Introduction
Worldwide, 69 million people sustain traumatic brain injury (TBI) annually (Citation1). The incidence of TBI in low- and middle-income countries is three times greater than in high-income countries, with fatality rates ranging from as low as 5.2/100,000/year in France to as high as 80.73/100,000/year in South Africa (Citation2).
TBIs may range from mild, including concussions, to severe, including coma and death. In general, a TBI is caused by a direct or indirect force to the brain that disrupts normal brain function (Citation3). The vast majority of TBIs are mild, but distinguishing mild injury from more severe TBI in the prehospital setting may not be immediately apparent. Severe TBI is a leading cause of morbidity and mortality, resulting in 2.87 million TBI-related emergency department visits, hospitalizations, and deaths in the United States annually. Approximately one-third of these events occurred in children (Citation3). The likelihood of moderate-severe TBI is heightened in any prehospital patient sustaining physical trauma with Glasgow Coma Scale (GCS) score <15, loss of consciousness, multisystem trauma requiring an advanced airway, or report of post-traumatic seizure (Citation4).
The mortality rate associated with blunt traumatic injury is exponentially increased when associated with TBI. Death from severe TBI often occurs within the first few hours following injury. Prehospital and early management of the primary injury with prevention of secondary brain injury and avoidance of secondary iatrogenic brain insults are critical to maximizing outcomes. Secondary brain injury is a pathophysiologic injury to the brain resulting from related insults that follow the primary event including cerebral hypoperfusion and ischemia, increased intracranial pressure (ICP), metabolic dysregulation, hypoxia, and temperature instability.
In the United States, fatality rates for all causes of TBI are lower in metropolitan areas and increase progressively in more rural areas. These differences are significant both for unintentional and assault-related TBIs depicted in (Citation3). Currently, the average fatality rate for TBI from all causes is 22% higher in rural versus urban America. illustrates this disparity (Citation3). These findings strongly suggest that longer transport intervals and limited access to prehospital care may be implicated in higher rates of morbidity and mortality from TBI in these communities.
Figure 1. Fatality rate for all causes of traumatic brain injury (TBI). Source: Brown et al. (Citation3); Used with permission.
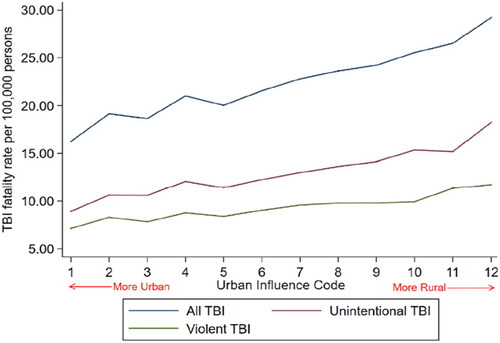
Figure 2. Rural vs. urban traumatic brain injury (TBI) fatality rates. Dark red areas indicate higher age-adjusted fatality rates from TBI. Black-lined areas indicate rural regions. Source: Brown et al. (Citation3). Used with permission.
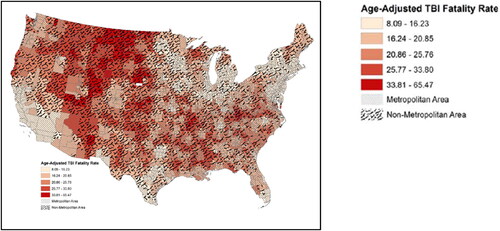
Emergency medical services (EMS) professionals are most often the first health care professionals to assess and treat a patient with TBI. Treatment begins on-scene and continues throughout transport until handoff at a hospital. Worldwide, and including in the US, first-responding EMS professionals have highly varied system configurations, equipment, training, skill capabilities, and skill proficiencies (Citation4). EMS systems incorporate emergency medical technicians (EMT), advanced emergency medical technicians, paramedics, registered nurses, nurse practitioners, and physicians in prehospital transport care, depending on the anticipated critical condition of the patient and available resources.
Evidence-based guidelines for the prehospital management of TBI have been in the literature since the 2000s, when the Brain Trauma Foundation published initial evidence-based guidelines on this topic (Citation5,Citation6). Recommendations for diagnosis and treatment were initially graded as “weak” due to the paucity of quality evidence supporting the recommendations. Since initial publication, evidence has grown supporting an outcome benefit of interventions, and most recently the Arizona Excellence in Prehospital Injury Care (EPIC) initiative documented a benefit in patients with severe TBI when guidelines are followed (Citation7). Unfortunately, despite the ready availability of guidelines, a recent study documents a wide variability of practice in 32 U.S. state protocols reviewed, demonstrating a failure to provide best practice in some systems, and underscoring the opportunity to improve quality of care provided to TBI patients in many systems (Citation8).
This evidence-based Prehospital Guideline on the Management of Traumatic Brain Injury aims to address key topics in the prehospital management of TBI focused on diagnosis and management of primary and secondary brain injury.
Methods
Expert Workgroup and Topic Refinement
To provide the most up-to-date, evidence-based guidance on the prehospital care of TBI, an expert workgroup was established. Workgroup candidates were identified from the Brain Trauma Foundation (BTF) 2nd Edition Guideline participants; a review of authorship of relevant literature; and recommendation from various academic, medical, and health organizations. Using a Population, Intervention, Comparator, Outcomes, Timing (PICOT) framework, the workgroup specified topics and key clinical questions for inclusion in the current update, pertaining to both adult and pediatric populations, distinguished by three overarching categories: assessment, treatment, and decision-making. A minimum of four participants were assigned to work on each topic initially. Participants finalized the scope of each topic and provided terms for the electronic literature search. In total, 22 members ultimately formed the workgroup, and they were required to declare financial and intellectual conflicts of interest. depicts the key categories and topics included in the following BTF guideline update.
Table 1. Categories and topics included in this update.
Inclusion/Exclusion Criteria
Study inclusion criteria are detailed in Appendix A, Online Supplementary Material and were as follows: human subjects, traumatic brain injury, English language, ≥25 subjects, randomized controlled trials (RCTs), cohort studies, case-control studies, case series, databases, registries.
Exclusion criteria were as follows: wrong independent variable (e.g., the intervention was not specific to the topic), wrong dependent variable (e.g., outcomes were not mortality or morbidity, or did not associate with clinical outcomes), statistics used in the analysis were not appropriate to the research design, variables, and/or sample size, case studies, editorials, comments, and letters.
Literature Search Strategy and Evidence Review
Registration for the review was submitted to PROSPERO, the international prospective registrar for reviews (Citation9). A systematic review was conducted based on methods from the Agency for Healthcare Research and Quality (Citation10) and the National Academy of Medicine (Citation1), with reporting based on the Preferred Reporting Items for Systematic Reviews and Meta-Analyses and Meta-analysis of Observational Studies in Epidemiology (Citation11,Citation12). A doctoral-level research librarian constructed electronic search strategies for each topic from May of 2005 through November 2019. Search strategies included the highest likelihood of capturing most of the targeted literature and used Ovid MEDLINE and Cochrane Data Base for published literature and ClinicalTrials.gov for ongoing/completed trials that had not been published. Results of the electronic searches were supplemented by workgroup recommendations and by reading reference lists of included studies.
Literature from the 2005–2019 search was imported into Covidence software (Veritas Health Innovation Ltd, Melbourne, Victoria, Australia), and duplicate articles were removed. Two assessors independently triaged abstracts (first level selection) and subsequently full-text studies (second-level selection) in accordance with inclusion/exclusion criteria with adjudication for discrepancies, as needed, by a third assessor.
An updated review of evidence was supplemented by the research team at the Icahn School of Medicine at Mount Sinai upon PUBMED search for the period from December 2019 through January 2021 using the search words “prehospital” and “traumatic brain injury.” This abbreviated review identified 124 abstracts of which 16 publications were read in full and four were used to support recommendations as related evidence (Citation13,Citation14).
Selected studies were classified according to which topics they included, with assignment allowed to multiple topics as relevant. In studies with duplicate data (companion publications), the original study or the study reporting more detailed or recent data (with a greater number of patients) was included. A total of 122 studies were included for evaluation following systematic and supplemented review.
Study data extraction included information provided relevant to predetermined topics in relationship with prehospital or emergent care and demographic data. After studies were selected for inclusion, data were abstracted into categories that included study design, year, setting, geographic location, sample size, eligibility criteria, patient characteristics, assessment or treatment characteristics, and results. Information was abstracted that is relevant for assessing applicability, including the characteristics of the population, intervention, and care settings. Data extraction was performed by two reviewers and verified by a third. Outcomes of data abstractions are summarized in the “in-text” tables, although detailed abstractions were shared in spreadsheets among the workgroup for use during the recommendation development process.
Data Synthesis, Quality Assessment, and Classification of Evidence
Predefined criteria were used to assess the quality of individual studies. Quality criteria for assessment and treatment topics are based on criteria developed by the U.S. Preventive Services Task Force, the National Health Service Center for Reviews and Dissemination (U.K.), and the Cochrane Collaboration. Clearly defined templates were developed, and criteria were selected appropriate to the study design. Different criteria were used to evaluate the quality of the evidence in assessment topics versus treatment topics. Examples of these criteria and how these criteria are used to label evidence Class I, II, or III corresponding to low, medium, and high risk of bias are detailed in Appendix B, Online Supplementary Material. Quality assessment was performed by two reviewers independently, with adjudication by a third, until consensus was reached.
The entire team gathered for a 2-day in-person work session, followed by multiple virtual meetings, to discuss the literature base, and to achieve consensus on classification of quality of the body of evidence for each topic and question, and strength of recommendations. The strength of evidence for each topic’s key clinical question was assessed by the workgroup using the standards established by the Agency for Healthcare Research and Quality evidence-based practice methods guidance, including:
Study limitations (low, medium, or high level of study limitations based on study design and the quality/risk of bias of the included studies)
Consistency (consistent or inconsistent findings, or unknown/not applicable)
Directness (direct or indirect evidence)
Precision (precise or imprecise estimates of effect)
The quality of the body evidence was assigned an overall grade of high, moderate, low, or insufficient according to a four-level scale by evaluating and weighing the combined results of the aforementioned domains:
High: very confident that the estimate of effect lies close to the true effect for this outcome; the body of evidence has few or no deficiencies; the findings are stable, for example, another study would not change the conclusions.
Moderate: moderately confident that the estimate of effect lies close to the true effect for this outcome; the body of evidence has some deficiencies; the findings are likely to be stable, but some doubt remains.
Low: limited confidence that the estimate of effect lies close to the true effect for this outcome; the body of evidence has major or numerous deficiencies; additional evidence is needed.
Insufficient: No evidence or very limited evidence; unable to estimate an effect or no confidence in the estimate of effect for this outcome; no evidence is available, or the body of evidence has unacceptable deficiencies, precluding reaching a conclusion.
Factors that may decrease the quality include potential bias, differing findings across studies, the use of indirect evidence, or lack of precision. For example, if two or more Class I studies demonstrate contradictory findings for a particular topic, the overall quality most probably will be low because there is uncertainty about the effect. Similarly, Class I or II studies that provide indirect evidence may only constitute low quality evidence, overall.
Recommendations
Recommendations were categorized in terms of strength and quality of evidence. The strength of the recommendation was derived from the overall quality of the body of evidence used to assess the topic. Ultimately the individual studies were considered in aggregate via meta-analyses and/or through qualitative assessment. Hence, the strengths of recommendations were derived from the quality of the overall body of evidence used to address the topic.
Consistent with methods generated by the Grades of Recommendation, Assessment, Development, and Evaluation (GRADE) working group, recommendations were categorized as either strong or weak, reflecting the degree of confidence that the favorable effects of recommendation adherence outweigh the unfavorable effects. Strong recommendations were derived from high-quality evidence that provide precise estimates of the benefits or unfavorable effects of the topic being assessed. With weak recommendations, there was lack of confidence that the benefits outweigh unfavorable effects, the favorable and unfavorable effects may have been equal, and/or there was uncertainty about the degree of favorable or unfavorable effects.
Recommendation development and adjudication of strength were also informed by indirect evidence and related evidence from studies conducted in other settings or other injury/disease processes, evidence published after the conduct of the literature search and informing the subsequent review, and workgroup consensus.
Assessments
Assessment: Oxygenation, Blood Pressure, and Ventilation
Introduction
Following the primary injury, secondary insults from hypoxia, hypoperfusion, and/or ischemia may occur in the prehospital setting. Hypotension reduces cerebral perfusion pressure to the injured brain and has a profound negative effect on outcome. Even brief periods of hypoxia and hypotension are harmful to the injured brain; together they create a larger effect on outcome than either alone. Prehospital care of the TBI patient aims to optimize brain perfusion while rapidly transporting the patient to a location where he or she can receive definitive care.
Current evidence suggests that the historical treatment thresholds for oxygen saturation and/or blood pressure are likely too low (Citation15). Stronger emphasis on avoiding the threshold “region” rather than focusing exclusively on waiting to treat already established low values is appropriate in the absence of more conclusive evidence, such as in a randomized study. Prehospital professionals should continuously monitor for, anticipate, prevent, and rapidly correct both hypoxia and hypotension in patients with suspected TBI.
Recommendations
Patients with suspected TBI should be carefully monitored in the prehospital setting for hypoxemia (<90% arterial hemoglobin saturation), hypotension (<100 mmHg systolic blood pressure [SBP]), hypertension (150 mmHg SBP or higher), hyperventilation (end tidal CO2 less than 35), and hypo- or hyperthermia. (Strength of Recommendation: Strong)
Optimal pediatric-specific SBPs following TBI should be targeted to the 75th and greater percentile for age. (Strength of Recommendation: Weak)
28 days and younger >70 mmHg
1–12 months > 84 mmHg
1–5 years > 90 mmHg
6 years and older > 100 mmHg
Adults 110 mmHg and above
While no specific data exists for hard cutoff values, optimal adult-specific SBPs following TBI are dependent on a variety of factors and should be targeted to 110 mmHg or greater, as lower values are associated with worse outcomes. Optimal targets may be higher. (Strength of Recommendation: Weak)
Blood oxygen saturation should be continuously measured in the prehospital setting with a pulse oximeter and supplemental oxygen administered to maintain blood oxygen saturation above 90%. (Strength of Recommendation: Strong)
Appropriately sized pediatric oximetry sensors should be used in children. (Strength of Recommendation: Strong)
While no specific data exist for hard cutoff values, optimal oxygen saturation levels following TBI are dependent on a variety of factors and should be targeted to 90% or greater, as lower values are associated with worse outcomes. Optimal targets may be higher. (Strength of Recommendation: Weak)
Systolic and diastolic blood pressure should be measured in the prehospital setting using the most accurate method available and should be measured frequently (every 5–10 min) or monitored continuously if possible. (Strength of Recommendation: Strong)
Appropriately sized pediatric blood pressure cuffs should be used to measure blood pressure in children. In resource-limited settings, where pediatric blood pressure cuffs are unavailable, documentation of mental status, quality of peripheral pulses, and capillary refill time should be monitored continuously as surrogate measures. (Strength of Recommendation: Strong)
Blood pressure cuffs should be matched to patients’ size. (Strength of Recommendation: Strong)
Infants: cuff size 6 x 12 cm
Children: cuff size 9 x 18 cm
Small adult: cuff size 12 x 22 cm
Adult: cuff size 16 x 30 cm
Large adult: cuff size 16 x 36 cm
Ventilation should be assessed in the prehospital setting for all patients with altered level of consciousness with continuous capnography to maintain end tidal CO2 values between 35 and 45 mmHg. (Strength of Recommendation: Strong)
Temperature should be measured in the prehospital setting and efforts should be undertaken to maintain euthermia in the patient equating to temperatures of 98–99 degrees Fahrenheit/36–37 degrees Celsius. (Strength of Recommendation: Weak)
In non-resource-limited settings, appropriately sized equipment to measure oxygenation, blood pressure, and temperature in children and adults should be maintained and available for routine use by trained prehospital health care professionals. (Strength of Recommendation: Strong)
Evaluation of the Evidence: Quality, Applicability, and Summary
Quality of Evidence: Moderate (one Class I, seven Class II; and nineteen Class III studies)
Twenty-seven studies inform this topic; 11 of them are carried over from the 2nd Edition. The evidence in this topic consists of cohort studies that examine the effects of oxygen, blood pressure, and temperature assessments and interventions on mortality and function by looking at associations between physiologic measures and outcomes.
The majority of retrospective studies examine multisite trauma databases, and several prospective studies examine multiple centers. Most studies are U.S.-based, but prehospital studies from Sweden, United Kingdom, Japan, Australia, Malaysia, and Italy are also included. Eight studies enrolled children (younger than 18 years of age) only, while 11 presented data of mixed populations of children and adults.
Most studies (Citation21) included in this 3rd Edition Update report hypotension and associated patient outcomes, including mortality and function. These studies reinforce the association between prehospital hypotension and poorer outcomes including higher mortality, lower survival to hospital, and lower survival to hospital discharge among children and adults with TBI (Citation7,Citation16). While earlier studies use standard thresholds to define hypotension (e.g., <90 mmHg for SBP), recent studies question previous thresholds and suggest there may not be a distinct BP inflection point and that increases in blood pressure from any baseline improve outcomes in both children and adults (Citation7,Citation15). Included pediatric studies report best outcomes for children who maintain SBPs more than the 75th percentile for age (Citation16). Early hypertension following TBI was reviewed, suggesting that extremely high blood pressure (160 mmHg and higher) is also related to higher mortality (Citation17,Citation18).
Measuring and addressing oxygenation and preventing episodes of hypoxia was associated with improved patient outcomes in children and adults (Citation7). Two Class III studies reported that untreated hypoxia was not significantly associated with death or disability in isolation (Citation19,Citation20). However, these studies conflict with two Class III studies reporting that hypoxia in isolation was associated with morbidity and other poor outcome (Citation21,Citation22).
The vast majority of studies reported that prehospital hypoxia and hypotension often occur concomitantly in patients with TBI and that this was associated with worse outcomes than when these insults occur independently. A single retrospective study of 299 children reported that approximately one-third of children are not appropriately monitored for blood pressure and oxygenation in the prehospital setting (Citation19). This systematic review also included a single study examining prehospital temperature variability, reporting that euthermia was associated with better outcomes (Citation23).
Scientific Foundation
Previous editions of the Prehospital TBI Guideline have stressed that both hypoxia and abnormal blood pressure (hypotension or hypertension) are strongly associated with poorer outcomes of both adult and pediatric patients with moderate to severe TBI. New evidence since the previous edition builds upon the understanding of these principles with the goal of rapidly identifying and correcting both hypoxia and blood pressure derangements. The prehospital incidence of these secondary insults in TBI patients is common and recent studies have further emphasized the effects of close and active monitoring of these parameters in the prehospital setting on outcomes.
Hypoxia has been estimated to occur frequently in patients with TBI, with one small Italian study (55 patients) demonstrating 55% of patients transported by an air medical service had recorded oxygen saturation <90% (Citation24). A retrospective registry-based analysis of the San Diego Trauma Registry evaluated 3,420 patients with moderate to severe TBI and concluded that hypoxemia (defined as emergency department [ED] arrival PO2 <110) was associated with decreased survival (OR 0.54, 95%CI 0.42, 0.69 p < 0.0001) (Citation21). Mortality for patients with hypoxia (<92%) was 37% in one prospective cohort study (Citation22). These findings support the importance of continuous, or in the minimum, near continuous pulse oximetry monitoring in the prehospital environment for patients with moderate to severe TBI.
Patients with moderate to severe TBI often require advanced airway management, a procedure that exposes patients to additional secondary insults, particularly in the absence of appropriate hemodynamic, oxygenation, and ventilation monitoring. The importance of close monitoring of oxygen saturation was documented in a study of patients in San Diego with suspected TBI undergoing rapid sequence intubation (RSI) in the prehospital setting (Citation25). Each of the 59 patients was matched to three historical nonintubated control patients. Investigators documented the occurrence, timing, and duration of hypoxic episodes and found that profound hypoxia (SpO2 <70%) and any single desaturation <90% was associated with higher mortality (OR 3.89, 95%CI 1.12–13.52 and 3.86, 95%CI 1.18–12.61, respectively) compared to matched controls. Given these findings, patients with significant TBI requiring intubation should undergo continuous pulse oximetry monitoring to allow for rapid correction of hypoxia.
Hypotension reduces cerebral perfusion pressure to the injured brain and has a profoundly negative effect on outcome for patients with moderate to severe TBI. A prospectively collected dataset from the Traumatic Coma Data Bank demonstrated that prehospital hypotension (defined as a single SBP less than 90 mmHg) and hypoxemia (defined as apnea, cyanosis, or oxygen saturation <90%) in the field were powerful predictors of patient outcome. In particular, a single episode of hypotension was associated with a two-fold increase in mortality in matched cohorts without hypotension (Citation26).
The EPIC study evaluated the effect of implementing prehospital TBI guidelines for patients with moderate to severe TBI across the state of Arizona (Citation15). This large, observational study linked to the Arizona State Trauma Registry and discussed further in the Airway section, evaluated 13,151 patients with prehospital TBI, and concluded that in the pre-implementation cohort, odds of death were higher with prehospital hypotension (OR 2.49, 95%CI 1.87–3.32) and hypoxia (OR 3.00, 95%CI 2.37–3.78). Importantly, the study demonstrated that the combined effect of both hypotension and hypoxia was associated with significant mortality (43.9%) and an adjusted odds ratio for death of 6.1 (95%CI 4.20–8.86).
The poor outcomes in TBI patients associated with single episodes of hypotension have been confirmed in multiple studies including EPIC. However, newer data from the EPIC study suggest that hypotension dose (defined as depth-duration dose of hypotension <90 mmHg integrated over time in minutes) is also associated with increased mortality in patients with TBI, with mortality rates exceeding 50% in patients with markedly prolonged episodes of hypotension (Citation27,Citation28). These findings highlight the importance of frequent blood pressure monitoring in the prehospital environment.
The value of 90 mmHg systolic pressure to delineate the threshold for hypotension arose in more of a statistical than physiologic parameter. In considering the evidence concerning the influence of cerebral perfusion pressure on patient outcome with TBI, it is possible that systolic pressures significantly > 90 mmHg may be beneficial during the prehospital and resuscitation phase of care. Since the writing of the prior update, several studies have addressed the issue of a hypotension threshold for TBI patients. The EPIC study specifically reported that across the blood pressure range of 40 mmHg to 119 mmHg in the prehospital setting, each 10-point increase in SBP was associated with a decrease in the adjusted odds ratio of death by 18.8% (adjusted OR, 0.812; 95%CI 0.748–0.883) (Citation7). Importantly, the study demonstrated that there was no identified threshold or inflection point within the range, and that the historic use of 90 mmHg may be incorrect, as decreased mortality rates were significantly associated with higher values.
Age may play a role in defining the optimal blood pressure threshold after TBI. Investigators from Japan evaluated the Japan Trauma Data Bank for in-hospital mortality for patients with severe TBI in an attempt to identify specific blood pressure thresholds. After studying on-arrival blood pressures in 12,537 patients, the investigators advocated for a threshold modified by age in which patients younger than 61 years are considered hypotensive at a SBP <100 mmHg, whereas older patients are considered hypotensive at a SBP <120 mmHg. The investigators identified a SBP of 110 mmHg as the optimal threshold for hypotension with adjusted odds ratio for mortality on admission of 1.58 (95%CI 1.46–1.76, p < 0.001) (Citation29). In 2021, Shibahashi et al. analyzed 34,175 patients with TBI and reported that SBP < 110 mmHg was significantly associated with in-hospital mortality (Citation14).
Extremes of blood pressure, including hypertension, may have significant negative physiological consequences for patients with TBI. The concept of paroxysmal sympathetic hyperactivity is seen in patients after severe TBI and is associated with elevated catecholamines resulting in hypertension and other negative effects (Citation30). Three studies included in this update reflect the importance of monitoring and awareness of hypertension in the prehospital setting (Citation17,Citation31). A retrospective review 305,503 TBI patients from the National Trauma Data Bank reported that adjusted odds for mortality was 1.33 for prehospital SBP of 160–180 mmHg (95%CI 1.22–1.44, p < 0.001) and 1.97 for prehospital SBP of 190–230 mmHg (95%CI 1.76–2.21, p < 0.001) (Citation17). Similar findings were reported in a German study, which retrospectively evaluated 8,788 patients with TBI from the German Society for Trauma Surgery Registry from 1993 to 2008 (Citation31). The primary variables of interest were prehospital SBP greater than 160 mmHg versus less than 160 mmHg. The investigators reported that in patients with TBI and hypertension (> 160 mmHg) there was significantly higher incidence of mortality compared to normotensive patients (25.3% vs. 13.5%, p < 0.001). Overall, the study reported that prehospital hypertension > 160 mmHg had an odds ratio of 1.9 (95%CI 1.4–1.6) for in-hospital mortality compared to normotensive patients.
The available data regarding TBI underscore the importance of frequent prehospital monitoring (at least every 5 minutes) in order to anticipate, prevent, and rapidly correct hypoxia, hypotension, and hypertension in patients with suspected TBI. This requires the thoughtful and intentional implementation of continuous monitoring practices across the spectrum of prehospital care. Effective prehospital assessment and monitoring of TBI patients also affects outcomes indirectly by allowing for adequate information to ultimately direct treatment and improve survival.
Pediatrics: Scientific Foundation
The deleterious effects of hypotension and hypoxemia seen in adults are also seen in children with severe TBI. High-quality studies demonstrating the importance of these prehospital physiological parameters in children are lacking, however this third edition update includes additional studies that support the role of close monitoring of blood pressure and hypoxia in children with TBI.
The prevalence of hypotension and hypoxia during early care (prehospital and emergency department) of children with TBI is high. One retrospective study of 299 children with moderate to severe TBI presenting to a Level I pediatric trauma center identified that blood pressure and oxygenation were recorded during a portion of early care in 31% and 34% of patients, respectively. Hypotension was documented in 118 children (39%). Lack of attempt to treat hypotension was associated with increased odds of death of 3.4 and patients were 3.7 times more likely to suffer disability compared with children in the cohort that underwent treatment. Of note, untreated hypoxia was not significantly associated with death or disability; however, the combined effect of hypotension and hypoxia was associated with poorer outcomes (Citation4). Further studies have indicated that increasing numbers of hypotensive episodes are associated with increased duration of hospitalization, days in the pediatric intensive care unit, and ventilator days (Citation32).
One small study in the United Kingdom evaluated 39 pediatric patients who were admitted between 2002 and 2015 (Citation33). Patients who were identified to have prehospital hypotension (SBP <70 mmHg) were noted to have higher mean intracranial pressure readings over the first 3 days of hospitalization in the intensive care unit setting. Another retrospective study of 10,473 patients from the National Trauma Data Bank addressed the question of specific blood pressure thresholds and association with mortality in children with isolated severe TBI. Admission SBP <75th percentile was associated with higher risk of in-hospital mortality for isolated severe TBI across all age subgroups (Citation16). Of note, the SBP targets used in this study were higher compared with traditional definitions outlined by the American College of Surgeons, suggesting that blood pressure goals for children with severe TBI may be higher than previously thought (Citation16).
The Pediatric Guideline Adherence and Outcomes Study (PEGASUS) was a multisite investigation at five regional pediatric trauma centers examining the effect of timely treatment of hypotension and hypoxia in pediatric patients with TBI. Parameters were defined using Brain Trauma Foundation guidelines: hypotension was defined as SBP less than 70 + 2 (age in years), hypoxia was defined as PaO2 <60 mmHg or oxygen saturation <90%. In this study hypotension that occurred in 26% (60/234) of cases during early care (prehospital or emergency department locations) and was associated with significantly higher in-hospital mortality (23.3% vs. 8.6% p = 0.01). Given that this study was performed after arrival to the hospital, extrapolation of these findings to the prehospital setting may be challenging. However, the authors concluded that timely treatment of hypotension (within 30 minutes) with intravenous fluids, blood product resuscitation, or vasopressors was associated with reduced in-hospital mortality (aRR 0.46%; 95%CI 0.24, 0.90). Hypoxia occurred in 17% of cases (41/236) and all patients in the study were noted to receive early treatment (Citation34).
Updates from the Previous Guideline
This update included evidence from 16 new studies with a moderate quality of the body of existing evidence and lending to improved strength of recommendations and new recommendations. Specific parameters of blood pressure and blood pressure cuff size for pediatric and adult patients with TBI have been added. A focus on ventilation monitoring and measures represents new recommendations following the incorporation of updated evidence. A weak recommendation regarding temperature monitoring and management was newly added. Finally, acknowledgement of resource limitations and recommendations for oxygenation, blood pressure, ventilation, and temperature monitoring in these settings was newly added.
Future Investigations
Examination of the effect of a single hypoxic event versus sustained hypoxia on TBI patient outcomes
Examination on the role of single episodes versus sustained hypotension among TBI patient outcomes
Identification of the optimal ETCO2 in patients with TBI who are either intubated or not
Examination of hypotension and hypoxia in the prehospital phase of care for children with TBI
Assessment: Glasgow Coma Scale and Other Assessment Scales
Introduction
The Glasgow Coma Scale (GCS) and Pediatric GCS (P-GCS) scores are the most widely used clinical measures of the level of consciousness following TBI. Teasdale and Jennett developed the GCS in 1974 describing three independent responses: eye opening, motor response, and verbal response (Citation35). The GCS permits a repetitive and moderately reliable standardized method of reporting and recording ongoing neurologic evaluations even when performed by a variety of health care professionals.
Of the three GCS components, the motor response carries the most similar level of prognostic information compared to the complete score. Authors have recognized limitations in accuracy and inter-rater reliability in GCS assessment, particularly in the prehospital scores. Multiple other scoring systems have been devised in efforts to simplify and improve reliability in assessment and to improve prediction of outcomes in TBI, including the simplified motor score (SMS) and simplified verbal score (SVS) systems.
The GCS score is affected by pre- and post-traumatic factors that may impair neurologic response. Hypoxia and/or hypotension are common complications in trauma patients that may negatively affect GCS scoring and require immediate treatment. Therefore, a patient’s airway, breathing, and circulation should be assessed and stabilized first, prior to measuring the GCS or P-GCS. Prehospital measurements of the total GCS score, inclusive of the P-GCS, are a fundamental component of assessing severity of TBI and allocating trauma resources.
Prehospital GCS scores may vary from ED-assessed GCS scores for a variety of reasons including a patient’s clinical improvement or decompensation following medical stabilization prior to ED arrival. Reversible conditions such as hypoglycemia and sedative or opioid overdose are identified and treated immediately and may affect accurate GCS scoring. Preverbal children have a modified GCS score applied, the P-GCS as outlined in . In the 1998 publication APLS—The Pediatric Emergency Medicine Course, The American College of Emergency Physicians and the American Academy of Pediatrics agreed that, for children under the age of 2 years, the modified GCS appropriately assigns a full verbal score (Citation5) for crying after stimulation (Citation36). This P-GCS has been validated in a large prospective cohort study with comparable accuracy for determining the presence of clinically important TBI in a group of preverbal patients below age 2 years (Citation37).
Table 2. Comparison of Pediatric GCS with GCS.
Recommendations
The adult protocol for standard GCS measurement should be followed in children over 2 years of age. In pre-verbal children, the P-GCS should be employed. (Strength of Recommendation: Strong)
The GCS score should be reported every 30 minutes in the prehospital setting and whenever there is a change in mental status to identify improvement or deterioration over time. Confounders to the GCS such as seizure and post-ictal phase, ingestions and drug overdose, and medications administered in the prehospital setting that affect GCS score should be documented. (Strength of Recommendation: Weak)
The GCS must be obtained through interaction with the patient (i.e., by giving verbal directions or, for patients unable to follow commands, by applying a painful stimulus such as nail bed pressure or axillary pinch). (Strength of Recommendation: Strong)
The GCS should be measured after airway, breathing, and circulation are assessed, after a clear airway is established, and after necessary ventilatory or circulatory resuscitation has been performed. (Strength of Recommendation: Strong)
The GCS should be measured prior to administering sedative or paralytic agents when possible and when not delaying airway stabilization, or after these drugs have been metabolized as they may obscure correct scoring. (Strength of Recommendation: Strong)
The GCS should be measured by prehospital professionals who are appropriately trained in how to administer the GCS to both adults and children. (Strength of Recommendation: Strong)
The GCS of the prehospital patient, including any changes in score, should be communicated to receiving facilities during all communications and upon arrival. (Strength of Recommendation: Strong)
Prehospital assessment of neurologic status using the SMS, or the isolated motor component of the GCS, may provide similar diagnostic and prognostic utility to the complete GCS in adults and may be used in trauma systems organized to incorporate these measures. (Strength of Recommendation: Weak)
Evaluation of the Evidence: Quality, Applicability, and Summary
Quality of Evidence: Moderate (1 Class II study, 17 Class III studies, and 2 systematic reviews of high and low quality)
This topical update included 20 studies; 19 added for this update and one continued from the 2nd Edition Guideline. These studies include two systematic reviews, and 18 individual studies. The evidence on this topic addresses the utility of the GCS in informing triage and treatment. Studies focus on whether GCS scores can identify patients with severe TBI or whether scores are associated with mortality or the need for treatments and interventions such as neurosurgery or intubation. A smaller number of studies have assessed properties of the GCS related to its accuracy when used by different health care professionals or when different training is provided.
The majority of included studies are retrospective and analyze multisite trauma registry databases. Most studies are U.S.-based, however prehospital studies from the United Kingdom, Taiwan, Iran, Germany, and Israel are also included. One study enrolled children (younger than 18 years of age) only, while another presented data of mixed populations of children and adults. The applicability of the evidence is moderate.
The prehospital GCS score, including any change in GCS from the field to hospital arrival, has important implications in TBI for prognosis, management, and destination decision-making. GCS scores are a key component of the prehospital assessment of any patient with suspected TBI. Several studies confirmed a moderate degree of inter- and intra-rater reliability in scoring the GCS, including GCS scores that prehospital EMS professionals obtain.
Scientific Foundation
Several included studies compared prehospital GCS scores and ED GCS scores for prognosis. In all studies, improvement was seen following stabilizing efforts in the field by EMS. Changes in GCS during prehospital treatment are common, and ED GCS may predict functional outcomes better than scene GCS, but the effect was inconsistent across studies. Scoring systems that include GCS, including the revised trauma score (RTS) and the trauma and injury severity score (TRISS), also were predictive of outcomes. Limitations in these studies include retrospective observational designs and missing data. Overall, these data support prehospital measurement of GCS with repeated assessments over time to determine severity of injury and allocation of resources.
Winkler et al. found that ED GCS scores were higher than on-scene scores for all categories of final neurologic outcome, and scene GCS scores were not predictive of eventual outcome (Citation38). For patients with favorable neurologic outcomes, mean GCS improved from 4.3 to 8.8, whereas for unfavorable outcomes scores mean GCS improved from 4.4 to 5.7.
A study of a large trauma registry in Europe restricted to patients with brain abbreviated injury score (AIS) 3 or greater, compared multiple permutations of the GCS both on scene and on arrival with the outcome of in-hospital mortality (Citation39). Median admission total GCS scores were higher than scene scores, and admission scores in their model resulted in higher area under the receiver operating characteristic curve (AUC) than scene scores. As in other included studies, the motor component of GCS had the best accuracy of all sub-scores, with similar accuracy to the overall GCS. Limitations of this study include high rates of missing data, particularly for scene GCS scores, which required imputation for final model creation.
Davis et al. evaluated a cohort of patients with severe TBI and GCS scores of 3 through 8 undergoing prehospital intubation by paramedics using RSI (Citation40). In this study, inter-rater agreement was extremely good between paramedic and ED physician scoring based on data derived from EMS reports, but prognostic accuracy of scene GCS for survival had AUC 0.63, with similar poor overall accuracy for prediction of patient ICU length of stay, Injury Severity Score (ISS), and AIS scores.
In another study, Davis et al. evaluated field and arrival GCS scores and TRISS scores in a large retrospective sample of patients with AIS 3 or greater (Citation41). Field and arrival GCS scores were strongly correlated. AUC for the GCS alone in this cohort was 0.84 for both prehospital and arrival GCS for prediction of mortality, with an optimized threshold value of 5 for prehospital GCS and 6 for arrival GCS. TRISS values in both settings were highly accurate for prediction of survival to hospital discharge in both intubated and nonintubated patients.
Najafi et al. assessed multiple acuity scores, including the RTS, ISS, National Early Warning Score (NEWS), Shock Index (SI), Modified Shock Index (MSI), and the TRISS for accurate prediction of short term 24-hour mortality in a prospective cohort of 185 patients (Citation42). All scores were collected both on scene and on hospital arrival. Stepwise modeling found that prehospital NEWS and in-hospital RTS were best predictive of outcomes.
This body of research also examined the relationship between GCS and pupillary changes in the prognostication of TBI outcome. Combining pupillary size and reactivity with GCS yielded incrementally improved accuracy for prognostication of mortality and functional outcomes across these studies. The combination of poor GCS and grossly abnormal pupillary measures was not found to be universally predictive of adverse functional outcomes, however, suggesting that their presence alone should not preclude continued therapy.
Sadaka et al. followed patients with GCS scores of 3 on presentation to the ED to evaluate Glasgow Outcome Score (GOS) at 6 months and mortality at 14 days (Citation43). At 6 months follow-up, 14.5% of patients had favorable GOS of 4–5, which was accurately predicted by the Corticosteroid Randomization after Significant Head Injury (CRASH) prognosis calculator. Mortality at 14 days was 66%, which was lower than CRASH-predicted mortality of 81%. Patients with favorable GOS had lower Acute Physiology and Chronic Health Evaluation (APACHE) IV scores, less frequently had bilateral fixed pupils, and had lower ICP burden. Favorable GOS occurred in 6.9% of patients in this study with GCS 3 and bilateral fixed pupils, suggesting that this combination is not universally predictive of bad outcome.
Hoffman et al. found in a large retrospective analysis of German trauma patients that GCS predicted mortality with AUC 0.808, but the best predictive ability came when using the GCS combined score and pupil size and reactivity, yielding AUC 0.830 (Citation44). Both pupillary assessments were correlated to each other, and a similar AUC of 0.827 was found with GCS and reactivity alone. For patients with fixed and dilated pupils, 8.0% had favorable outcomes with a GOS 4 or better.
Two studies compared GCS scores in patients stratified by age for accuracy of determining severity of TBI. Both studies found that elderly patients had higher injury severity than younger patients at the same GCS level. These data suggest that triage systems based on GCS should account for age differences, but the retrospective nature of these trials limits this data.
Rau et al. used AIS as a surrogate measure for TBI to compare GCS in elderly (≥65 years) vs. non-elderly patients in a large single-center study in Taiwan (Citation45). Across the same AIS score category, elderly patients had higher GCS scores than younger patients. Caterino et al. compared outcomes of mortality, TBI, neurosurgical intervention, and emergency intubation in a statewide registry, stratifying into a group of elder patients aged ≥ 70 years and adults aged 16–70 years (Citation46). A cutoff point of 14 for elder patients and 13 for adult patients resulted in similar values for sensitivity and specificity between groups.
The largest group of studies, including four observational samples, one meta-analysis, and one systematic review, compared prognostic accuracy of GCS with other scoring systems for mortality and morbidity outcomes. Consistent outcomes included good accuracy of the complete GCS for prognostication, with the motor component being the most predictive aspect. The SMS had similar accuracy to both complete GCS and the motor component of GCS in several studies. No alternate scoring system demonstrated superior accuracy to the complete GCS across studies. Possible benefits of the alternate scoring systems regarding simplicity, accuracy, and reproducibility are suggested but were not robustly assessed in these studies.
Thompson et al. evaluated predictive accuracy of the SMS in comparison to the full GCS within a large urban trauma registry (Citation47). Outcomes included emergent intubation, clinically meaningful brain injury (skull fracture or basilar skull fracture with corresponding evidence of brain laceration, hemorrhage, or contusion; cerebral laceration or contusion; subarachnoid hemorrhage; subdural hemorrhage or epidural hematoma; and other unspecified intracerebral hemorrhage after trauma), neurosurgical intervention (craniotomy, ventriculostomy, intracerebral pressure monitoring, or any other operative cranial procedure), and in-hospital mortality. Absolute values for AUC for the GCS were moderate for all outcomes at 0.70, 0.66, 0.70, and 0.82, respectively. SMS accuracy was lower for all outcomes as compared to the full GCS but differed by a maximal 0.08 points and was described as similar by the authors.
Caterino and Raubenolt used a statewide trauma registry to compare observational data of the SMS and the full GCS for outcomes including in-hospital mortality, neurosurgical intervention, and emergency intubation (Citation48). Their findings showed similar test characteristics and AUC predictive accuracy for SMS and GCS, with identical findings for the comparison of SMS with isolated motor component of the GCS. Mortality was predicted by SMS with AUC 0.82, and by GCS with AUC 0.85, and for the other outcomes AUC did not vary between outcomes by more than 6% between these two scoring systems. The authors suggest that the simpler SMS measuring system may improve accuracy and reduce inter-rater reliability issues in prehospital GCS assessment.
The GCS components and the SMS were further examined by Haukoos et al. in a secondary analysis of a large single-center trauma registry for outcomes of intubation, brain injury, neurosurgical intervention, and mortality (Citation49). In this study, AUC for the GCS was highest in comparison to any single component or to the SMS, with all AUC within 0.06 for all outcomes. For the outcome of mortality, accuracy by AUC was 0.92 for GCS and 0.89 for SMS. Inter-rater agreement in scores was not assessed in this study.
Gill et al. used data from a trauma registry over a 12-year period to compare the GCS, SMS, SVS, and the GCS component scores in accuracy for outcomes of intubation, brain injury, neurosurgical intervention, and mortality (Citation50). In this study, GCS had highest accuracy as measured by AUC for all measures, although the confidence interval for SMS accuracy for mortality (0.86 − 0.89) included the GCS AUC point estimate of 0.89. For the other three outcome measures, the GCS AUC either was superior or was at the upper end of the confidence interval for all other measures’ accuracy. Inter-rater reliability was unable to be assessed in this study.
Meta-analysis of several studies of SMS and GCS for prediction of four outcomes (intubation, clinically important brain injury, neurosurgical intervention, and mortality) was performed by Singh et al. with similar conclusions based on the review of five of the studies mentioned above (Citation51). In this meta-analysis of over 100,000 patients with TBI, pooled AUC for GCS was slightly higher than SMS for prediction of all the above outcomes, but with difference between scoring systems less than 0.04. The authors note that heterogeneity in the assessment of outcomes and the method of scoring subjects may lead to bias, and that the clinical significance of the difference in AUC is debatable.
A recent large systematic review of studies on GCS in TBI by Chou et al. has similar key findings to the above study interpretations (Citation52). In a synthesis of their included studies, the authors conclude that total GCS score has slightly higher accuracy compared to the isolated motor component of GCS for prediction of adverse outcomes. Studies on inter-rater reliability have low level of evidence for improved agreement on motor score compared to complete GCS, and these ratings may be improved using training tools. Prehospital and ED GCS scores showed generally good agreement, although changing patient status may be responsible for variations in these measures noted in some studies.
To evaluate the association of GCS with intracranial hemorrhage in a large blunt trauma population, Becker et al. used a large trauma database from Israel (Citation53). In this study, which excluded patients with GCS scores over 123, 42% of hemodynamically unstable patients (SBP <90 mmHg) with blunt trauma were found to have severe injuries but no TBI. The authors were not able to further characterize the severity of many patients’ TBI, but overall show lower GCS presentations regardless of TBI presence in the setting of hemodynamic instability.
Efforts are underway looking for improved assessment tools to aid in prehospital clinical decision-making and assist in prognosis. Gang et al., working from the Korean nationwide trauma database on severe TBI, have developed a scoring system using the GCS, hypotension, hypoxia, and age (Citation54). They reported an excellent correlation between this score and mortality. However, as this study was done in a single country with a unique trauma system, further study is needed to validate its external application.
Pediatrics
Scientific Foundation
A GCS score of 12–15 reflects the presence of higher integrative brain function. These higher functions may be difficult to assess in young children due to central nervous system immaturity. Maturation of the central nervous system is a continuum from intrauterine development to adolescence. Therefore, especially in young children, the GCS should reflect the expected normal verbal and motor responses for developmental stage. The GCS in its standard form is not applicable to infants and preverbal children. As stated earlier, the 1998 APLS—The Pediatric Emergency Medicine Course supports a modified GCS (Pediatric Glasgow Coma Scale) that assigns a full verbal score (Citation5) for spontaneous cooing in preverbal children.
Nesiama et al. evaluated the relationship between prehospital GCS and arrival GCS in a pediatric population aged 5–18 years (Citation55). In this group, similar to studies performed in adults, a strong agreement existed between prehospital and arrival GCS scores (Cohen’s kappa 0.69). Mean prehospital scores were 0.43 points below mean ED GCS scores, but no difference in median scores was present. Both scores were predictive of functional outcomes as measured by GOS and Disability Rating Scale scores at hospital discharge.
Emami et al. evaluated a large retrospective cohort of German patients with severe TBI including 8.9% aged ≤ 15 years (Citation56). In their study, they found lower mortality in children compared to adults both overall and when restricting to those with GCS 3 and bilateral fixed pupils (80.9% vs. 85% for the latter group). Results were possibly related to higher rates of cardiopulmonary resuscitation for pediatric patients in comparison to adults. Multivariable logistic regression analysis including age, GCS components, pupillary findings, and vital signs showed that lack of motor response, bilateral fixed pupils, and dilated pupils were each associated with higher risk of mortality in the combined cohort. Functional outcomes were better for pediatric patients compared to adults in this study, and the authors suggested that patients ≤15 years of age may benefit from early and aggressive interventions based on their results.
Updates from the Previous Guideline
This update included evidence from 18 new studies improving the moderate quality of the body of existing evidence and lending to greater strength of recommendations. Modified recommendations include a minimum time frame of GCS reassessment in children and adults of 30 minutes. New recommendations include documentation of GCS confounders and communication of the GCS score routinely to receiving hospitals. Finally, a weak recommendation allows for the incorporation of simplified GCS scores focusing on motor component or other factors when preferred by regional trauma systems.
Future Investigations
Identification of factors in prehospital care influencing changes in the GCS score between field and ED assessments, and prognostication of morbidity and mortality
Identification of associations/correlations of communication of GCS score with a receiving facility and improved treatment or outcomes
Examination of the effect of central nervous system depressants on the field measurement of the GCS and its predictive value
Determination of whether alternate or simpler scoring systems than the complete GCS led to improved reliability of scoring over time
Study of interventions, such as training or educational programs, that improve the reliability of GCS scoring
Assessment of the relationship between prehospital physical exam findings, such as pupillary exam, and improved patient triage with identification of critical interventions
Study of strategies to improve prehospital documentation of the GCS
Assessment: Pupil Examination
Introduction
Pupil size, symmetry, and reactivity are affected by many different neuroanatomical pathways and are supported by the literature as integral to clinical decision making, acute management, and long-term prognosis of TBI. Abnormalities of pupillary response or asymmetry are associated with impending neurological deterioration or poor neurologic outcomes. The GCS and pupillary reactivity are prognostic factors in TBI recovery, and in combination can be used to predict 6-month mortality in patients with moderate-to-severe TBI (Citation44,Citation56,Citation57). The associations between pupillary reactivity and GCS are discussed in the previous section. Metabolic, pharmacologic, or toxic etiologies can also lead to pupillary abnormalities, and thus a contextually appropriate and thorough examination is necessary, addressing differential and co-existing diagnoses.
Pupillary response can be an early marker for changes in the patient’s neurologic status in the setting of TBI and should be monitored and reassessed. New changes or anisocoria (unequal pupils with greater than 1 mm of difference) may indicate an increase in ICP that would require intervention, or progression of a mass lesion resulting in trans-tentorial herniation possibly requiring emergent evacuation. Technologies, such as infrared pupilometry, may improve inter-rater variability and allow for more consistent evaluation across the spectrum of prehospital and hospital care, though this has yet to be shown to be of practical benefit.
The bilateral pupillary exam consists of assessment of pupil size, symmetry, and reaction to light. The light reflex depends on a properly functioning lens, retina, optic nerve, brain stem, and oculomotor nerve (cranial nerve III). The direct pupil response assesses unilateral function of the oculomotor nerve; the consensual response assesses the function of the contralateral oculomotor nerve. Absence or asymmetry of these reflexes may indicate a herniation syndrome or ischemia of the brainstem. The pupil exam may be affected by trauma-related factors such as globe rupture and hyphema and non-trauma related factors including use of prescription or illicit drugs, past surgeries, and the lighting under which the exam is performed, malformations and genetic conditions, and the presence of ocular prostheses.
Recommendations
Pupils should be assessed in the prehospital setting after the patient has been resuscitated and stabilized, with the examination recorded and relayed to the receiving facility, for use in diagnosis, treatment, and prognosis. (Strength of Recommendation: Strong)
When assessing pupils, the following should be examined for and documented: (Strength of Recommendation: Strong)
Evidence of orbital and ocular trauma
Comparison of left and right pupillary findings. Clinically significant asymmetry is defined as > 1 mm difference in diameter
Presence of unilateral or bilateral dilated pupil(s)
Presence of fixed and dilated pupil(s). A fixed pupil is defined as <1 mm response to bright light
Confounders to pupil exam
Evaluation of the evidence: Quality, Applicability, and Summary
Quality of Evidence: Moderate to Low (0 Class I, 2 Class II, 10 Class III).
This topical update included 13 studies; eight added for this update and five continued from the 2nd Edition Guideline. The included studies address two questions: 1) What are the diagnostic and prognostic utilities of pupillary examination in the field, and 2) What is the prognosis for “bilateral, fixed and dilated” pupils and how should this affect treatment decisions? Though all studies are Class III except two, the findings are consistent, and the large size of the datasets establish the overall quality of the body of evidence as moderate.
One fair quality study (Citation58), and five poor quality studies—four in adult populations (Citation44,Citation56,Citation57) and one with pediatric patients (Citation59)—contributed data about the diagnostic or prognostic utility of prehospital pupillary examination. In a prehospital study by Sobuwa et al., logistic regression modeling was done on 121 severe TBI patients; pupil reactivity was found to be an independent predictor of outcome, i.e., having bilateral reactive pupils increased the odds of a good outcome by 341% (Citation60). From a large registry in Germany including 24,115 cases, Hoffman et al. found pupil reactivity together with the motor component of the GCS to be the best predictors of mortality from TBI (Citation44). These investigators also reported that the TBI patients with reactive, equal, nondilated pupils before resuscitation had the lowest mortality rates. Taken together, both the adult and pediatric studies demonstrate a strong association between pupillary abnormalities and outcomes.
In 2000, McCabe and Donahue evaluated 30 pediatric patients diagnosed with shaken baby syndrome and found 100% mortality for the eight patients with bilateral, fixed pupils on arrival to the trauma center (Citation59). Sadaka et al. performed a retrospective review of 62 patients with severe TBI and GCS scores of 3; 7% had bilateral fixed pupils yet achieved good outcomes at 6 months (Citation43). Two more recent studies of 185 patients examined the prognostic utility of pupil exams demonstrating bilateral fixed and dilated pupils (Citation61,Citation62). One retrospective study reported a 94% mortality rate (87 of 93) (Citation61). A prospective observational study reported an 88% mortality rate (81 of 92) (Citation63). Conversely, these studies demonstrate that approximately one in 10–20 patients with fixed, dilated pupils in the field have good functional outcomes thus underscoring the importance of early aggressive management of patients with severe TBI.
Scientific Foundation
The pupillomotor nuclei is located in the dorsal midbrain, and the oculomotor nerve exits from the midbrain to the superior orbital fissure. Compression of the midbrain and oculomotor nerve from transtentorial herniation can be identified by anisocoria and decreased light reflex. Physiologic anisocoria, defined as no more than 1 mm difference between pupil size and normal reactivity, is present in up to 20% of the population, depending on lighting conditions (Citation64). Pupillary asymmetry less than 1 mm is normal and has no pathologic significance. In one study of 310 healthy volunteers with 2,432 paired measurements using advanced technology, asymmetry of pupillary size greater than 0.5 mm was measured in less than 1% of subjects and was rarely seen in TBI patients unless the ICP exceeded 20 mmHg (Citation63).
Chesnut et al. retrospectively analyzed data from 608 patients with severe TBI to assess the reliability of pupillary asymmetry in predicting the presence and location of intracranial mass lesions (Citation65). Pupillary asymmetry had a positive predictive value of 30%, with almost 80% of those patients having lesions contralateral to the pupil finding. Anisocoria had a sensitivity of 40% and a specificity of 67%; even when the pupils were different by more than 3 mm there was only a 43% positive predictive value. Thus, a single measurement of pupillary asymmetry is neither a sensitive nor specific finding in either identifying or localizing an intracranial mass lesion.
Mamelak et al. studied 672 TBI patients aged 0–80+ years. They found that age was the most important predictor of outcome, followed by initial motor exam and then by pupil response, demonstrating some correlation between pupillary response and outcome (Citation66).
Pupillary changes may be associated with ischemia of the brainstem and may also be monitored as prognostic indicators of functional recovery in moderate-to-severe TBI (Citation67). Increased ICP resulting in uncal herniation compresses cranial nerve III, resulting in a reduction of parasympathetic tone to the pupillary constrictor fibers, producing a dilated pupil with decreased reactivity. Destruction of the nerve also results in a dilated and fixed pupil. Bilaterally dilated and fixed pupils are consistent with direct brain stem injury, as well as with marked elevation of ICP. Metabolic or cardiovascular disturbances including hypoxemia, hypotension, and hypothermia are associated with dilated pupils and abnormal reactivity, making it necessary to resuscitate and stabilize the patient before assessing pupillary function (Citation67).
Direct trauma to cranial nerve III in the absence of significant intracranial injury or herniation may result in pupillary abnormalities usually associated with ocular motor deficits. Asymmetric pupillary constriction can make the contralateral pupil appear dilated.
Pupillary function may be an indicator of TBI after trauma, however it is neither a specific indicator of the anatomy nor of the injury severity. In spite of this, there is moderate to good quality evidence to support the value of assessing pupillary functions in the field as both a guide to immediate medical decision making, and as a long-term prognosticator.
Updates from the Previous Guideline
This update included evidence from eight new studies informing a low-moderate quality of the body of existing evidence and lending to improved strength of recommendations. Though overall recommendation contents do not change, recommendations have been modified to be more specific and to include documentation of confounders to exam and communication with the receiving hospital.
Future Investigations
Examination of medical device accuracy and prognostication of prehospital pupil examination
Determination of whether repeat prehospital pupillary examination is associated with improved prognostic capability
Treatment
Treatment: Airway, Oxygenation, and Ventilation
Introduction
Airway management, oxygenation, and ventilation in the suspected TBI patient are critical treatment strategies in the prehospital setting to maximize good outcomes. Key considerations include the proactive prevention of secondary insults such as hypoxia resulting in reduced levels of tissue oxygen, and the identification of patients who may benefit from endotracheal intubation. Hypoxemia is a strong predictor of outcome in the patient with acute TBI. Prehospital airway management studies relate to assessment, equipment and technique, and performance skills. These examine whether intubation skills can be taught and safely maintained by prehospital professionals with minimal complications. Corollaries to this question include recognition of an esophageal intubation in the field and the degree to which prehospital professionals manage difficult or failed airways. Additionally, studies examine medication adjuncts to prehospital airway intubation, methods of oversight, monitoring, and quality improvement processes.
Prehospital airway management is dependent upon the accurate identification of patients who need intubation and minimizing secondary brain insult by avoiding peri-intubation hypoxia, hypotension, and hyperventilation. Ultimately, these studies aim to ascertain the conditions in which field intubation results in improved neurologic outcomes and decreased mortality.
Recommendations
All patients with suspected severe TBI should be placed on continuous oxygen supplementation via nasal cannula or face mask in the prehospital setting in order to minimize secondary insults related to hypoxia. (Strength of Evidence: Strong)
Hypoxemia (oxygen saturation [SpO2] < 90%) should be monitored using continuous pulse oximetry and corrected immediately upon identification by ensuring appropriate airway positioning and administering continuous, supplemental oxygen. (Strength of Evidence: Strong)
If signs of hypoxia persist (central cyanosis and/or hypoxemia on pulse oximetry) despite increasing the flow and concentration of continuous supplemental oxygen, the following stepwise strategies should be undertaken with reevaluation of oxygen saturation and respiratory effort following each strategy: (Strength of Evidence: Strong)
airway re-positioning, with attention to possible cervical spine injury
positive pressure ventilation as with bag-valve-mask ventilation in conjunction with appropriate airway adjuncts (e.g., oropharyngeal airway), and/or
supraglottic airway or endotracheal intubation by a trained health care professional.
An airway should be established, by the most appropriate means available, in patients who have signs of severe TBI, and the inability to maintain an adequate airway, or if hypoxemia is not corrected by supplemental oxygen. (Strength of Evidence: Strong)
EMS systems implementing endotracheal intubation protocols including the use of RSI protocols should confirm endotracheal tube placement in the trachea by the presence of bilateral breath sounds on auscultation, and ETCO2 detection and/or capnography. Intubated patients in the prehospital setting require continuously monitored oxygenation and ETCO2, and frequent blood pressure monitoring. (Strength of Evidence: Strong)
Patients requiring respiratory support with positive pressure ventilation should be ventilated with normal breathing rates (approximately 10 breaths per minute with ETCO2 35–45 mmHg), and hyperventilation (ETCO2 <35 mmHg) should be avoided. Ventilatory adjuncts such as pressure-controlled bags, ventilation-rate timers, ETCO2 monitoring, and ventilators should be used to support appropriate ventilation and minimize the risk of secondary insults by avoiding hypo- and hyperventilation. (Strength of Evidence: Strong)
Evaluation of the Evidence: Quality, Applicability, and Summary
Quality of Evidence: Moderate (3 Class I, 5 Class II, 12 Class III).
This topical update included 20 studies; 14 added for this update and two continued from the 2nd Edition Guideline. These studies include two systematic reviews (Citation68,Citation69). Of 27 studies originally included in the 2nd Edition, 10 were included in the systematic reviews, and are not summarized in the current evidence tables. An additional twelve studies from the 2nd Edition are descriptive or addressed surrogate outcomes and are not in these tables.
Studies included for this topic addressed five questions: 1) Should, when, how, and for whom should endotracheal intubation be used, 2) Under what conditions should paralytics be used to assist intubation, 3) What sedatives should be used for RSI, 4) What target ranges should be used to manage ventilation, and 5) What are the differences between the management of adult and pediatric airways?
Scientific Foundation
The use of airway management to allow for adequate oxygenation and ventilation is a critical component of the prehospital phase of resuscitation for patients with TBI. Since the 2nd Edition Guidelines were published, multiple additional studies have shed light on the importance of rapid assessment and correction of hypocapnia (secondary to hyperventilation) and hypoxia. A continued area of emphasis and investigation focuses on the role of prehospital intubation and the determination of which TBI patients will benefit from it.
As stated previously, hypoxia has significant and deleterious effects for the patient with TBI. Patients are defined as being hypoxemic by evidence of oxygen saturation <90% and/or central cyanosis (Citation70). While correction of patients who are already hypoxic is critical, this guideline update stresses the importance of prevention of hypoxia in all patients with suspected TBI. In the prehospital setting continuous high-flow oxygen should be administered for all patients with suspected TBI even if maintaining normal oxygenation. Ideally, oxygen saturation readings should be documented every 5 minutes with a goal of maintaining values > 90%.
For patients who do not appropriately respond to correction of oxygenation with supplemental administration via a high flow oxygen source, additional airway maneuvers should be performed, beginning with airway repositioning. If there continues to be persistent hypoxia, bag-valve-mask ventilation should be performed using appropriate airway adjuncts such as oropharyngeal airways. Consideration should also be given to supraglottic devices such as the esophageal tracheal airway or laryngeal mask airways. If there continues to be inadequate oxygenation and ventilation after these interventions, advanced airway maneuvers such as intubation should be performed by an experienced advanced life support professional. Furthermore, patients with severe TBI and depressed mental status (GCS <9) should be immediately considered for definitive advanced airway management. A retrospective review of the Trauma Registry of the German Society for Trauma Surgery evaluated 21,242 patients and concluded that intubated patients in the prehospital setting with GCS <9 had less difference between their actual and predicted mortality compared to nonintubated patients (Citation44).
The role of endotracheal intubation in the prehospital setting for patients with TBI has historically been an area of controversy. Multiple studies of varying quality have shown conflicting outcomes for TBI patients undergoing prehospital intubation. Initial studies, most notably a retrospective analysis of 981 patients with TBI who underwent intubation in both prehospital and ED settings combined, found significant mortality associated with intubation (Citation71). This particular study found the association of emergent intubation in either setting with mortality to have an odds ratio of 14.3 for death (95%CI 9.4–21.9).
Another retrospective study (Class III evidence) evaluating 310 suspected TBI patients with GCS <14 from the Sydney Trauma Center between 2007 and 2013 found that there was no improvement in survival to discharge (73% vs. 70%, p = 0.69) in the prehospital intubation versus ED intubation cohorts respectively (Citation72). A U.S.-based retrospective study examining the National Trauma Data Bank identified 8,139 patients who underwent prehospital intubation for TBI with matched controls who did not undergo intubation and reported longer scene intervals (median 9 vs. 8 minutes p < 0.001), longer transport intervals (median 26 vs. 19 minutes p < 0.001), lower ED GCS values (3.7 vs. 3.9, p = 0.026), and higher in-hospital mortality (31.4 vs. 27.5%, p < 0.001) in those who underwent prehospital intubation (Citation73).
More recent, larger systematic reviews have further informed the discussion on the role of prehospital intubation for patients with TBI, based primarily on collections of retrospective data cohorts. A systematic review of 17 studies up to 2007 did not support benefit from prehospital intubation and mechanical ventilation after TBI (Citation69). While many of the included studies were observational/retrospective, this systematic review was one of the first and largest to address the important question of whether prehospital intubation should be performed for patients with suspected TBI. This systematic review was followed by another searching through 2015, which included a total of 30 studies (24 studies in systematic review and six studies in meta-analysis) (Citation68). The investigators concluded that while the odds ratio for mortality was higher in the prehospital intubation group versus those who underwent no intubation, they did report significantly higher odds of death for prehospital intubation by professionals with limited experience (OR 2.33, 95%CI 1.61–3.38, p < 0.001) compared to professionals with higher levels of experience (OR 0.75, 95%CI 0.52 to 1.08, p = 0.13). While the study concluded that there was insufficient evidence of improved outcomes or mortality following prehospital intubation performed by trained health care professionals on patients with TBI, there were trends of improved overall outcomes. The single randomized trial included in these reviews, by Bernard et al. in 2010, reported an odds ratio of 1.6 for favorable outcome in those intubated in the prehospital setting compared to the in-hospital setting.
One large multicenter trial (Class I evidence) in the United States evaluated prehospital intubation for patients with TBI and reported favorable outcomes in 882 patients (Citation74). The study reported that prehospital intubation was associated with more favorable neurological outcome in patients who were enrolled with severe, moderate-to-severe, or moderate TBI due to blunt trauma with GCS score of 4 to 12. Specifically, favorable neurological outcome was present in 57.3% of patients who underwent prehospital intubation versus 46.0% of patients who did not (p = 0.003). The prehospital intubation group was also associated with lower overall mortality (13.8% vs. 19.5% p = 0.03). This particular study, which adjusted for index GCS, reported 47% lower odds of death in patients who underwent prehospital compared to those who did not (OR 0.53, 95%CI 0.36–0.78). However, in this trial, 80% of the patients who underwent prehospital intubation for TBI were transported by an air medical service, and the investigators were unable to find a significant effect on neurologic outcomes from a prehospital intubation after adjusting for the effect of transport method in a multivariable logistic regression model. The investigators reported that in patients who underwent intubation, odds of dying when transported by ground were higher when adjusted for GCS (OR 2.10, 95%CI 1.40–3.15) than when transported by air, but trended toward improvement as compared to no intubation. Ultimately, investigators concluded that prehospital intubation for TBI patients undergoing air medical transport was associated with favorable outcomes and lower mortality. They reported that prehospital intubation was not associated with increased morbidity or mortality irrespective of transport method or severity of injury.
The evidence in this update highlights the importance of adequate training and maintenance of skills associated with prehospital intubation to ensure safe performance and minimal complications. Such skills include recognition of an esophageal intubation in the field, the degree to which the prehospital professional is able to manage both the difficult or failed airway, and the use of RSI to facilitate first pass success.
The 2nd Edition Guideline included a series of US studies examining intubation success rate and reported an overall improvement in intubation success rate of approximately 85% as compared to 39% in historical controls with GCS <9 and suspected TBI following changes in training and equipment, including the use of RSI for intubation (Citation75). These studies reported an 11% higher mortality rate in the RSI group; 41% compared to 30% for those patients not intubated (OR 1.6; 95%CI 1.1–2.3). The RSI cohort also had a lower incidence of good neurologic outcome; 37% versus 49% (OR 1.6; 95%CI 1.1–2.3). Importantly, these studies did not evaluate for indications of intubation, and of the prospectively enrolled patients, 32% of patients who were intubated did not have TBI. The increased success rates with RSI for prehospital intubation seen in these studies was confirmed in an Australian study; however, no significant improvement in mortality rate or ICU length of stay was associated with this difference (Citation76).
Prior research has demonstrated that hyperventilation and hypocapnia are associated with worse outcomes for patients with TBI, emphasizing the fundamental importance of continuous ETCO2 monitoring with waveform capnography in the prehospital setting. Acutely following TBI, there may be a period of hypoperfusion with a marked reduction in cerebral blood flow by as much as two thirds of normal in approximately half of patients (Citation77). Hyperventilation, described in more detail in the next section with relationship to increased ICP, further decreases cerebral blood flow, potentially leading to cerebral ischemia, and in-hospital studies have demonstrated hyperventilation to compromise cerebral perfusion and worsen outcomes. Inadvertent hyperventilation during prehospital transport occurs frequently and is associated with increased mortality (Citation78). In a U.S. study, 15% of subjects undergoing prehospital RSI had severe hyperventilation (pCO2 < 25) upon hospital arrival as compared to 8% of non-intubated controls. These results emphasize the avoidance of hyperventilation as a mainstay of prehospital TBI resuscitation.
The EPIC study, a large EMS and public health partnership in Arizona involving statewide, multi-system implementation of Brain Trauma Foundation and National Association of EMS Physicians guidelines, used an intention to treat, before/after controlled design for patients with moderate to severe TBI between 2007 to 2015 (Citation7). The implemented guidelines enforced an oxygen saturation threshold of greater than or equal to 90% in all potential patients with TBI by applying continuous, high flow oxygen via non-rebreather facemask. The guidelines recommended pre-oxygenation for all patients to prevent subsequent hypoxia and deterioration. Patients who had inadequate oxygenation or ventilation progressed toward bag-valve-mask ventilation and then intubation when bag-valve-mask was unsuccessful in treating hypoxia. Set ventilatory rates of 10 breaths per minute for adult patients (greater than 15 years of age) with two-finger bag technique supported hyperventilation avoidance. Continuous ETCO2 monitoring was required, with a target value of 40 mmHg and a range of 35–45 mmHg. A total of 21,852 patients met criteria for inclusion in the study (15,228 patients in the pre-implementation phase, and 6,624 patients in the post-implementation phase), of whom 4,014 required intubation.
The key findings from EPIC emphasized that while statewide implementation of prehospital TBI guidelines was not associated with significant improvement in overall survival to hospital discharge, within severe injury cohorts, guideline implementation was associated with improved survival to discharge (Regional Severity Score-Head 3–4: aOR 2.03; 95%CI 1.52–2.72, p < 0.001; Injury Severity Score 16–24: aOR 1.61; 95%CI 1.07–2.48; p = 0.02). Statewide guideline implementation was successful at obtaining a greater likelihood of having an SpO2 of 100%, greater rates of reversal of hypoxemia, lower rates of hyperventilation/hypocapnia in intubated patients, and overall, a lower rate of intubation and higher rate of BVM-only airway management despite increased severity of both brain and overall injury in the post-intervention group.
Updates from the Previous Guideline
This update included evidence from 14 new studies contributing to the moderate quality of the body of existing evidence and lending to improved strength of recommendations advising airway, oxygenation, and ventilation treatments. Updated recommendations advise specific parameters of oxygenation and ventilation, continuous monitoring for hypoxia, and a stepwise approach to addressing airway management. Updated recommendations specify the avoidance of hyperventilation.
Future Investigations
Examine the effects of training programs on prehospital professional airway management proficiency
Compare patient outcomes following prehospital RSI using different sedatives
Identify variability in prehospital airway management among systems and investigate best practices for patient outcomes
Treatment: Fluid Resuscitation
Introduction
For patients with TBI, prehospital fluid resuscitation aims to prevent hypovolemia, hypotension, and resultant secondary injury to the brain. Prehospital hypotension is defined by different parameters in adults and children, and these are discussed in the Assessment section of this guideline. Current evidence suggests that the historical treatment thresholds for blood pressure are likely too low. Stronger emphasis on avoiding the threshold “region” rather than focusing exclusively on waiting to treat already established low values is appropriate in the absence of more conclusive evidence such as in a randomized study.
The objectives of fluid therapy are multiple, with a primary goal to augment cardiac preload and maintain cardiac output, thereby providing needed perfusion and oxygen to the brain without causing hemodilution or secondary blood loss. Depending on the mechanism of injury (blunt or penetrating trauma), the specific choice or volume of fluid delivered may differ and may include crystalloid fluids (isotonic or hypertonic) or colloid fluids (including blood or blood substitutes).
Recommendations
Intravenous fluids should be administered in the prehospital setting to treat hypotension and/or limit hypotension to the shortest duration possible. (Strength of Recommendation: Strong)
Hypotensive patients should be treated with isotonic fluids and/or blood products (if indicated and available) in the prehospital setting. (Strength of Recommendation: Strong)
Hypertonic fluid resuscitation may be administered to patients with GCS scores <8 in whom increased ICP is suspected in the prehospital setting. (Strength of Recommendation: Weak)
Evaluation of the Evidence: Quality, Applicability, and Summary
Quality of Evidence: Moderate (0 Class I studies, 10 Class II studies, 3 Class III studies).
This topical update included 13 studies; six added for this update and seven continued from the 2nd Edition Guideline. These studies include two systematic reviews (Citation79,Citation80), and five individual studies (Citation81–85).
The included studies compare different fluids for use in resuscitation. There are few new studies overall for review, and trials evaluating this area are limited by small sample sizes. Overall, these studies report limited and uncertain differences in patient outcomes with different fluids, despite potential biological differences and changes in biomarkers. This topic is complicated in that management of the polytrauma patient with hypotension may be different than management of the patient with an isolated TBI; in these cases, fluid resuscitation must be tailored to the circumstance.
Scientific Foundation
Hemorrhage following trauma decreases cardiac preload. When compensatory mechanisms are overwhelmed, peripheral perfusion and oxygen delivery decrease. Fluid therapy with blood products is used to replete preload supporting cardiovascular function and peripheral oxygen delivery. This is particularly important in patients with TBI, as decreased cerebral perfusion can increase the extent of primary neurological injury or can worsen neurological outcome. In particular, hypotension has been shown to produce secondary brain injury and worsen outcome (Citation26). In a prospective series of 6,908 adults and 1906 children less than 15 years of age at 41 centers, Luerssen et al. found that hypotension was significantly associated with higher mortality in all age groups, with a greater deleterious effect of hypotension in children as compared to adults (Citation86). Hypotension and its definitions are provided previously in Assessment.
If hypotension does occur, blood pressure and oxygen delivery should be promptly restored to avoid secondary brain injury. Ideally, this should be done in a way that does not cause secondary blood loss or hemodilution. Because the underlying cause of hypotension in these patients is almost always blood and/or fluid losses, intravascular volume repletion is the most effective way of restoring blood pressure. In contrast, data indicate that early restoration of blood pressure in patients with penetrating torso trauma worsens outcome. The relationship between these data and outcome in patients with TBI is unknown.
To date, crystalloid fluid has been used most often to augment cardiac preload, maintain cardiac output, and support peripheral oxygen delivery in trauma patients. Common prehospital resuscitation strategies include the bolus infusion of 1 to 2 liters of crystalloid volume. In children, fluid resuscitation is indicated for clinical signs of decreased perfusion even when an adequate blood pressure reading is obtained. In addition to crystalloid, other options such as hyperoncotic and hypertonic fluids as well as hemoglobin substitutes have been used. While these fluids are appealing in that they offer the potential for quicker infusions and smaller volumes, their benefits in TBI treatment have been inconsistent. In general, lower quality and earlier studies have reported outcome benefits that are not replicated in larger, methodologically superior trials performed later.
In the early 1990s, Vassar et al. published four randomized, double-blind trials comparing various combinations of isotonic fluids, hypertonic saline, and added dextran (Citation84,Citation87,Citation88). The studies reported that hypertonic saline use in the prehospital setting was safe and with potential outcome benefit; dextran did not provide any additional benefit. In another trial comparing hypertonic to normal saline, no differences in outcome were found (Citation86). Hypertonic saline was compared with Ringer’s lactate in 166 patients (32% with severe TBI), and logistic regression analysis showed the hypertonic saline group to have improved survival (Citation84). A third study of 258 patients (10% with severe TBI) compared hypertonic saline with and without dextran, finding that in patients with GCS scores less than 8 or with severe anatomic cerebral damage, survival with either fluid was greater than predicted by TRISS (Citation85). In 1993, Vassar et al. published a multicenter trial of 194 patients, 74% with severe TBI, showing improved survival in patients with GCS scores ≤8 treated with hypertonic saline compared to Ringer’s lactate (Citation87).
Wade et al. published a review of studies containing data for patients with TBI who received hypertonic saline (Citation83). Survival to discharge was 38% for patients treated with hypertonic saline and 27% for those treated with standard therapy (p = 0.08). When logistic regression analysis was performed comparing hypertonic with isotonic fluids, the odds ratio was 1.92 for 24-hour survival and 2.12 for survival to discharge (p = 0.048).
In 2004, Cooper et al. reported a randomized, double-blind prehospital trial of hypertonic saline or standard fluid therapy in 229 patients with severe TBI and hypotension (Citation89). Multitrauma patients were included but patients with comorbid conditions, peripheral edema, or close proximity to the hospital were excluded. Following an initial fluid bolus of 250 mL, patients received standard resuscitation, both in the field and in the hospital. There were no differences in outcomes between the two groups.
In 2011 Tan et al. performed a comprehensive review of the prehospital fluid management in the TBI literature (Citation80). Nine randomized trials and one cohort study were included. The authors concluded that neither hypertonic fluids nor the use of dextran improved functional outcomes. A study by Junger et al. in 2013 compared outcomes in TBI patients resuscitated with 250 ml of normal saline, vs hypertonic saline, vs hypertonic saline plus dextran (Citation81). Though this was not a prehospital study, no difference in outcome was found between the three groups.
A systematic review and meta-analysis of prehospital fluid resuscitation, published in 2017 by Blanchard et al. did not find an outcome benefit of hypertonic saline over isotonic saline; however, this study included all types of trauma patients and not just those with TBI (Citation79). A systematic review and meta-analysis by Bergmans et al. in 2020 also did not find sufficient evidence to demonstrate an outcome benefit of any one specific fluid type used in the prehospital setting (Citation13). No harm was found in the use of hypertonic solutions among these studies and thus the evidence allows for prehospital systems to assess all risks and benefits when choosing the intravenous fluid to administer in TBI patients.
Updates from the Previous Guideline
This update included evidence from six new studies improving the quality of the body of existing evidence and lending to improved strength of recommendations, though recommendation contents do not significantly change.
Future Investigations
Further data on the effect of the prehospital use of hypertonic saline on TBI outcome is needed. Cognitive recovery as a separate endpoint from blood pressure resuscitation needs to be investigated.
Compare the effectiveness of hypertonic solutions as plasma volume expanders in the prehospital patient with suspected TBI.
Treatment: Hyperventilation and Hyperosmolar Therapy for Suspected Increased Intracranial Pressure
Introduction
Early recognition and treatment of increased ICP is fundamental to maximizing improved outcomes in patients with TBI. Classic indicators of increased ICP include Cushing’s triad (hypertension, bradycardia, irregular breathing pattern), GCS <9, posturing or lateralizing findings, progressive neurologic deterioration, and unilateral or bilateral fixed, dilated pupil(s). However, these indicators may not be sufficiently sensitive and may be associated with a delay in definitive treatment (Citation90).
In human physiology, ventilation is the exchange of oxygen and carbon dioxide in the alveoli of the lungs. Hyperventilation, achieved by increasing the respiratory rate, increases the absorption of O2 and elimination of CO2. A lower CO2 is known to vasoconstrict cerebral microvasculature and decrease overall blood volume in the brain. A lower blood volume in the brain results in a lower ICP. Historically, hyperventilation was considered an effective tool to lower ICP in the setting of TBI and potentially prevent brain herniation. It was widely adopted as a preventative and/or prophylactic measure in the prehospital and ED settings when elevated ICP was suspected.
A series of studies published in the early 2000s challenged the traditional management of TBI. These prospective observational studies reported higher mortality rates and a lower incidence of good neurologic outcome in patients who received prehospital RSI as opposed to the group of patients not intubated. Further analysis of the data demonstrated that the RSI group had a higher rate of severe hypocarbia on arrival to the hospital, suggesting that hyperventilation was an important contributor to the increased mortality. These data raise the concern that vasoconstriction induced by hypocapnia (low PaCO2) may lead to ischemia in the microvasculature and accumulation of neurotoxic agents (e.g., lactate and glutamate).
A growing body of evidence from prehospital and in-hospital studies supports that routine hyperventilation is detrimental and worsens outcomes in TBI patients; therefore, recommendation for the prehospital monitoring of ETCO2 has emerged as a fundamental component of TBI management. Patients with ETCO2 monitoring have a lower incidence of severe hyperventilation, and the use of capnography with ventilator adjustments during transport significantly decreases the incidence of hypocapnia upon arrival at the trauma center.
Intracranial hemorrhage results in a mass effect with subsequent elevations in ICP, and prevention of intracranial hemorrhage could reduce the risk of herniation. Prehospital administration of tranexamic acid (TXA) has been postulated as a potential treatment for the prevention of intracranial hemorrhage expansion. A large NIH-funded placebo controlled multicenter clinical trial examining the efficacy of two dosing regimens of TXA initiated in the prehospital setting in patient with moderate to severe TBI revealed no differences in 28-day mortality, progression of intracranial hemorrhage, or 6-month GOS-E scores (Citation91). TXA is not a direct treatment in the setting of increased ICP and therefore falls outside the scope of this section.
Recommendations
Hyperventilation should be avoided in the prehospital care of children and adults with TBI in the absence of signs of active cerebral herniation. Signs of active cerebral herniation include Cushing’s triad (hypertension, bradycardia, irregular breathing pattern), GCS <9, posturing or lateralizing findings, progressive neurologic deterioration, and unilateral or bilateral fixed, dilated pupil(s). (Strength of Recommendation: Strong)
Ventilation strategies should target eucapnia (i.e., ETCO2 of 35–40) and avoid hypocapnia and be monitored using capnography. (Strength of Recommendation: Strong)
When used to address signs of active and imminent herniation, hyperventilation should target an ETCO2 of 30–35 using capnography. (Strength of Recommendation: Strong)
Hyperosmolar therapy (mannitol and hypertonic saline) should not be administered for the prophylactic treatment of suspected elevated ICP, with or without signs of cerebral herniation, in the prehospital setting. (Strength of Recommendation: Weak)
Prehospital administration of TXA therapy is not generally and widely indicated for the prophylactic treatment of suspected ICH or elevated ICP. However, decisions by health care systems may vary and further evidence may support more general use. (Strength of Recommendation: Strong)
Evaluation of the Evidence: Quality, Applicability, and Summary
Quality of Evidence: Moderate (1 Class I, 2 Class II, 7 Class xcIII).
This topical update included 10 studies; four added for this update and six continued from the 2nd Edition Guideline. Hyperventilation in the acute setting reduces ICP by causing cerebral vasoconstriction with a subsequent reduction in cerebral blood flow. Hyperventilation has been shown to reduce ICP in many patients with cerebral edema (Citation92). Class II data indicate that patients chronically hyperventilated in the in-hospital setting have worse outcomes at 3 and 6 months but equivalent outcomes at one year (Citation93). Two new studies and one study from the 2nd Edition evaluate prehospital hyperventilation as an intervention in severe TBI. A number of studies from the 2nd Edition contain data collected in-hospital rather than in prehospital settings with descriptive data.
The most common causes of increased ICP secondary to TBI include expanding mass lesions (intracranial hemorrhage) and cerebral edema resulting from inflammatory responses, and a complex array of issues leading to a loss of vascular and cellular integrity. Hyperosmolar agents target the reduction of extracellular fluid by increasing the osmolar gradient and drawing fluid into the vasculature while improving viscosity. Though of theoretical benefit, no high-quality studies were identified directly addressing the use of hyperosmolar therapy for the acute prehospital management of increased ICP, and this is discussed further in Fluid Resuscitation.
Scientific Foundation
Hyperventilation
Davis et al. in 2005 examined the association of prehospital hyperventilation and negative outcomes for severe TBI patients (Citation94) and reported a relationship between lower PaCO2 upon arrival to the ED and poorer outcomes (Citation78,Citation95). Dumont et al. in 2010 reported on the occurrence of prehospital hyperventilation in patients who were not actually herniating; both hyper- and hypoventilation increased the risk of mortality (Citation96). Caulfield et al. in 2009 also reported negative associations with hyperventilation and both mortality and neurological function (measured by the GOS) (Citation97). Spaite et al. in 2019 demonstrated improvements in survival-to-hospital in patients with severe TBI by implementing Brain Trauma Foundation guidelines, inclusive of avoiding hyper- and hypoventilation (Citation7). This body of evidence supports that unintentional hyperventilation may be common in the prehospital setting for a variety of factors (Citation98). While capnography is critical to monitor ETCO2, it does not assure the avoidance of inadvertent hyperventilation (Citation99).
Although a preponderance of evidence fails to show a benefit in early TBI, in a subset of patients with objective evidence of active and imminent herniation, the risks and benefits of hyperventilation are less clear. Observable clinical signs suggesting possible cerebral herniation in the prehospital setting include dilated or unreactive pupil(s), asymmetric pupils, posturing, or progressive neurologic deterioration (decrease in the GCS score of more than two points from the patient’s prior best score), but these signs have moderate to low predictive values (Citation100).
The optimal prehospital approach to a TBI patient maintains adequate ventilation with the PaCO2 in the range of 35–45 mmHg. Care must be taken not to inadvertently hyperventilate patients during transport. Monitoring PaCO2 is highly recommended as a confirmatory measure of appropriate ventilation. Hypoxia should be treated by increasing the oxygen tension of inspired air as opposed to increasing the ventilatory rate. In a patient with clinical signs of active herniation, transient hyperventilation to a PaO2 level of 30–35 mmHg may be considered, but only in settings where surgical decompression or other interventions will not be rapidly available.
Hyperosmolar agents
Mannitol and hypertonic saline are effective and commonly used hyperosmolar agents for temporarily reducing ICP. However, mannitol’s effect on outcome has not been demonstrated in a high-quality trial of prehospital use. Schwartz et al. conducted a study comparing mannitol to pentobarbital that failed to demonstrate the superiority of pentobarbital and that demonstrated better outcomes and maintenance of cerebral perfusion pressure in the mannitol group (Citation101). Hypertonic saline, a low-volume resuscitation fluid, is an alternative to mannitol as a brain-targeted hyperosmotic therapy. Multiple animal studies and several human studies demonstrated that bolus-dosed hypertonic saline reduces ICP in a structured environment such as the operating room or ICU where ICP monitoring is present. However, there is a minimal amount of evidence of hypertonic saline’s benefit as an acute intervention to improve morbidity and mortality.
Normal saline (0.9% NaCl), delivered via intravenous bolus or infusion, is isotonic to human cells and, in theory, causes very little osmolar shift. Any solution that increases the tonicity of the extracellular space is considered hypertonic. There is no standard convention for the concentrations of solutions that are hypertonic, other than how they are supplied. Concentrations of 3%, 7.2%, 7.5%, 10%, and 23.4% are clinically available and used for a variety of indications in a variety of settings. There currently is no definitive evidence on the optimum concentration or delivery mechanism (bolus versus continuous infusion) for reduction of ICP (Citation89,Citation102–105) making comparison of these agents difficult since studies do not use the same concentrations or protocols. No study has reported on hypertonic saline’s effect on clinical signs of herniation such as pupillary changes or posturing similar to the data available for mannitol.
When using hypertonic saline for ICP management in the in-hospital setting, the target serum sodium level is often around 155–160 mEq/L. This elevated serum sodium is postulated to stabilize ICP and reduce the therapeutic intensity required to prevent elevated ICP (Citation105). However, such a therapeutic target is not feasible in the prehospital environment.
One Class II study evaluated the effect of prehospital hypertonic saline on neurological outcome (Citation89). Hypertonic saline did not demonstrate any advantage over normal saline on neurological outcome when given as a prehospital resuscitation fluid. Similarly, a Class III study comparing 1.6% saline to lactated Ringer’s solution found no difference in outcomes between groups, but baseline differences and other factors limit the interpretation of findings (Citation106).
Relevant studies (one Class II and one Class III) failed to demonstrate improvements on outcome with prehospital administration of hyperosmolar fluids. Therefore, the current literature does not support the use of mannitol or hypertonic saline for the prophylactic ICP reduction in the prehospital setting.
Tranexamic Acid
Though not a treatment per se of increased ICP, TXA has been studied as a preventive prehospital intervention against intracranial bleeding, which may contribute to increased ICP. The CRASH-3 trial suggested an outcome benefit in the prehospital use of TXA in TBI patients with mild to moderate injury, but not severe (Citation107). The Prehospital TXA for TBI trial was a randomized double blinded multicenter phase II trial performed in 20 trauma centers across the United States and Canada. There was no statistical benefit found with the administration of TXA (Citation91). Likewise, the BRAIN-PROTECT study found no overall outcome benefit (in fact, it reported increased mortality in the TXA treated group) (Citation108). This was a multicenter, prospectively collected observational study that involved 680 patients treated with TXA. Based on these studies, TXA may or may not be beneficial in the prehospital management of severe TBI (Citation91).
Pediatrics: Additional Considerations
As stated in the Guidelines for the Acute Medical Management of Severe Traumatic Brain Injury in Infants, Children, and Adolescents, the effect of hyperventilation on long-term outcome has not been addressed in pediatric TBI and this guideline’s recommendations were based upon indirect evidence from adult studies.
Updates from the Previous Guideline
This update included evidence from 10 new studies improving the moderate quality of the body of existing evidence and lending to improved strength of recommendations. Current modifications discuss TXA but do not recommend its use in prehospital settings. They warn against hyperventilation and recommend monitoring using capnography.
Future Investigations
Examine the accuracy and reliability of indicators for increased ICP in the prehospital setting
Identify and determine the superiority of ventilation control devices that improve outcome after moderate to severe TBI
Determine whether transient hyperventilation in response to suspected, imminent herniation results in poorer/improved outcomes as compared to no transient hyperventilation
Determine whether prehospital hyperosmolar therapy in the setting of suspected, imminent cerebral herniation improves/worsens outcomes
Identify indications for prehospital hypertonic resuscitation in children
Explore the role for tranexamic acid in the prehospital management of TBI patients with suspected, imminent cerebral herniation
Diagnosis
Diagnosis: Decision-Making Within the EMS System: Dispatch and Destination, On-Scene, and Transportation
Introduction
Prehospital health care professionals treating patients with TBI make critical decisions regarding where and how to transport them. This is determined by balancing the patient’s need for the clinical resources of a trauma center with the most appropriate mode of and time to transport to the designated location. While the severity of injury dictates the type of receiving facility, determination of injury severity by prehospital health care professionals is a time-sensitive decision based on measures from the limited diagnostic equipment available in the field and rapid clinical assessment. In the unstable patient, prehospital professionals must often choose between transport to the nearest hospital versus extended transport intervals to a higher-level trauma center. Prehospital professionals also help determine the mode of transport: ground or air. These important decisions have the potential for affecting morbidity and mortality for TBI patients.
A U.S. study reported that the overall risk of death for severely injured adults, after adjusting for differences in severity, decreased by 25% when care was provided at a Level I-II trauma center (Citation109). The Field Triage Decision Scheme developed by the American College of Surgeons and updated in collaboration with the Centers for Disease Control and Prevention is intended to assist prehospital professionals in identifying the general trauma patient with severe injury (Citation110). This decision scheme uses a four-step process to determine trauma patient needs. Recent research suggests that, when strictly applied, these guidelines have limitations. Based on a study by MacKenzie et al., under-triage, defined as not being treated at a Level I or II facility when severely injured, is associated with increased mortality (Citation109). However, over-triage, defined as bypassing closer hospitals to reach a higher-level trauma center when a patient does not need those services, has a cost for those patients and their families who live far from a trauma center, increases safety risks to the patient and crew, and creates a community cost when an ambulance is taken out of service for an extended transport to a specialty hospital. Ultimately, lives and resources are saved when access to an organized trauma system is readily available for the optimally matched patient requiring that level of care.
Recommendations
All regions should have an organized trauma care system with comprehensive documentation of each encounter including time, assessment, and care provided. (Strength of Recommendation: Strong)
EMS should establish specific protocols directing destination decisions for patients with suspected traumatic brain injury (TBI). (Strength of Recommendation: Strong)
Pediatric patients with suspected TBI should be treated in pediatric trauma centers, or in adult trauma centers with added qualifications to treat children in preference to Level I or II adult trauma centers without added qualifications for pediatric treatment. (Strength of Recommendation: Strong)
Patients with suspected moderate-severe TBI should be transported directly to facilities with immediately available computed tomography (CT) neuroimaging capabilities, prompt neurosurgical care, and the ability to monitor ICP and treat intracranial hypertension. (Strength of Recommendation: Strong)
While direct transport to a trauma center is preferable for most patients, in the event that this transport is not possible, stabilization at a non-trauma center with subsequent transfer within an established trauma system may occur. (Strength of Recommendation: Weak)
In a metropolitan area, pediatric patients with severe TBI should be transported directly to pediatric trauma centers if available. (Strength of Recommendation: Strong)
The mode of transport should be selected to minimize the interval to needed definitive interventions for the patient with TBI. (Strength of Recommendation: Strong)
Evaluation of the Evidence: Quality, Applicability, and Summary
Quality of Evidence: Moderate (1 Class I study, 8 Class II studies, 30 Class III studies).
This topical update included 39 studies; 28 added for this update and 11 continued from the 2nd Edition Guideline. These studies include prospective and retrospective cohort studies of varying sizes. While some use national or statewide data from large registries, others report the experience of a single center.
The evidence addresses five subtopics:
Regionalization of trauma care;
Direct versus indirect transport to a trauma center;
Level of trauma center (type of treatment);
Mode of transport; and
Time to treatment.
Within each of these subtopics, the results were found to be inconsistent. Differences in reports are likely due to varying environments, regional standards, or specific practices.
Scientific Foundation
Prehospital health care professional recognition of TBI and subsequent management are important factors in patient outcome. A host of decisions and execution points are made in the prehospital setting by EMS telecommunicators and first responders, and at the transition point to receiving hospitals. These generally occur in a stepwise fashion as described in .
Figure 3. Stepwise execution of prehospital decision-making in the care of a patient with head injury.
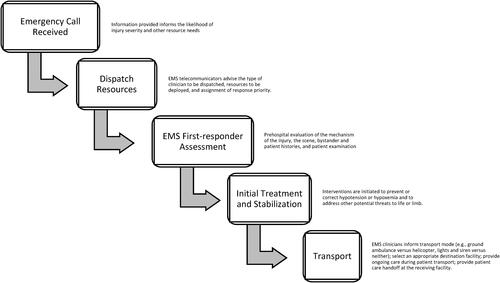
Regionalization of Trauma Care
Regionalization of care for time-sensitive medical conditions has demonstrated outcome benefits in ST-elevation myocardial infarction, stroke, and trauma. Regionalization of care is an essential component of an efficient and effective trauma system and, in most regions, transport destination decisions are made in the context of a formalized trauma system.
Excellent care for the TBI patient requires timely transportation to a neurotrauma-capable receiving facility; scientific evidence has demonstrated a decrease in morbidity and mortality among these patients. An early report of preventable deaths in the United States compared non-TBI and TBI deaths before and after instituting a regional trauma care system (Citation111). After a trauma system was put in place, preventable deaths decreased for both non-TBI patients (20% to 1%, p < 0.005) and patients with TBI (5% to 0.7%, p < 0.10). Another U.S.-based retrospective study compared TBI outcomes before and after the implementation of a trauma system reporting an odds ratio of 0.80 for mortality after system implementation (Citation112). An older 1995 study by Hunt et al. evaluated the benefit of a regionalized system of trauma care for mortality in patients with severe TBI (Citation113). While mortality did fall, this change was not significant. However, time to emergency decompression and mortality among those requiring emergent decompression decreased significantly. Another study compared the pre-and-post outcomes of injured patients in a rural hospital before implementing American College of Surgeons Committee on Trauma Guidelines for Level II trauma center verification (Citation114). Survival for all subjects with calculated probability of survival of 25% was 13% before and 30% after meeting trauma center criteria. For patients with TBI, survival was 15.4% before and 32% after meeting the criteria.
A retrospective observational study examining patients in the New York State Trauma Registry over a 3-year period with one or more physiologic criteria by the field triage criteria found that head-injured patients transported to regional trauma centers had significantly lower mortality as compared to those transported to nontrauma centers (OR 0.67; 95%CI 0.53–0.85) (Citation115). There was no identifiable effect with other injury types, although the study was not powered to find these differences.
This sub-topical update includes one new study (Class II evidence) evaluating the effect of regionalized trauma care on the long-term outcomes of head injured patients (Citation116). A total of 3,496 patients with severe TBI were identified from the registry of a single trauma system, 1,359 before regionalization and 2,137 after. In-hospital mortality decreased significantly from 19% to 14% (p < 0.0001) following regionalization. Six-month mortality decreased from 24% to 20% (p = 0.004). Functional independence measure scores did not change significantly after regionalization despite an increase in survival. The authors note that this suggests that the increase in survival did not come at the expense of poorer neurologic outcomes, but also did not improve them.
Direct versus Indirect Transport to a Trauma Center
The highest priority of on-scene prehospital treatment is stabilization of immediate life threats and prevention of secondary injury. However, EMS personnel must also quickly determine which receiving hospital is the most appropriate for primary transfer. Recent literature about general trauma patients suggests that the patient outcomes improve when prehospital care, triage, and admission to designated trauma centers are coordinated within regional trauma systems (Citation109). However, this is balanced with the benefits of rapid stabilization and subsequent transfer, as well as stress to the system when over-triage occurs.
The available literature has not shown any definitive benefit of direct transport to a trauma center as compared to transport to a non-trauma center with subsequent transfer for adult patients with TBI. In a study using the 2012 National Emergency Department Sample, Fakhry et al. found that almost 20% of all ED visits for severe TBI resulted in admission to a non-Level I or II trauma center, representing 45% of all ED visits for severe TBI presenting to these centers (Citation117). Patients with isolated TBI transported directly to Level I or II trauma centers were more likely to be admitted (89% vs. 45%) and had significantly higher predicted mortality (11.3% vs. 6.8%). For those patients with severe TBI transported to lower-level trauma centers, there was no significant difference in probability of death for patients admitted as compared to those transferred to Level I or II trauma centers. Kuimi et al. aimed to assess the effect of access to an integrated trauma system on in-hospital mortality and length of stay for major trauma (Citation118). A total of 22,749 patient records were reviewed, of which 89% were taken directly to trauma centers. Neither in-hospital mortality nor length of stay were significantly different for patients with TBI transported directly to trauma centers as compared to those who were not. The authors note that in this established trauma system, those patients in need of the services of a trauma center likely were transported there initially, explaining the perceived lack of difference between the two groups. Gale et al. examined TBI occurring in a rural setting over a 6-year study period (Citation119). Comparing the 44% of patients receiving initial evaluation and stabilization at referral centers to those transported directly to Level I trauma centers, the latter were significantly younger and had significantly higher ISS and lower GCS scores. After regression analysis, only increased age and increased ISS contributed significantly to mortality, with neither distance to a trauma center nor time to transfer contributing to mortality.
In a systematic review of 19 studies from 1988 through 2012, Pickering et al. also found no significant outcome difference for patients directly transported directly to trauma centers (Citation120). Of the three studies that adjusted for age and injury severity in head trauma, one favored immediate transport to a specialty center while the other two favored transfer, though the finding was not significant. Pooled data showed no significant difference in mortality between groups, though heterogeneity was high. Unadjusted analysis of 10 studies also did not demonstrate any significant difference between groups. In a more recent study, Nishijima et al. evaluated 350 older patients (age > 54) with TBI, 73% of whom were transported directly to Level I or II trauma centers (Citation121). No functional outcome difference was found for those transported directly to trauma centers compared with those who were not.
In 2021, Sewalt et al. published their findings from the prospective European CENTER-TBI study, a multicenter study involving 22 European countries (Citation122). The aim of their analysis was to assess outcome differences between moderate and severe TBI patients who were directly admitted to neuroscience centers as compared to those who were secondarily transferred. Of 1,347 moderate and severe TBI patients, 195 were managed after secondary referral. After adjusting for case mix, no effect on functional outcome or survival was determined. The study was limited by its observational design, loss of data, and risk of missing cases who died before transfer. However, the study underscores the importance of meticulous management of blood pressure and oxygenation during the early stages of moderate and severe TBI management.
A single Class I study did not find any difference in 30-day mortality between TBI patients brought directly to specialty neurosurgical centers as compared to those transported to nonacute hospitals and subsequently transferred (Citation123). This cluster-randomized study allocated ambulance stations to transport TBI patients to nonacute hospitals or bypass for neurosurgical specialty centers. Unfortunately, enrollment was poor and lower than the target of 700 patients, with 293 patients identified over 12 months, and only 24% of the enrolled patients having evidence of TBI on CT imaging. The authors note a substantial over-triage rate with no associated difference in mortality, suggesting that transport directly to a specialty center might not be necessary. However, poor enrollment and low rates of protocol adherence limit the study’s generalizability.
Two Class III studies suggest some benefit of direct transport. In a retrospective registry study, Prabhakaran et al. examined prospectively collected registry data over 2 years for patients with TBI transported directly (64.7%), or subsequently transferred (35.3%), to a single Level I trauma center and admitted to a trauma or neurosurgical service (Citation124). While there was no difference in overall mortality, for patients with GCS <9 time to neurosurgical intervention was significantly longer and mortality was significantly higher for those initially transported to non-Level I trauma centers. A study by Johnson et al. evaluating a pediatric population likewise suggests benefit of direct transport to a trauma center (Citation125). In a prospective, nonrandomized comparison of mortality among admitted patients transported directly to a pediatric Level I trauma center compared to those subsequently transferred, the overall mortality rate was significantly greater in the indirect transport group (4.7%) than the direct transport group (1.9%). However, the trauma score was significantly higher in the direct transport group, indicating that patients in the latter group were less stable physiologically, and constituting a baseline difference between groups. The authors suggest that this is better viewed as an outcome than a baseline difference; that the physiological deterioration in the delayed transfer group occurred as a function of delays in appropriate treatment due to the transfer. This suggests that in this metropolitan area, pediatric patients with severe TBI are more likely to survive if transported immediately to pediatric trauma centers than if transported first to another type of center and subsequently transferred.
Level of Trauma Center (Type of Treatment)
The American College of Surgeons has established standards for trauma centers, defining the level of trauma center based not only on available resources but also on educational and research commitments. While both Level I and Level II trauma centers have the resources to appropriately care for trauma patients, including patients with TBI, previous studies have demonstrated improved patient outcomes when patients are treated at Level I trauma centers. Further studies focusing on TBI patients have sought to evaluate patient outcomes when they are transported directly to Level I trauma centers from the field, or subsequently transferred after initial management. Current evidence does not definitively support transport directly to Level I trauma centers over Level II trauma centers, though some studies of mixed quality may suggest benefit of direct transport to Level I centers McConnell et al. evaluated patients initially presenting to rural trauma centers and subsequently transferred to Level I or Level II trauma centers (Citation126). Patients transferred to Level I trauma centers had a 10% absolute reduction in 30-day post-discharge mortality as compared to patients transferred to Level II trauma centers. This finding was also demonstrated in a retrospective review of data from the National Trauma Data Bank showing a significantly higher mortality rate in TBI patients initially transported to Level II trauma centers, with an adjusted odds ratio of 1.57 (95%CI 1.41–1.75) (Citation127). Furthermore, the complication rate of patients treated in Level I trauma centers was also significantly lower.
However, other studies suggest that there may be little to no benefit of direct transport to Level I trauma centers as compared to transport to Level II centers. Cornwell et al. evaluated the change in trauma patient mortality using a before-and-after design to determine the effect of achieving a Level 1 trauma center designation at a single university-affiliated trauma center (Citation128). The time for patients to get to the operating room and intensive care unit decreased significantly after implementation of a full-time trauma service. While there was a trend toward improved in-hospital mortality amongst patients with severe TBI, this finding was not statistically significant. Lombardo et al. examined TBI patients admitted to a neurosurgical ICU as compared to a trauma ICU or a general medical/surgical ICU (Citation129). Survival for patients with isolated TBI admitted to a neurosurgical ICU did not differ significantly from patients admitted to a trauma ICU, suggesting that a Level II trauma center with generalized trauma care may be adequate when compared to the sub-specialization available at a Level I trauma center. Alkhoury and Courtney used the National Trauma Data Bank to examine isolated TBI presenting to Level I or Level II trauma centers (Citation130). Outcomes including mortality and complications were non-inferior for patients transported to Level II trauma centers.
While evidence for adolescent patients does not definitively support transport directly to pediatric trauma centers over adult trauma centers, evidence does support the direct transport of pediatric patients with TBI to trauma centers with pediatric capabilities. In a 2017 study evaluating outcome differences for patients aged 15 to 17 years with TBI, Gross et al. found no significant difference in outcomes for patients transported to adult versus pediatric trauma centers (Citation131). This finding persisted when controlling for Level I versus Level II trauma centers. However, Potoka et al. found that pediatric patients aged 0 through 16 years with severe TBI are more likely to survive if treated in pediatric trauma centers (Citation132). Furthermore, the authors note that pediatric patients with severe TBI who require neurosurgical procedures have a lower chance of survival in adult Level II trauma centers as compared to other types of trauma centers. Bardes et al. used National Trauma Data Bank data to compare pediatric patients aged <14 years with isolated TBI transported to pediatric trauma centers to patients transported to adult Level I trauma centers (Citation133). Mortality for patients treated at adult trauma centers was significantly higher than for those treated in pediatric trauma centers (OR 1.55, p = 0.01). There was no significant difference between those treated in pediatric trauma centers compared to those treated in mixed adult/pediatric trauma centers, suggesting that the mortality benefit comes from the expertise afforded by the pediatric subspecialists available at these centers.
Mode of Transport
Determining the most appropriate mode of transport includes decision-making regarding ground versus air transportation and, when considering ground transport via ambulance, whether to deploy emergency lights and sirens. These decisions hinge on whether patient care needs require expedited transport intervals and higher levels of care on scene, including the presence of an advanced health care professional with further critical care training such as a nurse practitioner or physician. While the existing evidence suggests a benefit of helicopter transport over ground transport for patients with severe TBI, some of this benefit is likely attributable to the additional capabilities provided by air medical transport services.
Bekelis et al. studied TBI patients transported by ground (ambulance) or air (helicopter) to Level I or Level II trauma centers within the National Trauma Data Bank (Citation134). While patients transported by helicopter likely represented a sicker patient population as indicated by a lower average GCS, higher average ISS, and higher overall mortality (12% vs. 7.8%), propensity score matching was performed to reduce potential confounding between the two groups, with excellent quality of matching. After matching, TBI patients transported to Level I trauma centers by helicopter had significantly better survival as compared to those transported by ambulance (OR 1.88, 95%CI 1.74–2.03). There were similar results in TBI patients transported to Level II trauma centers (OR 1.73, 95%CI 1.55–1.94). Aiolfi et al. also used this databank to examine patients with severe TBI transported by air or ground EMS. Despite significantly higher injury scores, helicopter transport was independently associated with increased survival (OR 0.55, 95%CI 0.47–0.67) (Citation135). Sun et al. identified a subset of skiers and snowboarders in the same databank with isolated TBI (Citation136). Patients transported by air were found to have increased in-hospital survival as compared to patients transported by ground (OR 8.58, 95%CI 1.09–67.64).
In an early study evaluating the effect of helicopter versus ground ambulance transport for TBI patients, Baxt and Moody found a 9% reduction in mortality for TBI patients transported by helicopter. However, it should be noted that, in this EMS system, the helicopter was staffed by a physician and a nurse while the ground ambulance was staffed by a paramedic (Citation137).
Davis et al. retrospectively assessed 10,314 patients from the trauma registry of a single county in California with head AIS scores of 3 or more (Citation94). They found that those who were transported by helicopter had better odds of survival (1.90; 95% confidence interval 1.6 to 2.25) as compared to ground transport after controlling for potential confounding variables. Air transport was particularly beneficial for patients with more severe injuries, indicated by lower GCS. In an Italian study examining TBI patients transported by air or ground EMS, helicopter-transported patients had significantly longer scene intervals, but a shorter interval to intervention, and significantly lower mortality (21% vs. 25%, p < 0.05) (Citation138). Both groups were staffed by nurses and physicians, although the helicopter group had advanced airway capabilities and patients in this group were intubated more often and received more intravenous fluids.
In contrast to these studies favoring helicopter transport for patients with TBI, Bulger et al. did not find benefit to helicopter transport for severe TBI as compared to ground EMS (Citation139). This retrospective analysis of prospective data from two randomized controlled trials evaluated adult patients with severe TBI transported by ground or air to Level I or II trauma centers, excluding patients with evidence of hemorrhagic shock. Patients transported by air had longer scene intervals, more substantial injury burden, and overall higher predicted mortality. When controlling for differences in injury mechanism, injury severity, and initial physiology, there was no significant difference in 24-hour or 28-day survival for ground versus air ambulance transport. In an Italian study by Di Bartolomeo et al., outcomes following TBI were examined for association with ground ambulance transport including nursing care as compared to helicopter transport including physician care (Citation140). Similarly, this study found no significant difference in patient outcome between the transport protocols. An older registry study also found no outcome difference in patients with TBI transported to designated trauma centers by air as compared to ground (Citation141), and a 2012 study by de Jongh et al. found a non-significant trend toward higher in-hospital mortality for patients with TBI transported by helicopter (Citation142). In this latter study, TBI patients were matched to control patients transported by ground. The risk of in-hospital mortality for the helicopter group was not significantly higher than that of the ground transport group (OR 1.3, 95%CI 0.6–2.7). When controlling for scene interval, the risk of in-hospital mortality decreased but was not significantly different between groups (OR 0.8, 95%CI 0.4–2.0).
Data specifically addressing helicopter transport for pediatric patients with TBI are limited. Missios and Bekelis used multivariate analysis with propensity matched scoring to examine the association of helicopter transport and survival in comparison to transport by ground EMS among subjects from the National Trauma Data Bank (Citation143). Helicopter transport was associated with an increased likelihood of in-hospital survival for patients transported to both Level I (OR 1.77, 95%CI 1.25–2.52) and Level II (OR 2.56, 95%CI 1.28–5.11) trauma centers. As with studies in adult populations, the subjects transported by helicopter had more severe injuries and higher unadjusted mortality. Heschl et al. also studied children with TBI who were transported by helicopter or ground EMS, again finding favorable neurologic outcomes in the subset of helicopter-transported patients with TBI and major trauma (66% vs. 17%, p = 0.06) (Citation144). However, in addition to a small sample size, all patients in the helicopter group were intubated in the field as compared to none of the patients in the ground transport group, making the results difficult to interpret. Given the unclear benefit of helicopter transport in pediatric TBI, there is some concern that helicopter transport may be overutilized in certain circumstances. Elswick et al. used data on pediatric patients referred to a single Level I trauma center to determine factors associated with appropriate helicopter EMS transport (Citation145). The study algorithmically concluded that air transport use is best reserved for pediatric patients with TBI demonstrating GCS 3–8, mass effect or herniation on CT imaging, cerebral edema, epidural hematoma, or open depressed skull fractures; although, this cohort did not include transports from the scene of injury. However, time to specialist intervention must be considered in all decisions regarding ground or air transport.
Time to Treatment
The effects of prehospital intervals, including delayed transport, on the outcomes for patients with TBI remain unclear. General prehospital professionals training supports the teaching that all suspected TBI patients should be rapidly transported to be able to undergo surgery within the first hour after injury if needed, and clinical experience confirms that there is an important subset of patients in whom rapid treatment dramatically improves outcomes. However, the reviewed prehospital literature does not definitively support any association between prehospital interval and patient outcomes.
Acute subdural hematomas in severe TBI patients are associated with 90% mortality if evaluated more than 4 hours after injury, and 30% mortality if evaluated earlier (Citation128). Hunt et al. reported a 70% decrease in mortality when patients with subdural hematoma are evaluated less than 2 hours after injury (Citation113). These data suggest that decreased prehospital interval, with associated rapid hospital evaluation and treatment, may be beneficial. Wilberger et al. evaluated the effect of the interval from injury to operative care among patients with subdural hematoma, and found no statistically significant difference in overall mortality (Citation146). However, for those patients who were treated within 4 hours there was a 10% absolute reduction in mortality compared to those treated greater than 4 hours. Furthermore, this study only looked at time to operative intervention and did not look at the potential positive effects of other hospital interventions. In the study conducted by Di Bartolomeo et al. described previously, the authors did not identify a difference in patient outcome in patient groups, with an almost 60-minute difference in the interval to arrival at the receiving facility (Citation140). Lokkeberg and Grimes, while controlling for confounding variables including injury severity score, found that among patients with severe blunt TBI, interval to definitive care was not a significant predictor of patient outcome (Citation141).
Additional studies queried the relationship of prehospital interval and outcomes for patients with TBI. In a 2011 study, scene interval of greater than 60 minutes was not associated with poor outcome, although scene intervals for intubated patients were significantly longer (Citation20). Using prehospital vital signs as compared to emergency department vital signs as an indicator of clinical deterioration, Fuller et al. found no association between scene interval and changes in vital signs, and EMS interval had no significant association with clinical deterioration during transport (Citation147). While 25% of patients had vital sign changes during transport, there was no relationship between the magnitude of vital signs changes in the prehospital interval and the length of EMS interval after adjusting for potential confounders. In a 2015 analysis of registry data, adult patients with significant TBI admitted to specialty neurologic centers were evaluated for the effect of EMS interval on 30-day inpatient mortality. The authors did not find any significant association between EMS interval and mortality on regression analysis adjusting for confounders including the use of propensity matching (Citation148). Newgard et al. evaluated patients with TBI to compare out-of-hospital time with 6-month neurologic function (Citation149). Using a secondary analysis of Resuscitations Outcomes Consortium data, the group compared patients with an out-of-hospital interval of less than 60 minutes to those with an out-of-hospital interval greater than 60 minutes. TBI patients with GCS scores of less than 9 and with transport intervals greater than 60 minutes had higher ISS scores, more airway interventions, and more frequent air transport. However, despite a sicker cohort, there were no significant differences in 6-month neurologic outcomes or 28-day mortality. Finally, Meizoso et al. evaluated the association of prehospital interventions with mortality in severely injured trauma patients (Citation150). This group found that in their trauma system, while prehospital interventions were associated with decreased mortality, they were not associated with significantly longer scene intervals. Taken together, these studies suggest that the interventions provided on scene, and not necessarily the time spent on scene, may be the main driver of patient outcomes following TBI.
Two studies included in this update suggest some benefit to more timely transportation to specialty centers. In a retrospective cohort study of patients with traumatic subdural hemorrhage, Tien et al. found that longer prehospital intervals were associated with increased mortality, with an OR of 1.03 for each additional minute on scene (95%CI 1.005–1.05) (Citation151). However, limitations include a small cohort from a single institution with very high mortality (40%). Dinh and colleagues also found an association between scene interval and mortality, with a hazard ratio of 1.002 for each additional minute on scene after controlling for GCS (95%CI 1.001–1.004) (Citation152). However, after adjusting for potential confounders, there was no significant difference between those arriving within 1 hour and those arriving after.
Updates from the Previous Guideline
This update included evidence from 28 new studies improving the quality of the body of existing evidence and lending to improved strength of recommendations, though recommendation contents do not change.
Future Investigations
Determination of the relationship between informational factors and the accuracy of EMS telecommunicators in identifying TBI and dispatching resources
Study of the association of dispatch decisions and TBI patient outcome
Development of pre-arrival instructions for callers requesting emergency to aid to improve patient outcome
Identification of effects of prehospital assessment, treatment, transport, and destination decisions on the outcome of the patient with severe TBI
Comparison of TBI patient outcomes among those treated by organized EMS systems within trauma systems versus EMS systems without trauma systems. Such study should evaluate the various levels of EMS professional training and hospital preparation and include patients with different degrees of severity of injury.
Association of transport interval with TBI patient outcome in the setting of special circumstances
Exploration of the minimum facility resources to support primary transportation of patients with severe TBI
Examination of optimum destination for patients with mild to moderate TBI based on patient outcome
Guideline Update and Conclusions and Summary of Future Directions
In conclusion, a robust body of evidence supports that organized and scientifically based medical care of TBI patients in the prehospital setting improves outcomes. Prehospital health care professionals are charged with the challenging tasks of accurately suspecting TBI following head injury and providing life-supporting interventions in a matter of minutes. Scientific evidence directs focus to parameters informing airway, breathing, circulation, and other physiologic measures that guide timely medical interventions and are summarized in . In the midst of providing critical care to a TBI patient, prehospital professionals also strategize transport, including risk to their own safety.
Figure 4. Prehospital algorithm for evaluation and management of patients with suspicion for moderate to severe TBI.
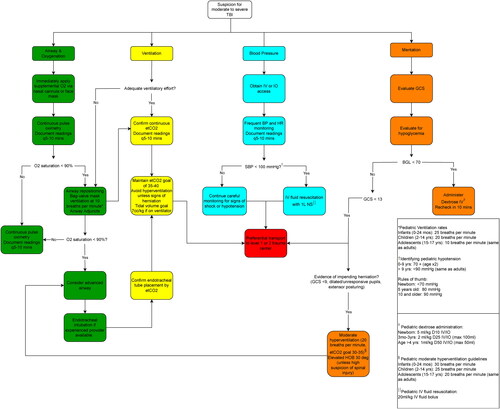
While this guideline informs best practices in care and has identified important differences among pediatric and geriatric populations, it did not evaluate the body of evidence pertaining to other demographical and medical differences such as sex, specific region, race/ethnicity, insurer status, socioeconomical status, effect of other medical problems on prehospital care determinants, and beyond. These important topics, along with those discussed in each topical update’s Future Interventions section represent much work to be done to advance the quality of care provided to patients acutely following TBI. In general, the most meaningful effect to patient care occurs via high-quality controlled studies that include implementation plans.
Algorithm
Individual studies will be rated as “good,” “fair,” or “poor.” Studies rated “good” quality will be considered to have low risk of bias, and their results will be considered valid. Good quality studies include clear descriptions of the population, setting, interventions, and comparison groups; a valid method for allocation of patients to treatment or identifying the treatment and control groups in observational studies; low dropout rates and clear reporting of dropouts; appropriate means of controlling for confounding; and appropriate measurement of outcomes. Studies rated “fair” quality will be susceptible to some bias, though not enough to invalidate the results. These studies may not meet all the criteria for a rating of good quality, but no flaw is likely to cause major bias. The study may be missing information, making it difficult to assess limitations and potential problems. The fair quality category is broad, and studies with this rating will vary in their strengths and weaknesses. The results of some fair quality studies are likely to be valid, while for others the validity may be uncertain. Studies rated “poor” quality will have significant flaws that imply biases of various types that may invalidate the results. They will have a serious or “fatal” flaw in design, analysis, or reporting; large amounts of missing information; discrepancies in reporting; or serious problems in the delivery of the intervention. The results of these studies will be as likely to reflect flaws in the study design as the true difference between the compared interventions. Poor quality studies will be considered to be less reliable than higher quality studies when synthesizing the evidence, particularly if discrepancies between studies of differing quality are present.
Each study evaluated were dual-reviewed for quality by two review team members and reviewed by one to three clinical investigators (CIs) designated as the lead on the topic. Any disagreements were resolved by consensus.
Data Synthesis
We constructed evidence tables identifying the study characteristics (as described in the Data Abstraction and Data Management section), outcomes, and quality ratings for all included studies. These are then summarized in the “in-text” tables, although detailed abstractions in spreadsheets are provided to CIs for use during the recommendation development process.
To determine whether meta-analysis could be meaningfully performed, we considered the quality of the studies and the heterogeneity among studies in the design, patient population, interventions, and outcomes. The key questions are designed to assess the comparative effectiveness and harms by patient demographics, comorbidities, and treatment features.
Qualitative data about the studies will be summarized in tables, and descriptive analysis and interpretation of the results will be provided to the CIs for review and elaboration.
Grading the Quality of the Body of Evidence
The strength of evidence for each key question will be initially assessed by one researcher for each outcome (see the PICOTS above) once all evidence is identified and the searches are closed. This will be assess using the standards established by the Agency for Healthcare Research and Quality Evidence-based Practice methods guidance. To ensure consistency and validity of the evaluation, the grades will be reviewed by the entire panel of CIs as well as the review team for:
Study limitations (low, medium, or high level of study limitations based on study design and the quality/risk of bias of the included studies)
Consistency (consistent or inconsistent findings, or unknown/not applicable)
Directness (direct or indirect evidence)
Precision (precise or imprecise estimates of effect)
The quality of the body evidence will be assigned an overall grade of high, moderate, low, or insufficient according to a four-level scale by evaluating and weighing the combined results of the above domains:
High: We are very confident that the estimate of effect lies close to the true effect for this outcome. The body of evidence has few or no deficiencies. We believe that the findings are stable, i.e., another study would not change the conclusions.
Moderate: We are moderately confident that the estimate of effect lies close to the true effect for this outcome. The body of evidence has some deficiencies. We believe that the findings are likely to be stable, but some doubt remains.
Low: We have limited confidence that the estimate of effect lies close to the true effect for this outcome. The body of evidence has major or numerous deficiencies (or both). We believe that additional evidence is needed before concluding either that the findings are stable or that the estimate of effect is close to the true effect.
Insufficient: We have no evidence or very limited evidence. We are unable to estimate an effect, or we have no confidence in the estimate of effect for this outcome. No evidence is available, or the body of evidence has unacceptable deficiencies, precluding reaching a conclusion.
Assessing Applicability
Applicability considers the extent to which results from a study or a body of evidence can be used to answer the questions of interest. Variability in the studies or studies with unique attributes may limit the ability to generalize the results to other populations, and settings. What may affect applicability can vary depending on the question of interest and currently the assessment of applicability is not standardized.
For this review we will consider if applicability is affected by the characteristics of the patient populations (e.g., demographic characteristics, primary condition or disability, presence of co-morbidities) and the setting of the study (including geographic location and practice context). We will identity if individual studies have potential applicability issues during data abstraction and quality assessment, and then we will summarize our findings into an assessment of the applicability of the body of evidence available to answer each question and to inform evidence-based recommendations.
Brain Trauma Foundation Investigators (Ordered)
1. Al Lulla, MD, Department of Emergency Medicine, UT Southwestern Medical Center, Dallas, Texas ([email protected]); 2. Angela Lumba-Brown, MD, Department of Emergency Medicine, Stanford University, Stanford, California ([email protected]); 3. Annette M. Totten, PhD, Department of Medical Informatics and Clinical Epidemiology, Oregon Health & Science University, Portland, Oregon ([email protected]); 4. Patrick J. Maher, MD, MS, Department of Emergency Medicine, Mount Sinai, New York, New York ([email protected]); 5. Neeraj Badjatia, MD, MS, Department of Neurocritical Care, Neurology, Anesthesiology, Neurosurgery, University of Maryland School of Medicine, Baltimore, Maryland ([email protected]) (Lorine Anderson [email protected]); 6. Randy Bell, MD, Uniformed Services University, Bethesda, Maryland, ([email protected]); 7. Christina “TJ” Donayri, BSN, RN, CEN, TCRN, Trauma Services, Queens Medical Center, Honolulu, Hawaii ([email protected]); 8. Mary E. Fallat, MD, Hiram C. Polk Jr Department of Pediatric Surgery, University of Louisville, Norton Children’s Hospital, Louisville, Kentucky ([email protected]); 9. Gregory W. J. Hawryluk, MD, PhD, Department of Neurosurgery, Cleveland Clinic, Fairlawn OH, ([email protected]); 10.Scott A. Goldberg, MD, Department of Emergency Medicine, Brigham and Women’s Hospital, Harvard Medical School, Boston, Massachusetts ([email protected]); 11. Halim M. A. Hennes, MD, MS, Department of Pediatric Emergency Medicine, UT Southwestern Medical Center, Dallas Children’s Medical Center, Dallas, Texas ([email protected]); 12. Steven P. Ignell, MD, Department of Emergency Medicine, Stanford University, Stanford, California ([email protected]); 13. Jamshid Ghajar, MD, PhD, Department of Neurosurgery, Stanford University, Stanford, California ([email protected]); 14. Brian Krzyzaniak, Brian P. Krzyzaniak BA, EMT-P, Brian ([email protected]); 15. E. Brooke Lerner, PhD, MS, EMT-P, Department of Emergency Medicine, Medical College of Wisconsin, Milwaukee, Wisconsin ([email protected]) (Katherine Murray [email protected]); 16. Daniel Nishijima, MD, MAS, Department of Emergency Medicine, UC Davis, Sacramento, California ([email protected]); 17. Charles Schleien, MD, MBA, Pediatric Critical Care, Cohen Children’s Medical Center, Hofstra Northwell School of Medicine, New Hyde Park, New York ([email protected]) (Jennifer Genovese [email protected]); 18. Stacy Shackelford, MD, Trauma and Critical Care, USAF Center for Sustainment of Trauma Readiness Skills ([email protected]); 19. Erik Swartz, PhD, ATC, Department of Physical Therapy and Kinesiology, University of Massachusetts, Lowell, MA ([email protected]); 20. David W. Wright, MD, Department of Emergency Medicine, Emory University, Atlanta, Georgia ([email protected]); 21. Rachel Zhang, University of Arizona College of Medicine, Phoenix, Arizona ([email protected]); 22. Andy Jagoda, MD, Department of Emergency Medicine, Mount Sinai, New York, New York [email protected] ([email protected]); 23. Bentley J. Bobrow MD, Department of Emergency Medicine, University of Texas, Houston, Texas [email protected]; Specialties represented: Emergency medicine, pediatric emergency medicine, emergency medical services, trauma surgery, neurosurgery, pediatric surgery, pediatric critical care, critical care, neurocritical care, trauma services, nursing, previous patients.
Education and Implementation Subcommittee
Algorithm Leads: Bentley J. Bobrow, Department of Emergency Medicine, University of Texas, Houston, Texas [email protected], Angela Lumba-Brown, Department of Emergency Medicine, Stanford University, Stanford, California [email protected], Al Lulla, Department of Emergency Medicine, UT Southwestern Medical Center, Dallas, Texas [email protected]
Disclaimer of Liability
This prehospital guideline on the management of traumatic brain injury reflects the current state of scientific knowledge at the time of completion of the literature search with incorporation of related evidence through January 2022 and clinical consensus-based recommendations. This work is intended to provide support in clinical decision-making for health care professionals, and future need for recommendation updates is anticipated as the scientific body of evidence surrounding the management of traumatic brain injury is expanded. This guideline and its recommendations are not intended to provide stand-alone or absolute medical decision-making. If medical advice or assistance is required, the services of a qualified physician should be sought. The recommendations contained in these guidelines may not be appropriate for use in all clinical settings or circumstances. Ultimate clinical decision-making remains the responsibility of treating health care professionals weighing circumstances presented by the individual patient, available resources for care, and the known variability and biological behavior of the injury. Any opinions, findings, and conclusions expressed in this material are those of the authors and do not necessarily reflect the views of the U.S. Army Contracting Command, Aberdeen Proving Ground, Natick Contracting Division, Stanford University, or the Brain Trauma Foundation.
Supplemental Material
Download Zip (38.1 KB)Disclosure Statement
Jamshid Ghajar is the President of Brain Trauma Foundation. No potential conflict of interest was reported by the other authors.
Additional information
Funding
References
- Dewan MC, Rattani A, Gupta S, Baticulon RE, Hung YC, Punchak M, et al. Estimating the global incidence of traumatic brain injury. J Neurosurg. 2018:1–18.
- Centers for Disease Control and Prevention, National Center for Injury Prevention and Control. Get the facts about TBI: Centers for disease control and prevention; 2022. Available from: https://www.cdc.gov/traumaticbraininjury/get_the_facts.html.
- Brown JB, Kheng M, Carney NA, Rubiano AM, Puyana JC. Geographical disparity and traumatic brain injury in America: rural areas suffer poorer outcomes. J Neurosci Rural Pract. 2019;10(1):10–5.
- The National Highway Traffic Safety Administration. The national EMS scope of practice model; 2007. Available from https://www.ems.gov/pdf/education/EMS-Education-for-the-Future-A-Systems-Approach/National_EMS_Scope_Practice_Model.pdf.
- Badjatia N, Carney N, Crocco TJ, Fallat ME, Hennes HMA, Jagoda AS, et al. Guidelines for prehospital management of traumatic brain injury 2nd edition. Prehosp Emerg Care. 2008;12(1):S1–S52.
- The Brain Trauma Foundation. The American Association of Neurological Surgeons: the joint section on neurotrauma and critical care. J Neurotrauma. 2000;17(6–7):513–20.
- Spaite DW, Bobrow BJ, Keim SM, Barnhart B, Chikani V, Gaither JB, et al. Association of statewide implementation of the prehospital traumatic brain injury treatment guidelines with patient survival following traumatic brain injury: the excellence in prehospital injury care (EPIC) study. JAMA Surg. 2019;154(7):e191152.
- Chuck CC, Martin TJ, Kalagara R, Shaaya E, Kheirbek T, Cielo D. Emergency medical services protocols for traumatic brain injury in the United States: a call for standardization. Injury. 2021;52(5):1145–50.
- Centre for Reviews and Dissemination: University of York, National Institute for Health Research. Prospero. Available from https://www.crd.york.ac.uk/prospero/.
- Agency for Healthcare Research and Quality. Methods guide for effectiveness and comparative effectiveness reviews: agency for healthcare research and quality (US); 2008. Available from https://www.ncbi.nlm.nih.gov/books/NBK47095.
- Moher D, Liberati A, Tetzlaff J, Altman DG. Preferred reporting items for systematic reviews and meta-analyses: the PRISMA statement. J Clin Epidemiol. 2009;62(10):1006–12.
- Stroup DF, Berlin JA, Morton SC, Olkin I, Williamson GD, Rennie D, et al. Meta-analysis of observational studies in epidemiology: a proposal for reporting. JAMA. 2000;283(15):2008–12.
- Bergmans SF, Schober P, Schwarte LA, Loer SA, Bossers SM. Prehospital fluid administration in patients with severe traumatic brain injury: a systematic review and meta-analysis. Injury. 2020;51(11):2356–67.
- Shibahashi K, Hoda H, Okura Y, Hamabe Y. Acceptable blood pressure levels in the prehospital setting for patients with traumatic brain injury: a multicenter observational study. World Neurosurg. 2021;149:e504–e11.
- Spaite DW, Hu C, Bobrow BJ, Barnhart B, Chikani V, Gaither JB, et al. Optimal out-of-hospital blood pressure in major traumatic brain injury: a challenge to the current understanding of hypotension. Ann Emerg Med. 2022;80(1):46–59.
- Suttipongkaset P, Chaikittisilpa N, Vavilala MS, Lele AV, Watanitanon A, Chandee T, et al. Blood pressure thresholds and mortality in pediatric traumatic brain injury. Pediatrics. 2018;142(2):e20180594.
- Barmparas G, Liou DZ, Lamb AW, Gangi A, Chin M, Ley EJ, et al. Prehospital hypertension is predictive of traumatic brain injury and is associated with higher mortality. J Trauma Acute Care Surg. 2014;77(4):592–8.
- Yumoto T, Mitsuhashi T, Yamakawa Y, Iida A, Nosaka N, Tsukahara K, et al. Impact of Cushing’s sign in the prehospital setting on predicting the need for immediate neurosurgical intervention in trauma patients: a nationwide retrospective observational study. Scand J Trauma Resusc Emerg Med. 2016;24(1):147.
- Zebrack M, Dandoy C, Hansen K, Scaife E, Mann NC, Bratton SL. Early resuscitation of children with moderate-to-severe traumatic brain injury. Pediatrics. 2009;124(1):56–64.
- Brorsson C, Rodling-Wahlström M, Olivecrona M, Koskinen LO, Naredi S. Severe traumatic brain injury: consequences of early adverse events. Acta Anaesthesiol Scand. 2011;55(8):944–51.
- Davis DP, Meade W, Sise MJ, Kennedy F, Simon F, Tominaga G, et al. Both hypoxemia and extreme hyperoxemia may be detrimental in patients with severe traumatic brain injury. J Neurotrauma. 2009;26(12):2217–23.
- Chi JH, Knudson MM, Vassar MJ, McCarthy MC, Shapiro MB, Mallet S, Holcroft JJ, Moncrief H, Noble J, Wisner D, et al. Prehospital hypoxia affects outcome in patients with traumatic brain injury: a prospective multicenter study. J Trauma. 2006;61(5):1134–41.
- Gaither JB, Chikani V, Stolz U, Viscusi C, Denninghoff K, Barnhart B, et al. Body temperature after EMS transport: association with traumatic brain injury outcomes. Prehosp Emerg Care. 2017;21(5):575–82.
- Stocchetti N, Maas AI. Traumatic intracranial hypertension. N Engl J Med. 2014;370(22):2121–30.
- Davis DP, Dunford JV, Poste JC, Ochs M, Holbrook T, Fortlage D, et al. The impact of hypoxia and hyperventilation on outcome after paramedic rapid sequence intubation of severely head-injured patients. J Trauma. 2004;57(1):1–8.
- Chesnut RM, Marshall LF, Klauber MR, Blunt BA, Baldwin N, Eisenberg HM, Jane JA, Marmarou A, Foulkes MA. The role of secondary brain injury in determining outcome from severe head injury. J Trauma. 1993;34(2):216–22.
- Spaite DW, Hu C, Bobrow BJ, Chikani V, Barnhart B, Gaither JB, et al. The effect of combined out-of-hospital hypotension and hypoxia on mortality in major traumatic brain injury. Ann Emerg Med. 2017;69(1):62–72.
- Spaite DW, Hu C, Bobrow BJ, Chikani V, Barnhart B, Gaither JB, et al. Association of out-of-hospital hypotension depth and duration with traumatic brain injury mortality. Ann Emerg Med. 2017;70(4):522–30.
- Shibahashi K, Sugiyama K, Okura Y, Tomio J, Hoda H, Hamabe Y. Defining hypotension in patients with severe traumatic brain injury. World Neurosurg. 2018;120:e667–e74.
- Jafari AA, Shah M, Mirmoeeni S, Hassani MS, Nazari S, Fielder T, Godoy DA, Seifi A. Paroxysmal sympathetic hyperactivity during traumatic brain injury. Clin Neurol Neurosurg. 2022;212:107081.
- Sellmann T, Miersch D, Kienbaum P, Flohé S, Schneppendahl J, Lefering R. The impact of arterial hypertension on polytrauma and traumatic brain injury. Dtsch Arztebl Int. 2012;109(49):849–56.
- Kokoska ER, Smith GS, Pittman T, Weber TR. Early hypotension worsens neurological outcome in pediatric patients with moderately severe head trauma. J Pediatr Surg. 1998;33(2):333–8.
- Young AMH, Donnelly J, Liu X, Guilfoyle MR, Carew M, Cabeleira M, et al. Pre-hospital predictors of impaired ICP trends in continuous monitoring of paediatric traumatic brain injury patients. Acta Neurochir Suppl. 2018;126:7–10.
- Kannan N, Wang J, Mink RB, Wainwright MS, Groner JI, Bell MJ, et al. Timely hemodynamic resuscitation and outcomes in severe pediatric traumatic brain injury: preliminary findings. Pediatr Emerg Care. 2018;34(5):325–9.
- Teasdale G, Jennett B. Assessment of coma and impaired consciousness. A practical scale. Lancet. 1974;2(7872):81–4.
- American Academy of Pediatrics, American College of Emergency Physicians. APLS: the pediatric emergency medicine course. 3rd ed. Dallas, TX: American Academy of Pediatrics; 1998. p. 287.
- Borgialli DA, Mahajan P, Hoyle JD, Powell EC, Nadel FM, Tunik MG, et al. Performance of the pediatric Glasgow coma scale score in the evaluation of children with blunt head trauma. Acad Emerg Med. 2016;23(8):878–84.
- Winkler JV, Rosen P, Alfry EJ. Prehospital use of the Glasgow coma scale in severe head injury. J Emerg Med. 1984;2(1):1–6.
- Lesko MM, Jenks T, O'Brien SJ, Childs C, Bouamra O, Woodford M, et al. Comparing model performance for survival prediction using total Glasgow coma scale and its components in traumatic brain injury. J Neurotrauma. 2013;30(1):17–22.
- Davis DP, Vadeboncoeur TF, Ochs M, Poste JC, Vilke GM, Hoyt DB. The association between field Glasgow coma scale score and outcome in patients undergoing paramedic rapid sequence intubation. J Emerg Med. 2005;29(4):391–7.
- Davis DP, Serrano JA, Vilke GM, Sise MJ, Kennedy F, Eastman AB, et al. The predictive value of field versus arrival Glasgow coma scale score and TRISS calculations in moderate-to-severe traumatic brain injury. J Trauma. 2006;60(5):985–90.
- Najafi Z, Zakeri H, Mirhaghi A. The accuracy of acuity scoring tools to predict 24-h mortality in traumatic brain injury patients: a guide to triage criteria. Int Emerg Nurs. 2018;36:27–33.
- Sadaka F, Jadhav A, Miller M, Saifo A, O'Brien J, Trottier S. Is it possible to recover from traumatic brain injury and a Glasgow coma scale score of 3 at emergency department presentation? Am J Emerg Med. 2018;36(9):1624–6.
- Hoffmann M, Lefering R, Rueger JM, Kolb JP, Izbicki JR, Ruecker AH, et al. Pupil evaluation in addition to Glasgow coma scale components in prediction of traumatic brain injury and mortality. Br J Surg. 2012;99(1):122–30.
- Rau C-S, Wu S-C, Chen Y-C, Chien P-C, Hsieh H-Y, Kuo P-J, et al. Effect of age on Glasgow coma scale in patients with moderate and severe traumatic brain injury: an approach with propensity score-matched population. Int J Environ Res Public Health. 2017;14(11):1378.
- Caterino JM, Raubenolt A, Cudnik MT. Modification of Glasgow Coma Scale criteria for injured elders. Acad Emerg Med. 2011;18(10):1014–21.
- Thompson DO, Hurtado TR, Liao MM, Byyny RL, Gravitz C, Haukoos JS. Validation of the simplified motor score in the out-of-hospital setting for the prediction of outcomes after traumatic brain injury. Ann Emerg Med. 2011;58(5):417–25.
- Caterino JM, Raubenolt A. The prehospital simplified motor score is as accurate as the prehospital Glasgow coma scale: analysis of a statewide trauma registry. Emerg Med J. 2012;29(6):492–6.
- Haukoos JS, Gill MR, Rabon RE, Gravitz CS, Green SM. Validation of the Simplified Motor Score for the prediction of brain injury outcomes after trauma. Ann Emerg Med. 2007;50(1):18–24.
- Gill M, Steele R, Windemuth R, Green SM. A comparison of five simplified scales to the out-of-hospital Glasgow Coma Scale for the prediction of traumatic brain injury outcomes. Acad Emerg Med. 2006;13(9):968–73.
- Singh B, Murad MH, Prokop LJ, Erwin PJ, Wang Z, Mommer SK, et al. Meta-analysis of Glasgow coma scale and simplified motor score in predicting traumatic brain injury outcomes. Brain Inj. 2013;27(3):293–300.
- Chou R, Totten AM, Pappas M, Carney N, Dandy S, Grusing S, et al. Glasgow coma scale for field triage of trauma: a systematic review. Rockville (MD): Agency for Healthcare Research and Quality (US); 2017.
- Becker A, Peleg K, Olsha O, Givon A, Kessel B. Israeli Trauma G. Analysis of incidence of traumatic brain injury in blunt trauma patients with Glasgow Coma Scale of 12 or less. Chin J Traumatol. 2018;21(3):152–5.
- Gang MC, Hong KJ, Shin SD, Song KJ, Ro YS, Kim TH, et al. New prehospital scoring system for traumatic brain injury to predict mortality and severe disability using motor Glasgow Coma Scale, hypotension, and hypoxia: a nationwide observational study. Clin Exp Emerg Med. 2019;6(2):152–9.
- Nesiama J-AO, Pirallo RG, Lerner EB, Hennes H. Does a prehospital Glasgow Coma Scale score predict pediatric outcomes? Pediatr Emerg Care. 2012;28(10):1027–32.
- Emami P, Czorlich P, Fritzsche FS, Westphal M, Rueger JM, Lefering R, et al. Impact of Glasgow Coma Scale score and pupil parameters on mortality rate and outcome in pediatric and adult severe traumatic brain injury: a retrospective, multicenter cohort study. J Neurosurg. 2017;126(3):760–7.
- Majdan M, Steyerberg EW, Nieboer D, Mauritz W, Rusnak M, Lingsma HF. Glasgow coma scale motor score and pupillary reaction to predict six-month mortality in patients with traumatic brain injury: comparison of field and admission assessment. J Neurotrauma. 2015;32(2):101–8.
- Schreiber MA, Aoki N, Scott BG, Beck JR. Determinants of mortality in patients with severe blunt head injury. Arch Surg. 2002;137(3):285–90.
- McCabe CF, Donahue SP. Prognostic indicators for vision and mortality in shaken baby syndrome. Arch Ophthalmol. 2000;118(3):373–7.
- Sobuwa S, Hartzenberg HB, Geduld H, Uys C. Predicting outcome in severe traumatic brain injury using a simple prognostic model. S Afr Med J. 2014;104(7):492–4.
- Chaudhuri K, Malham GM, Rosenfeld JV. Survival of trauma patients with coma and bilateral fixed dilated pupils. Injury. 2009;40(1):28–32.
- Mauritz W, Leitgeb J, Wilbacher I, Majdan M, Janciak I, Brazinova A, et al. Outcome of brain trauma patients who have a Glasgow Coma Scale score of 3 and bilateral fixed and dilated pupils in the field. Eur J Emerg Med. 2009;16(3):153–8.
- Gabriel EJ, Ghajar J, Jagoda A, Pons PT, Scalea T, Walters BC. Guidelines for prehospital management of traumatic brain injury. J Neurotrauma. 2002;19(1):111–74.
- Steck RP, Kong M, McCray KL, Quan V, Davey PG. Physiologic anisocoria under various lighting conditions. Clin Ophthalmol. 2018;12:85–9.
- Chesnut RM, Gautille T, Blunt BA, Klauber MR, Marshall LE. The localizing value of asymmetry in pupillary size in severe head injury: relation to lesion type and location. Neurosurgery. 1994;34(5):840–5.
- Mamelak AN, Pitts LH, Damron S. Predicting survival from head trauma 24 hours after injury: a practical method with therapeutic implications. J Trauma. 1996;41(1):91–9.
- Ritter AM, Muizelaar JP, Barnes T, Choi S, Fatouros P, Ward J, et al. Brain stem blood flow, pupillary response, and outcome in patients with severe head injuries. Neurosurgery. 1999;44(5):941–8.
- Bossers SM, Schwarte LA, Loer SA, Twisk JWR, Boer C, Schober P. Experience in prehospital endotracheal intubation significantly influences mortality of patients with severe traumatic brain injury: a systematic review and meta-analysis. PLoS One. 2015;10(10):e0141034.
- von Elm E, Schoettker P, Henzi I, Osterwalder J, Walder B. Pre-hospital tracheal intubation in patients with traumatic brain injury: systematic review of current evidence. Br J Anaesth. 2009;103(3):371–86.
- Sarkar M, Niranjan N, Banyal PK. Mechanisms of hypoxemia. Lung India. 2017;34(1):47–60.
- Denninghoff KR, Griffin MJ, Bartolucci AA, Lobello SG, Fine PR. Emergent endotracheal intubation and mortality in traumatic brain injury. West J Emerg Med. 2008;9(4):184–9.
- Lansom JD, Curtis K, Goldsmith H, Tzannes A. The effect of prehospital intubation on treatment times in patients with suspected traumatic brain injury. Air Med J. 2016;35(5):295–300.
- Haltmeier T, Benjamin E, Siboni S, Dilektasli E, Inaba K, Demetriades D. Prehospital intubation for isolated severe blunt traumatic brain injury: worse outcomes and higher mortality. Eur J Trauma Emerg Surg. 2017;43(6):731–9.
- Denninghoff KR, Nuño T, Pauls Q, Yeatts SD, Silbergleit R, Palesch YY, et al. Prehospital intubation is associated with favorable outcomes and lower mortality in ProTECT III. Prehosp Emerg Care. 2017;21(5):539–44.
- Davis DP, Hoyt DB, Ochs M, Fortlage D, Holbrook T, Marshall LK, Rosen P. The effect of paramedic rapid sequence intubation on outcome in patients with severe traumatic brain injury. J Trauma. 2003;54(3):444–53.
- Bendinelli C, Ku D, Nebauer S, King KL, Howard T, Gruen R, Evans T, Fitzgerald M, Balogh ZJ. A tale of two cities: prehospital intubation with or without paralysing agents for traumatic brain injury. ANZ J Surg. 2018;88(5):455–9.
- Bendinelli C, Bivard A, Nebauer S, Parsons MW, Balogh ZJ. Brain CT perfusion provides additional useful information in severe traumatic brain injury. Injury. 2013;44(9):1208–12.
- Davis DP, Stern J, Sise MJ, Hoyt DB. A follow-up analysis of factors associated with head-injury mortality after paramedic rapid sequence intubation. J Trauma. 2005;59(2):486–90.
- Blanchard IE, Ahmad A, Tang KL, Ronksley PE, Lorenzetti D, Lazarenko G, et al. The effectiveness of prehospital hypertonic saline for hypotensive trauma patients: a systematic review and meta-analysis. BMC Emerg Med. 2017;17(1):35.
- Tan PG, Cincotta M, Clavisi O, Bragge P, Wasiak J, Pattuwage L, et al. Review article: Prehospital fluid management in traumatic brain injury. Emerg Med Australas. 2011;23(6):665–76.
- Junger WG, Rhind SG, Rizoli SB, Cuschieri J, Baker AJ, Shek PN, et al. Prehospital hypertonic saline resuscitation attenuates the activation and promotes apoptosis of neutrophils in patients with severe traumatic brain injury. Shock. 2013;40(5):366–74.
- Shackford SR, Bourguignon PR, Wald SL, Rogers FB, Osler TM, Clark DE. Hypertonic saline resuscitation of patients with head injury: a prospective, randomized clinical trial. J Trauma. 1998;44(1):50–8.
- Wade CE, Grady JJ, Kramer GC, Younes RN, Gehlsen K, Holcroft JW. Individual patient cohort analysis of the efficacy of hypertonic saline/dextran in patients with traumatic brain injury and hypotension. J Trauma. 1997;42(5):S61–S5.
- Vassar MJ, Perry CA, Gannaway WL, Holcroft JW. 7.5% sodium chloride/dextran for resuscitation of trauma patients undergoing helicopter transport. Arch Surg. 1991;126(9):1065–72.
- Vassar MJ, Perry CA, Holcroft JW. Prehospital resuscitation of hypotensive trauma patients with 7.5% NaCl versus 7.5% NaCl with added dextran: a controlled trial. J Trauma. 1993;34(5):622–32.
- Luerssen TG, Klauber MR, Marshall LF. Outcome from head injury related to patient’s age. A longitudinal prospective study of adult and pediatric head injury. J Neurosurg. 1988;68(3):409–16.
- Vassar MJ, Fischer RP, O'Brien PE, Bachulis BL, Chambers JA, Hoyt DB, et al. A multicenter trial for resuscitation of injured patients with 7.5% sodium chloride. The effect of added dextran 70. Arch Surg. 1993;128(9):1003–11.
- Vassar MJ, Perry CA, Holcroft JW. Analysis of potential risks associated with 7.5% sodium chloride resuscitation of traumatic shock. Arch Surg. 1990;125(10):1309–15. doi:10.1001/archsurg.1990.01410220093013.
- Cooper DJ, Myles PS, McDermott FT, Murray LJ, Laidlaw J, Cooper G, Tremayne AB, Bernard SS, Ponsford J. Prehospital hypertonic saline resuscitation of patients with hypotension and severe traumatic brain injury: a randomized controlled trial. JAMA. 2004;291(11):1350–7.
- Ter Avest E, Taylor S, Wilson M, Lyon RL. Prehospital clinical signs are a poor predictor of raised intracranial pressure following traumatic brain injury. Emerg Med J. 2021;38(1):21–6.
- Rowell SE, Meier EN, McKnight B, Kannas D, May S, Sheehan K, Bulger EM, Idris AH, Christenson J, Morrison LJ, et al. Effect of out-of-hospital tranexamic acid vs placebo on 6-month functional neurologic outcomes in patients with moderate or severe traumatic brain injury. JAMA. 2020;324(10):961–74.
- Russell GB, Graybeal JM. Reliability of the arterial to end-tidal carbon dioxide gradient in mechanically ventilated patients with multisystem trauma. J Trauma. 1994;36(3):317–22.
- Muizelaar JP, Marmarou A, Ward JD, Kontos HA, Choi SC, Becker DP, et al. Adverse effects of prolonged hyperventilation in patients with severe head injury: a randomized clinical trial. J Neurosurg. 1991;75(5):731–9.
- Davis DP, Peay J, Serrano JA, Buono C, Vilke GM, Sise MJ, Kennedy F, Eastman AB, Velky T, Hoyt DB, et al. The impact of aeromedical response to patients with moderate to severe traumatic brain injury. Ann Emerg Med. 2005;46(2):115–22.
- Davis DP, Dunford JV, Ochs M, Park K, Hoyt DB. The use of quantitative end-tidal capnometry to avoid inadvertent severe hyperventilation in patients with head injury after paramedic rapid sequence intubation. J Trauma. 2004;56(4):808–14.
- Dumont TM, Visioni AJ, Rughani AI, Tranmer BI, Crookes B. Inappropriate prehospital ventilation in severe traumatic brain injury increases in-hospital mortality. J Neurotrauma. 2010;27(7):1233–41.
- Caulfield EV, Dutton RP, Floccare DJ, Stansbury LG, Scalea TM. Prehospital hypocapnia and poor outcome after severe traumatic brain injury. J Trauma. 2009;66(6):1577–82.
- Lal D, Weiland S, Newton M, Flaten A, Schurr M. Prehospital hyperventilation after brain injury: a prospective analysis of prehospital and early hospital hyperventilation of the brain-injured patient. Prehosp Disaster Med. 2003;18(1):20–3.
- Donnelly JA, Smith EA, Hope AT, Alexander RJ. An assessment of portable carbon dioxide monitors during interhospital transfer. Anaesthesia. 1995;50(8):703–5.
- Servadei F, Nasi MT, Cremonini AM, Giuliani G, Cenni P, Nanni A. Importance of a reliable admission Glasgow Coma Scale score for determining the need for evacuation of posttraumatic subdural hematomas: a prospective study of 65 patients. J Trauma. 1998;44(5):868–73.
- Schwartz ML, Tator CH, Rowed DW, Reid SR, Meguro K, Andrews DF. The University of Toronto head injury treatment study: a prospective, randomized comparison of pentobarbital and mannitol. Can J Neurol Sci. 1984;11(4):434–40.
- De Vivo P, Del Gaudio A, Ciritella P, Puopolo M, Chiarotti F, Mastronardi E. Hypertonic saline solution: a safe alternative to mannitol 18% in neurosurgery. Minerva Anestesiol. 2001;67(9):603–11.
- Gemma M, Cozzi S, Tommasino C, Mungo M, Calvi MR, Cipriani A, et al. 7.5% hypertonic saline versus 20% mannitol during elective neurosurgical supratentorial procedures. J Neurosurg Anesthesiol. 1997;9(4):329–34.
- Munar F, Ferrer AM, de Nadal M, Poca MA, Pedraza S, Sahuquillo J, et al. Cerebral hemodynamic effects of 7.2% hypertonic saline in patients with head injury and raised intracranial pressure. J Neurotrauma. 2000;17(1):41–51.
- Qureshi AI, Wilson DA, Traystman RJ. Treatment of elevated intracranial pressure in experimental intracerebral hemorrhage: comparison between mannitol and hypertonic saline. Neurosurgery. 1999;44(5):1055–63.
- Smith HP, Kelly DL, McWhorter JM, Armstrong D, Johnson R, Transou C, et al. Comparison of mannitol regimens in patients with severe head injury undergoing intracranial monitoring. J Neurosurg. 1986;65(6):820–4.
- Collaborators C-t. Effects of tranexamic acid on death, disability, vascular occlusive events and other morbidities in patients with acute traumatic brain injury (CRASH-3): a randomised, placebo-controlled trial. Lancet. 2019;394(10210):1713–23.
- Bossers SM, Loer SA, Bloemers FW, D, Hartog D, Van Lieshout EMM, Hoogerwerf N, et al. Association between prehospital tranexamic acid administration and outcomes of severe traumatic brain injury. JAMA Neurol. 2021;78(3):338–45.
- MacKenzie EJ, Rivara FP, Jurkovich GJ, Nathens AB, Frey KP, Egleston BL, et al. A national evaluation of the effect of trauma-center care on mortality. N Engl J Med. 2006;354(4):366–78.
- Sasser SM, Hunt RC, Faul M, Sugerman D, Pearson WS, Dulski T, et al. Guidelines for field triage of injured patients: recommendations of the National Expert Panel on Field Triage, 2011. MMWR Recomm Rep. 2012;61(1):1–20.
- Guss DA, Meyer FT, Neuman TS, Baxt WG, Dunford JV, Jr., Griffith LD, et al. The impact of a regionalized trauma system on trauma care in San Diego County. Ann Emerg Med. 1989;18(11):1141–5.
- Mullins RJ, Veum-Stone J, Hedges JR, Zimmer-Gembeck MJ, Mann NC, Southard PA, et al. Influence of a statewide trauma system on location of hospitalization and outcome of injured patients. J Trauma. 1996;40(4):536–45.
- Hunt J, Hill D, Besser M, West R, Roncal S. Outcome of patients with neurotrauma: the effect of a regionalized trauma system. Aust N Z J Surg. 1995;65(2):83–6.
- Norwood S, Fernandez L, England J. The early effects of implementing American College of Surgeons level II criteria on transfer and survival rates at a rurally based community hospital. J Trauma. 1995;39(2):240–4.
- Hannan EL, Farrell LS, Cooper A, Henry M, Simon B, Simon R. Physiologic trauma triage criteria in adult trauma patients: are they effective in saving lives by transporting patients to trauma centers? J Am Coll Surg. 2005;200(4):584–92.
- Kelly ML, Roach MJ, Banerjee A, Steinmetz MP, Claridge JA. Functional and long-term outcomes in severe traumatic brain injury following regionalization of a trauma system. J Trauma Acute Care Surg. 2015;79(3):372–7.
- Fakhry SM, Ferguson PL, Johnson EE, Wilson DA. Hospitalization in low-level trauma centres after severe traumatic brain injury: review of a population-based emergency department data base. Brain Inj. 2017;31(11):1486–93.
- Kuimi BL, Moore L, Cissé B, Gagné M, Lavoie A, Bourgeois G, et al. Influence of access to an integrated trauma system on in-hospital mortality and length of stay. Injury. 2015;46(7):1257–61.
- Gale SC, Peters J, Hansen A, Dombrovskiy VY, Detwiler PW. Impact of transfer distance and time on rural brain injury outcomes. Brain Inj. 2016;30(4):437–40.
- Pickering A, Cooper K, Harnan S, Sutton A, Mason S, Nicholl J. Impact of prehospital transfer strategies in major trauma and head injury: systematic review, meta-analysis, and recommendations for study design. J Trauma Acute Care Surg. 2015;78(1):164–77.
- Nishijima DK, Gaona SD, Faul M, Tancredi DJ, Waechter T, Maloney R, et al. The association of trauma center transport and long-term functional outcomes in head-injured older adults transported by emergency medical services. Acad Emerg Med. 2020;27(3):207–16.
- Sewalt CA, Gravesteijn BY, Menon D, Lingsma HF, Maas AIR, Stocchetti N, et al. Primary versus early secondary referral to a specialized neurotrauma center in patients with moderate/severe traumatic brain injury: a CENTER TBI study. Scand J Trauma Resusc Emerg Med. 2021;29(1):113.
- Lecky FE, Russell W, McClelland G, Pennington E, Fuller G, Goodacre S, et al. Bypassing nearest hospital for more distant neuroscience care in head-injured adults with suspected traumatic brain injury: findings of the head injury transportation straight to neurosurgery (HITS-NS) pilot cluster randomised trial. BMJ Open. 2017;7(10):e016355.
- Prabhakaran K, Petrone P, Lombardo G, Stoller C, Policastro A, Marini CP. Mortality rates of severe traumatic brain injury patients: impact of direct versus nondirect transfers. J Surg Res. 2017;219:66–71.
- Johnson DL, Krishnamurthy S. Send severely head-injured children to a pediatric trauma center. Pediatr Neurosurg. 1996;25(6):309–14.
- McConnell KJ, Newgard CD, Mullins RJ, Arthur M, Hedges JR. Mortality benefit of transfer to level I versus level II trauma centers for head-injured patients. Health Serv Res. 2005;40(2):435–57.
- DuBose JJ, Browder T, Inaba K, Teixeira PG, Chan LS, Demetriades D. Effect of trauma center designation on outcome in patients with severe traumatic brain injury. Arch Surg. 2008;143(12):1213–7.
- Cornwell EE, 3rd, Chang DC, Phillips J, Campbell KA. Enhanced trauma program commitment at a level I trauma center: effect on the process and outcome of care. Arch Surg. 2003;138(8):838–43.
- Lombardo S, Scalea T, Sperry J, Coimbra R, Vercruysse G, Enniss T, et al. Neuro, trauma, or med/surg intensive care unit: Does it matter where multiple injuries patients with traumatic brain injury are admitted? Secondary analysis of the American Association for the Surgery of Trauma Multi-Institutional Trials Committee decompressive craniectomy study. J Trauma Acute Care Surg. 2017;82(3):489–96.
- Alkhoury F, Courtney J. Outcomes after severe head injury: a National Trauma Data Bank-based comparison of Level I and Level II trauma centers. Am Surg. 2011;77(3):277–80.
- Gross BW, Edavettal MM, Cook AD, Rinehart CD, Lynch CA, Bradburn EH, et al. Big children or little adults? A statewide analysis of adolescent isolated severe traumatic brain injury outcomes at pediatric versus adult trauma centers. J Trauma Acute Care Surg. 2017;82(2):368–73.
- Potoka DA, Schall LC, Gardner MJ, Stafford PW, Peitzman AB, Ford HR. Impact of pediatric trauma centers on mortality in a statewide system. J Trauma. 2000;49(2):237–45.
- Bardes JM, Benjamin E, Escalante AA, Wu J, Demetriades D. Severe traumatic brain injuries in children: does the type of trauma center matter? J Pediatr Surg. 2018;53(8):1523–5.
- Bekelis K, Missios S, Mackenzie TA. Prehospital helicopter transport and survival of patients with traumatic brain injury. Ann Surg. 2015;261(3):579–85.
- Aiolfi A, Benjamin E, Recinos G, De Leon Castro A, Inaba K, Demetriades D. Air versus ground transportation in isolated severe head trauma: a national trauma data bank study. J Emerg Med. 2018;54(3):328–34.
- Sun H, Samra NS, Kalakoti P, Sharma K, Patra DP, Dossani RH, et al. Impact of prehospital transportation on survival in skiers and snowboarders with traumatic brain injury. World Neurosurg. 2017;104:909–18.
- Baxt WG, Moody P. The impact of advanced prehospital emergency care on the mortality of severely brain-injured patients. J Trauma. 1987;27(4):365–9.
- Berlot G, L, Fata C, Bacer B, Biancardi B, Viviani M, Lucangelo U, et al. Influence of prehospital treatment on the outcome of patients with severe blunt traumatic brain injury: a single-centre study. Eur J Emerg Med. 2009;16(6):312–7.
- Bulger EM, Guffey D, Guyette FX, MacDonald RD, Brasel K, Kerby JD, et al. Impact of prehospital mode of transport after severe injury: a multicenter evaluation from the Resuscitation Outcomes Consortium. J Trauma Acute Care Surg. 2012;72(3):567–73.
- Di Bartolomeo S, Sanson S, Nardi G, Scian G, Michelutto F, Lattuada V. L. Effects of 2 patterns of prehospital care on the outcome of patients with severe head injury. Arch Surg. 2001;136(11):1293–300.
- Lokkeberg AR, Grimes RM. Assessing the influence of non-treatment variables in a study of outcome from severe head injuries. J Neurosurg. 1984;61(2):254–62.
- de Jongh MA, van Stel HF, Schrijvers AJ, Leenen LP, Verhofstad MH. The effect of Helicopter Emergency Medical Services on trauma patient mortality in the Netherlands. Injury. 2012;43(9):1362–7.
- Missios S, Bekelis K. Transport mode to level I and II trauma centers and survival of pediatric patients with traumatic brain injury. J Neurotrauma. 2014;31(14):1321–8.
- Heschl S, Meadley B, Butt AE, Bernard W, Smith S. K. Efficacy of pre-hospital rapid sequence intubation in paediatric traumatic brain injury: A 9-year observational study. Injury. 2018;49(5):916–20.
- Elswick CM, Wyrick D, Gurien LA, Rettiganti M, Gowen M, Pownall A, et al. Resource utilization and indications for helicopter transport of head-injured children. J Pediatr Surg. 2018;53(9):1795–9.
- Wilberger JE, Jr., Harris M, Diamond DL. Acute subdural hematoma: morbidity, mortality, and operative timing. J Neurosurg. 1991;74(2):212–8.
- Fuller G, Woodford M, Lawrence T, Coats T, Lecky F. Do prolonged primary transport times for traumatic brain injury patients result in deteriorating physiology? A cohort study. Prehosp Emerg Care. 2014;18(1):60–7.
- Fuller G, Lawrence T, Woodford M, Coats T, Lecky F. Emergency medical services interval and mortality in significant head injury: a retrospective cohort study. Eur J Emerg Med. 2015;22(1):42–8.
- Newgard CD, Meier EN, Bulger EM, Buick J, Sheehan K, Lin S, et al. Revisiting the "golden hour": an evaluation of out-of-hospital time in shock and traumatic brain injury. Ann Emerg Med. 2015;66(1):30–41.
- Meizoso JP, Valle EJ, Allen CJ, Ray JJ, Jouria JM, Teisch LF, et al. Decreased mortality after prehospital interventions in severely injured trauma patients. J Trauma Acute Care Surg. 2015;79(2):227–31.
- Tien HC, Jung V, Pinto R, Mainprize T, Scales DC, Rizoli SB. Reducing time-to-treatment decreases mortality of trauma patients with acute subdural hematoma. Ann Surg. 2011;253(6):1178–83.
- Dinh MM, Bein K, Roncal S, Byrne CM, Petchell J, Brennan J. Redefining the golden hour for severe head injury in an urban setting: the effect of prehospital arrival times on patient outcomes. Injury. 2013;44(5):606–10.