square meter), respectively.
For a BLH RA of light sources with an angular subtense , it is necessary to determine
based on the solid angle. The Ω value is calculated by the ratio of a spherical calotte with area
and the sphere’s radius d. A common approximation is the use of a plane circular area with diameter D instead of
. The Ω value can also be described via the resulting cone’s opening angle
:
(3)
(3) Although the solid angle is a dimensionless quantity, it is usually expressed in units of steradian. The relation between radiance and irradiance follows as:
(4)
(4) The ratio of
and
, or equally of
and
, is a common quantity to characterize white-light sources [Citation16], and the resulting
is usually presented as a function of CCT,
:
(5)
(5) The Planckian radiator’s
value is calculated the same way and can be described empirically via an S-shaped four-parameter logistic function [Citation19]:
(6)
(6) with saturation value
(watt per kilo lumen), with temperature
for that the curvature changes direction or sign, with hill slope
(steepness of the curve) and asymmetry parameter
(with respect to
,
corresponds to symmetry). Note that
is constant for any fixed CCT; thus, it can be pre-calculated (see Appendix 1) and directly used on site at workplaces.
2.2. Exposure limits and averaging angles
The BLH EL according to the ICNIRP [Citation7] are expressed in terms of weighted quantities and depend on the exposure duration, t, as well as on the angular subtense of the source, :
(7a)
(7a)
(7b)
(7b) For sources with
, the EL are given as BLH weighted radiances whereas the alternative irradiance limit may be determined for smaller sources.
and
are time-dependent for exposure durations shorter than 10,000 and
, respectively; otherwise, they are constant values.
For determination of the exposure level, must be spatially averaged over a certain FOV. The ICNIRP recommends a time-dependent
value between a lower (
) and an upper (
) limit with the important note that ‘If the visual task and the behavior can be characterized, a safety analysis can account for more realistic eye movements and a larger averaging angle can be used’ (80) [Citation7]. IEC 62471:2006 [Citation9] defines more or less the same
values but with an upper limit of
. In praxis, it is common to average
over 11 or
, both constant, and most instruments are designed for these two FOVs.
2.3. Instruments
Each partner of the HiPoSisAs project brought its own device(s) that is (are) usually applied for BLH RA at workplaces. Table presents these 21 instruments, first with regard to their measurands and then in alphabetical order. For the sake of simplicity, a shortcut is assigned. If different sensors or entrance optics (EOP) can be mounted, the applied one is indicated.
Table 1. Devices used for the instrument comparison and the RAs.
Participants were encouraged not to perform a recalibration before the two measurement series, one in May 2022 and the other in March 2023. This is important to allow for a best possible field instrument comparison because recalibrations are usually done no more than once a year. However, as can been seen in Table , the variety of (re)calibration dates is quite large: seven devices had not been calibrated for at least 3 years whereas seven instruments were calibrated prior to the start of the measurement campaign in 2022. In addition, the institute operating the Flame is accredited according to ISO/IEC 17025:2017 [Citation21] to test to IEC 62471:2006, even on site; thus, the device is recalibrated very frequently. Note that not all HiPoSisAs partners were able to take part in each measurement series.
2.4. Radiation sources and measurement locations
A Bentham Halogen Spectral Radiance Standard (SRS 8) with a ground glass window of diameter was measured by most devices presented in Table to test their inter-comparability. The SRS 8 is equipped with a
quartz-halogen lamp placed in an integrating sphere of
diameter coated with BaSO4. Its spectral radiance was calibrated last time in August 2019. Based on the expanded uncertainty with coverage factor
, the SRS 8 BLH weighted radiance,
, and luminance,
, were calculated by means of Equations (1) and (2), respectively.
A Robe Spiider washbeam luminaire was used to compare RA approaches under increased measurement demands. The spotlight is equipped with 18 circularly arranged 40 W RGBW LED multichips and one centered 60 W RGBW LED multichip, all situated within a measured inner front diameter of ; however, the actual emitting surface is much smaller due to empty spaces between the LEDs. Measurements were performed at
(equal to
) and at
(in-laboratory exposure scenario), approximately at eye level (
). The Robe Spiider was operated at
intensity and 150/255 zoom with a DMX-controlled CCT of Tcp = 5600 K.
The field tests were performed in two different places. The spotlights of setting I (see Table ) were compiled and arranged by the authors in a large room. It was necessary to perform these measurements in a controlled surrounding to be able to try and discuss the applied RA approaches. Setting II was a stage used commercially for varying events. The three spotlights presented in the lower part of Table were chosen from eight different options. At both settings I and II, the horizontal distances were large enough so that no spotlights overlapped with each other within a FOV of .
Table 2. Lamp characteristics and measurement distances for Bentham’s Spectral Radiance Standard SRS 8 (inter-comparability test), the Robe Spiider (demanding measurement conditions), for the in-laboratory exposure scenario (setting I) and at the assessed workplace (setting II).
2.5. BLH risk assessment methods
The basic idea of any BLH RA approach is to identify each spotlight that a worker might look at during an 8h working day, and to find out (by asking about the visual task and the behavior) for what length of time eye exposures accumulate. Then, a comparison of the determined or
values with the related EL in Equations (7a) and (7b) allows the calculation of MPED and thus a recommendation concerning BLH workplace safety.
BLH RA methods that are common among the HiPoSisAs partners will be presented in the following. Note that glare usually prevents long-time viewing into the source, so that exposure durations less than will be assumed hereafter for an 8h working day. Consequently, only the upper parts of Equations (7a) and (7b), the time-dependent parts, must be regarded.
2.5.1. Lamp safety standard approach
The value can always be detected and directly compared to the EL of Equation (7a). However, this can lead to problems for sources with
, as discussed later with respect to the Showtec Stage Blinder 2. In addition, radiance instruments are expensive, and an experienced operator is needed, e.g., because of potential misalignments. IEC 62471:2006 [Citation9] defines
for exposure durations of t ≥ 10,000 s with the related EL equal to
, in accordance with the ICNIRP. If the measured
value is smaller than this EL, no MPED needs to be considered.
The determination of is usually based on an open FOV and not limited by an angle of acceptance. The respective irradiance EL of Equation (7b) can be applied for sources smaller than
. Using this method also in the case of
, the exposure distance,
, and the source diameter, D, must be known to calculate
(or equally
) via Equation (4) in conjunction with Equation (3). In a simple approach, the open FOV
value can be directly compared to the irradiance EL of
stemming from the multiplication of
and Ω = 9.5 msr for ICNIRP’s
.
2.5.2. Luminance distribution approach
A BLH RA should consider the spectral as well as the spatial distribution of the light source, because the retina’s sensitivity is wavelength dependent and the highest risks are found where the source’s image is formed on the retina. Consequently, a spectral irradiance measurement together with the luminance distribution, , aim to collect the required information. For this combined method, first, the measured
value is used to determine
via Equations (1), (2) and (5). Then, the spatial luminance distribution recorded with a specifically calibrated camera is converted to
by means of this experimental
value. Based on the new image, considering the exposure duration and distance, it is possible to average
in the area that is limited by the averaging angle
.
2.5.3. Alternative illuminance approach
An alternative BLH RA method is based on the CCT-dependent pre-calculated (see Appendix 1), in conjunction with a single
measurement at the distance of exposure,
[Citation19]. The spotlight’s CCT can be found either in the manual (for fixed
) or is set by the operator via DMX. The underlying assumption that
is close to the ‘actual’
value is fulfilled quite well not only for halogen lamps, but also for many other white-light sources like LED spotlights [Citation16,Citation19]. It is important to emphasize that a mismatch error of
and
is smaller than any uncertainty resulting from, e.g., a poor radiance detector alignment or imprecisely determined exposure conditions such as the distance, duration or viewing angle. In particular, the latter is difficult to find out precisely and results in the largest error.
Combining the described approach with the first lines of Equations (7a) and (7b) and the L-to-E conversion via Ω in Equations (3) and (4) yields:
(8)
(8) as explained in the following. Provided that the distance
between the spotlight and the exposed person is sufficiently long, the angular subtense of the source is smaller than
, and the EL is expressed as irradiance. A direct conversion of the measured
to
can be done via Equation (5), yielding the first line of Equation (8). For sources with
, the radiance EL must be used. According to the left side of Equation (3), Ω = 7.85 msr for
leading to the second line of Equation (8).
A different situation exists if is larger than the applied acceptance angle
. Then, the spatially averaged ‘biologically relevant’ radiance is equal to the distance independent ‘physical’ one, at least in theory neglecting inhomogeneities of the emitting surface, and the right side of Equation (3) must be considered with a variable Ω. The resulting third line of Equation (8) can also be applied for a worst-case simplification: the over-restrictive assumption that light from a source with angular subtense
is emitted from a constant
(
) combined with the time-dependent EL of
leads to the first line of Equation (8) that can be regarded as a worst-case approach for all types of
–
combinations.
3. Results
3.1. Instrument inter-comparability test
The optical radiation emitted by the SRS 8 ground glass window was detected at a distance of . Taking into account its diameter
, the angular subtense of the source is equal to
. Some of the devices presented in Table also performed measurements at two additional distances,
(
) and
(
). Because of spatial limitations of the experimental set-up,
(
) could not be realized.
The measured and
values were weighted with the BLH action spectrum and the photopic luminous efficiency function according to Equations (1) and (2).
and
values were converted to
and
, respectively, by means of Equations (3) and (4). An average value was determined from two-dimensional luminance distributions,
, by the operating software. All of these data are presented in Figure as percentage deviations with regard to the calibration values
and
(coverage factor
).
Figure 1. Percentage deviations of measurement results at three distances with regard to (a) the luminance and (b) the blue-light hazard (BLH) weighted radiance of the SRS 8. Note: The instruments labeled according to Table are grouped in terms of their measurands on the upper abscissa. The gray hatched areas reflect the expanded calibration uncertainties with coverage factor .
= percentage deviation of measured/calculated to calibrated BLH weighted radiance;
= percentage deviation of measured/calculated to calibrated luminance;
= spectral irradiance;
= illuminance;
= BLH weighted radiance;
= spectral radiance;
= luminance;
= two-dimensional luminance distribution; SRS 8 = Bentham Halogen Spectral Radiance Standard.

Figure (a) shows that most of the devices were able to determine within the expanded uncertainty (gray hashed area) or that they were close to it for distances of
and
. The mean value of the absolute (positive) deviations,
, is given by 2.6 ± 1.7%, which is an excellent outcome. Examining the
data reveals that the percentage deviations are much higher at
, reaching up to
, and that the
and
instruments detect too much, except for the VL-TEC that needed to be recalibrated afterwards, in contrast to the
,
and
devices. Both findings can be explained with the detection of reflected light from the surrounding. At a distance of
to the SRS 8, the
value becomes very small (<10 lx) approaching the lower end of the detectors’ sensitivity ranges. As a result, the relative contribution of detected reflections to the actual values increases leading to higher
values. Radiance and luminance are virtually unaffected by light from the surrounding.
Percentage deviations with regard to the calibrated BLH weighted radiance, , are depicted in Figure (b), and show findings more or less consistent with those for
whereby individual
values are higher up to
at
and
. For both distances, the mean of
yields 4.2 ± 3.1% and therefore is close to the
average. A comparison of both PD-BLH instruments (identical types) demonstrates the effect of either varying calibrations and/or the operators’ handling.
Summarizing the inter-comparability test, it can be stated that all participating instruments can be compared among each other within percentage deviations of for sufficiently high illuminance levels.
3.2. Demanding measurement conditions
While the instrument comparison described in the previous section was intended to verify the devices’ inter-comparability, the second test not only increases measurement demands regarding inhomogeneities of the emitting surface and the optical output power but also applies all RA approaches described in Section 2.5. For this, Robe’s Spiider was chosen with luminance levels in the order of (mega candela per square meter). As can be seen in the iso-luminance distribution depicted in Figure (a), each of the eighteen 40 W and the centered 60 W RGBW LED have different
values. In addition, most of the front side does not emit light so that the
and
FOVs shown (green color) do not correspond to the actual extent of the illuminating surface. This strongly varying light emission distribution is reflected in the large standard deviations of the mean luminance values of 2.1 ± 6.7
(
) and 18 ± 11
(
) determined by the Opticam software.
Figure 2. (a) Iso-luminance distribution of a Robe Spiider measured with an Opticam at a distance of . (b) Blue-light hazard (BLH) weighted radiances at
with their mean of
shown as the dashed line and gray hatched area (not considering the LDM-BLH and Opticam values). Note: The color scale in panel (a) (not shown) ranges up to a maximum of
(white). The green circles represent 11 and
fields of view (FOVs).
= spectral irradiance;
= illuminance;
= BLH weighted radiance;
= spectral radiance;
= two-dimensional luminance distribution.

Figure (b) summarizes the measurement results obtained at a distance of (equal to
). Spectral radiance,
with
, and irradiance,
, were convoluted with
according to Equation (1). (Il)Luminance was converted to BLH weighted (ir)radiance by means of Equations (5) and (6) with
for
(see Appendix 1).
was subsequently converted to
via Equations (3) and (4).
It is striking that the LDM-BLH value of 28.8 kW m−2 sr−1 strongly deviates from the results of all other devices although it matched the expanded
uncertainty of the SRS 8 in Figure (b). A discussion with the experienced operator revealed that the most likely explanation is incorrect handling of the photometer that is coupled to the LDM-BLH and whose FOV parameter must be set either to
,
or
. If the emitted light is detected within
but is mistakenly associated with a
setting, such a large deviation can occur.
The mean (not considering the LDM-BLH and the Opticam) is given by 2.8 ± 0.2
, equal to a standard deviation of
and comparable to the previous findings for
and
. Note that the averaged
of the imaging Opticam was not considered because of its large standard deviation. The median for all
values is 2.9 kW m–2 sr–1.
Close attention must be paid to the HCT and VL-3701 with respect to the alternative illuminance approach described in Section 2.5.3. Despite the complex distribution of the Spiider and the approximation of
with
, the measured and converted illuminances are as accurate as the other results.
3.3. BLH risk assessment field tests
Changing from rather close measurement distances (with ) and laboratory conditions to (almost) real-life situations, two field tests were performed as described in Section 2.4. Each spotlight was assessed via radiance, irradiance and illuminance measurements. The results are presented in Table .
Table 3. BLH assessment results for the spotlights of the in-laboratory exposure scenario and of the workplace.
For sources with , MPED values based on
were calculated via Equation (7a). Irradiance was converted to
values by means of Equations (3) and (4) and subsequently compared with the radiance BLH EL of 1 MJ m−2 sr−1 t−1. For the alternative
approach,
was taken from Appendix 1 with the CCTs presented in Table . Based on the restrictive assumption of an
FOV, the angular subtense of 5 from the 8 spotlights is larger than
, and the third line of Equation (8) must be used.
The beam diameters of the MegaPointe and the Quantum Profile were measured to be approximately equal to and
yielding angular subtenses of
and
, respectively. For these sources,
and
values were compared with their respective EL. The illuminance RA method can be applied with the first line of Equation (8) that is also equal to the worst-case approach with the most restrictive MPED,
. A detailed discussion of the Showtec Stage Blinder 2 as well as of all MPEDs is presented in the following sections.
4. Discussion
During the in-laboratory exposure scenario, problems arose with regard to both PAR 56 spotlights. Frequently switching them on and off presumably led to a varying filament temperature, resulting in a different light emission that is also reflected in the MPED. At the workplace, the distribution method (v), based on the bracketing technique, failed for the Robe Robin 600+ (Table ). A real-time recording revealed that the emission of the spotlight’s LEDs were rotating and thus flickering. Furthermore, the HiPoSisAs partners planned to assess a Martin Atomic 3000 flashlight (xenon discharge lamp), not presented in Table , but its pulsed emission yielded noisy spectra being close to zero, caused by a mismatch of the integration time, pulse length and spectral averaging. Special equipment will be necessary for a RA of the Atomic 3000.
4.1. Showtec stage blinder 2
Although photobiologically safe, the Showtec Stage Blinder 2 resulted in the longest discussion among the HiPoSisAs partners. As can be seen from its luminance distribution shown in Figure , the spotlight consists of two separate sources with their filaments being rotated by . One RA approach, carried out by the PD-BLH-2 instrument (ii), measured
with
for each lamp individually. Assuming that light from both parts is focused on one retinal site, their
sum is converted to one
value and compared to the irradiance BLH EL, but using an averaged diameter of
(substituting both emitting areas by a single circular one) instead of
. The resulting MPED is given as
. A similar approach was taken by the Specbos-ACC (iii) applying the doubled mean
value of both lamps (MPED of
). The Flame (viii) spectroradiometer determined the maximum
value from the brightest of the two sources, yielding an MPED of
.
Figure 3. Two-dimensional luminance distribution, , of the Showtec Stage Blinder 2 recorded by the Canon camera. Note: The
scale is given as
. The white double arrow represents the spotlight’s diameter of D = 8.5 cm. The red circle visualizes
. D = front side inner diameter;
= field of view (FOV);
= luminance;
= two-dimensional luminance distribution.

The latter two instruments also followed the RA approach based on IEC 62471:2006. Measurements with provided
values well below
so that no MPED must be considered, and the Stage Blinder 2 can be regarded as BLH safe.
recorded by the Canon camera (v) was used to identify the maximum luminance,
, of both parts. In combination with an experimentally determined
from the AvaSpec-1 (v), this maximum luminance was converted to
via Equation (5) yielding a MPED of
.
4.2. Comparison of maximum permissible exposure durations
In order to compare all MPED values presented in Table , mean values with standard deviations (absolute and percentage) were calculated. Over-restricted MPED, , values were not considered.
Regarding the in-laboratory exposure setting (upper part of Table ) most MPED values agree among each other except those of the irradiance and illuminance RA approach for the ARRI L7-C and for the MegaPointe, respectively. Potential detector misalignments, different calibrations or a -to-
mismatch (for the
method) can be reasons for this. Percentage deviations of the radiance and irradiance RA approaches for the Spiider are higher than
and thus do not meet the maximum
measurement uncertainty given by EN 14255-2:2005 [Citation22], whereas the
method is more accurate. However, the validity of this finding is not sufficient taking into account only two different instruments for each RA method. The irradiance and illuminance RA approach for the PAR 56 (B) also have percentage deviations larger than
. In general, increasing the number of instruments for the workplace RA (lower part of Table ) improves the statistics. Except for the Stage Blinder 2, all MPED values agree among each other within their percentage deviations.
Table 4. Mean MPED calculated on the basis of the data presented in Table .
Overall, it is notable that the alternative illuminance RA approach has the lowest standard deviation for six of the eight assessed spotlights. In accordance with previous findings [Citation19], it can be concluded that the method is applicable for a BLH workplace RA of the tested white-light sources just as well as the radiance or irradiance approach.
5. Conclusion
The idea of a simple and practical BLH RA approach arose from demanding radiance measurements with expensive equipment, whereas luxmeters are easy to handle, cost-effective, usually available at workplaces with high-power spotlights and thus known by persons commissioned with performing a RA. Combining a single measurement, a pre-calculated tabular
value for a blackbody radiator (for given CCT) and some solid angle considerations yielded three simple equations for the MPED. It was the aim of the present work to evaluate the applicability of this alternative illuminance RA method.
In order to make a statement regarding inter-comparability, first, the applied instruments were tested with respect to and
values of a comparably low-power halogen radiance standard. The determined values were found within or near to the expanded uncertainties (
) for close distances. Otherwise, they deviated up to
as the consequence of a small illuminance (Ev < 10 lx) challenging the detectors’ lower dynamic ranges. In a second step, the light emission of a complex high-power spotlight was detected. Most measurement results including those from the alternative illuminance RA approach were presented by means of
and were found within a standard deviation of
. Finally, two exposure settings were assessed, a semi-in-laboratory setting and a workplace setting, with eight spotlights in total. Not all of the RA methods could meet the
maximum measurement uncertainty criterion given by EN 14255-2:2005 for every spotlight. However, most of the standard deviations fulfilled this requirement, and those from the illuminance RA approach were the smallest (<20%) for six of the eight spotlights. Concluding these findings, the alternative
method is at least as accurate as the radiance or irradiance RA approach; thus, its practicality can be stated for a BLH RA of white-light sources at workplaces. For this, it is recommended to use luxmeters that have a certificate of calibration.
For a continued assessment of the alternative illuminance RA method, a larger number of different spotlights should be considered to characterize potential problems, e.g., regarding sources with a specific color where the experimental value does not agree with the
value. During the preparation of this article, the Austrian Workers’ Compensation Board (AUVA) and Seibersdorf Laboratories were conducting detailed measurements on selected spotlights (on site and in the laboratory) with the aim to compare results obtained by using simplified methods for photochemical and photothermal retinal hazards with those from a highly accurate ‘artificial eye’ set-up for radiance determination. Their findings will provide further information about the practicality of this work’s alternative illuminance RA approach.
Acknowledgements
The authors thank PEROSH for providing the framework within the HiPoSisAs project took place. The regular support of PEROSH but also that of the participating occupational safety and health institutes (AUVA, BAuA, CIOP-PIB, IFA, INAIL, INRS, Unisanté) and cooperative partners (DGUV, GL Optic, JETI, OSRAM, Seibersdorf Laboratories) allowed the experimental evaluation of the proposed illuminance BLH RA approach on a European level.
Disclosure statement
No potential conflict of interest was reported by the authors.
References
- O’Hagan J, Khazova M, Price L. Low-energy light bulbs, computers, tablets and the blue light hazard. Eye. 2016;30:230–233. doi:10.1038/eye.2015.261
- Ion RM, Proykova A, Samaras T, et al. Scientifc Committee on Health, Environmental and Emerging Risks (SHEER). In: Opinion on potential risks to human health of light emitting Diodes (LEDs); 2018. p. 92. doi: 10.2875/605415.
- International Commission on Illumination. CIE position statement on the blue light hazard. Color Res Appl. 2019;44(4):672–673. doi:10.1002/col.22389
- International Commission on Non-Ionizing Radiation Protection. Light-emitting diodes (LEDs): implications for safety. Health Phys. 2020;118(5):549–561. doi:10.1097/HP.0000000000001259
- Hietanen MTK, Hoikkala MJ. Ultraviolet radiation and blue light from photofloods in television studios and theaters. Health Phys. 1990;59(2):193–198. doi:10.1097/00004032-199008000-00005
- Salsi S, Barlier-Salsi A. Exposure to scenic lighting devices: risk to the health of entertainment professionals. Radioprotection. 2013;48(3):391–410. French. doi:10.1051/radiopro/2013064
- International Commission on Non-Ionizing Radiation Protection. ICNIRP guidelines on limits of exposure to incoherent visible and infrared radiation. Health Phys. 2013;105(1):74–96. doi:10.1097/HP.0b013e318289a611
- Fiorentin P, Scroccaro A. A simplified approach to the assessment of photobiological safety of led sources. In: 2014 IEEE International Instrumentation and Measurement Technology Conference (I2MTC) Proceedings, Montevideo, Uruguay; 2014, May 12–15. p. 792–796. IEEE.
- Photobiological safety of lamps and lamp systems. Geneva: International Electrotechnical Commission (IEC); 2006. IEC 62471:2006.
- Schulmeister K, Buberl A, Weber M, et al. Simplified method to assess the UV and blue light hazard of lamps. In: International Congress on Applications of Lasers & Electro-Optics. ICALEO 2013: 32nd International Congress on Laser Materials Processing, Laser Microprocessing and Nanomanufacturing. Orlando (FL): Laser Institute of America (LIA); 2013, March 18–21. p. 357–365. ICALEO.
- American Conference of Governmental Industrial Hygienists. 2009 TLVs and BEIs: threshold limit values for chemical substances and physical agents and biological exposure indices. Cincinnati (OH): ACGIH; 2009.
- Shibuya T, Akiba T, Iwanaga T. Research note: measurement of blue light hazard risk level using a hyperspectral camera. Lighting Res Technol. 2020;52(5):692–697. doi:10.1177/1477153519893419
- Shibuya T, Akiba T, Iwanaga T. Assessment of the blue light hazard for light sources with non-uniform luminance. LEUKOS. 2021;17(2):205–209. doi:10.1080/15502724.2020.1774775
- Liu J, Zhuang XB, Yao H, et al. Methodology for measurement of the blue light hazard of light-emitting diodes with imaging luminance meter. In: Applied Mechanics and Materials, Vol. 455; 1. Trans Tech Publications, Ltd; 2013. p. 460–465. doi:10.4028/www.scientific.net/amm.455.460
- Fantozzi FF, Rocca M, Burattini C, et al. Simplified assessment of blue light emissions based on photometric measurements. In: 2018 IEEE International Conference on Environment and Electrical Engineering and 2018 IEEE Industrial and Commercial Power Systems Europe (EEEIC/I&CPS Europe); 2018. p. 1–6. IEEE.
- Application of IEC 62471 for the assessment of blue light hazard to light sources and luminaires. Geneva: International Electrotechnical Commission (IEC); 2014. IEC/TR 62778:2014. Withdrawn 2023 May.
- Photobiological safety of lamps and lamp systems – part 7: light sources and luminaires primarily emitting visible radiation. Geneva: International Electrotechnical Commission (IEC); 2023. IEC 62471-7:2023.
- Bauer S. Blue-light hazard of light-emitting diodes assessed with Gaussian functions. Int J Environ Res Public Health. 2021;18(2):1–18. doi:10.3390/ijerph18020680.
- Bauer S. Blue-light hazard risk assessment of incoherent high-power spotlights-The Planck Approximation. BAuA report. 1st ed. Dortmund: Federal Institute for Occupational Safety and Health (BAuA); 2022. doi:10.21934/baua:bericht20220825
- Colorimetry – part 1: CIE standard colorimetric observers. International Organization for Standardization/International Commission on Illumination; 2019. ISO/CIE 11664-1:2019.
- General requirements for the competence of testing and calibration laboratories. International Organization for Standardization/International Eletrotechnical Commission; 2017. ISO/IEC 17025:2017.
- Measurement and assessment of personal exposures to incoherent optical radiation – part 2: visible and infrared radiation emitted by artificial sources in the workplace. Brussels: European Committee for Standardization (CEN); 2005. EN 14255-2:2005.
Appendix 1.
Calculated values for 

Table A1. CCT-dependent BLH efficacy of luminous radiation for a Planckian radiator calculated according to Equation (6).