Abstract
Physical and chemical properties of activated carbon (AC) were analyzed to investigate the effects of adsorbate properties on AC adsorption performance. Fixed-bed adsorption experiments were conducted with toluene, acetone, and xylene as adsorbates. From the results, the adsorption capacities of the three adsorbates had the following order: xylene > toluene > acetone. The correlation between experimental data and adsorbate properties was also analyzed. The results showed that different functional groups corresponding to the properties of adsorbates influenced the adsorptive properties of AC differently. The adsorption capacity of AC increased linearly as the molecular weight, dynamic diameter, boiling point, and density of the adsorbate increased. However, adsorption capacity decreased as the polarity index and vapor pressure of the adsorbate increased. For adsorption onto three types of AC, the adsorption energies of the three adsorbates had the following order: xylene > toluene > acetone.
This paper focused on the research on adsorption behavior of activated carbon based on adsorbate properties. Adsorption experiments were conducted under the same condition while the adsorbates were toluene, acetone, and xylene, respectively. Correlation analysis between experimental data and adsorbate properties was conducted. The different groups have different influence on the adsorptive properties of ACs. The adsorption capacity of activated carbon increases with the increase of adsorbate molecular weight, dynamic diameter, boiling point, and density, and that this relationship is linear. The relationship between adsorption capacity and the polarity index and vapor pressure of adsorbate shows an opposite trend, and the adsorption capacities and adsorption energies of three kinds of activated carbon for these three adsorbates had the following order: xylene > toluene > acetone.
Introduction
Activated carbon (AC), an excellent adsorbent with an extensive pore structure and large specific surface area, is widely used to purify air (CitationAo et al., 2005; CitationLillo-Ródenas et al., 2006; CitationByeon et al., 2006). Its physical properties, including specific surface area, pore volume, and pore structure, are the main elements that determine its adsorptive capacity.
Substantial research on AC has been conducted. Chiang (CitationChiang, 2001) studied the influence of pore structure on AC's adsorptive properties and concluded that the adsorptive capacity was related to the pore size, shape, and distribution. Using benzene and toluene as adsorbates, Lillo-Ródenas (CitationLillo-Ródenas et al., 2005) studied the adsorption behavior of AC in low concentrations and concluded that the adsorption capacity of AC was closely related to pore structure. However, research on the influence of AC's physical properties on the adsorptive capacity is limited. The adsorbate molecular weight, concentration, boiling point, saturation vapor pressure, dynamic diameter, and polarity initially affect the affinity between the adsorbate and the AC, and eventually, they influence the breakthrough curve, adsorption isotherm, and adsorptive capacity. Hsieh (CitationHsieh et al., 2002) noted that sorbent molecule size, shape, and structure affected adsorptive capacity. Li (CitationLi et al., 2010) studied the impact of aromatic compound structure and property on adsorptive capacity and found that the adsorbate polarity and molecular structure affected adsorptive capacity. This research focused on the impact that adsorbate properties had on adsorption behavior. Adsorption experiments were conducted with toluene, acetone, and xylene as adsorbates, and the experimental results were analyzed.
Experimental
Adsorbent and adsorbate
Three types of commercial AC were selected as adsorbents. The specific surface area and pore diameter were measured using a SA3100 (Beckman Coulter, Inc., USA), an analyzer of specific surface area and pore diameter (CitationChen et al., 2009; CitationYang et al., 2006). The adsorption isotherm of nitrogen was measured at 77.4 K using the volumetric method. The specific surface area was calculated using the Brunauer-Emmett-Teller (BET) method. The microporous specific surface area and volume were calculated using the t-plot method, and the pore diameter distribution was measured using the Barrett-Joyner-Halenda (BJH) method, which analyzes the branches of the adsorption and desorption isotherms. The surface texture of the AC was captured with a scanning electron microscope (SEM) (CitationGong et al., 2009) (S-3000N; Hitachi Ltd., xx, xx, Japan). AC surface groups were tested with a Fourier infrared spectrometer (NEXUS670; Nicolet Company, xx, xx, USA) (CitationLu et al., 2007; CitationCosnier et al., 2005), and their content was analyzed with Boehm titration (CitationDaifullah et al., 2003).
Toluene, acetone, and xylene were selected as adsorbates. The molecular weight, concentration, boiling point, saturation vapor pressure, dynamic diameter, and polarity of the adsorbates are shown in (CitationMaštovská et al., 2004; CitationLee et al., 2002). As shown in , the molecular weight and boiling point of toluene and xylene were larger than those of acetone, whereas the polarity and saturation vapor pressure of acetone were larger than those of toluene and xylene. The molecular weights and concentrations had the following order: xylene > toluene > acetone. The properties listed in affected the adsorption behavior of AC.
Table 1. Comparison of different adsorbate properties
Adsorption experiments
A constant-temperature adsorption experiment was conducted at 25 °C under normal atmospheric pressure. The mass of AC was 20 g, and the inlet concentration of adsorbate was 2000 ppm. A diagram of the experimental device is shown in . The system was composed of an air distribution system (miniature washing spray device), a constant-temperature system (constant-temperature water tank; DC1015; Balance and Instruments Company, Shanghai, China), fixed-bed adsorbers, and a testing system (gas chromatograph; P-6890; Luan Ruihong Chemical Instrument Company, Shandong, China). The miniature pump cycled organic solvents until the vapor flowing through the spray and constant-temperature zones became saturated. The saturated vapor was then mixed with dry air, and the mixture ratio was regulated with a valve. The mixed air was pumped through the fixed-bed adsorbers where AC could adsorb the adsorbates. Effluent gas was discharged after purification. Equilibrium was established when the effluent gas concentration equaled that of the inlet concentration for 30 min.
Results and Discussion
Physicality of AC
The specific surface areas and pore volumes are shown in . As shown in this table, the specific surface areas had the following order: AC-1 > AC-3 > AC-2. The microporous specific surface areas had the following order: AC-2 > AC-3 > AC-1. The large specific surface area of AC-1 could be attributed to its macropores and mesopores. AC-2 had the maximum microporous specific surface area, and therefore its adsorption capacity was significant. The pore volumes and micropore volumes had the following orders: AC-1 > AC-2 > AC-3 and AC-2 > AC-3 > AC-1, respectively.
Table 2. Specific surface area and pore volume of AC
shows the SEM images of the three types of AC, with different-sized pores distributed on the AC surface. The pores of AC-1 were bigger than those of the others, indicating that AC-1 had more macropores and mesopores.
The pore diameter and micropore distribution are shown in . As shown in this figure, the pore diameter distribution was extensive. The three types of AC had pore diameters smaller than 200 nm, and micropores were the most numerous. However, the macropores and mesopores of AC-1 were significantly larger than those of the others. The micropore distribution was centered between 0.5–0.8 and 1.4–2 nm. Between 0.8 and 1.4 nm, the distribution of the three types of AC was similar. In the range from 1.4 to 2 nm, AC-1 > AC-3 > AC-2, and all the types of AC reached a plateau when the diameter was 1.65 nm. The micropores of AC-1 had a multimodal distribution: peaks occurred when the diameter was 0.55, 0.61, 0.63, 0.66, 0.71, and 0.76 nm. AC-2 and AC-3 exhibited bimodal distributions. The micropores of AC-2 were centered at 0.51, 0.72, and 0.78 nm, whereas those of AC-3 were centered at 0.62 and 0.74 nm.
To analyze the content of the surface groups, infrared (IR) analysis and Boehm titration were conducted. The results are shown in . There was no clear fluctuation in the peak value. The three types of AC had the same chemical properties, and the surface functional groups were mostly free. As shown in , Boehm titration has also shown that the surface functional groups were mostly free. The acidic functional group contents of AC-1 were larger than those of AC-2 and AC-3, which were similar. The basic group contents of AC-2 were smaller than AC-1 and AC-3, which were similar.
Table 3. Chemical surface groups of AC (mmol·g−1)
Adsorption results
shows the breakthrough curves of AC-1, AC-2, and AC-3. Adsorption was assumed to reach penetration when the ratio of outlet concentration to inlet concentration (C/C 0) equaled 0.1 and saturation when C/C 0 equaled 0.95. Acetone was the first to penetrate and saturate, toluene was the second, and xylene was the third. Different adsorbates behaved differently with the same type of AC; this was mainly caused by differences in adsorbate properties. The same adsorbate behaved differently with different types of AC; this was mainly caused by differences in AC properties.
The adsorption capacities of the three types of AC are shown in . For the same type of AC, the adsorption capacities had the following order: xylene > toluene > acetone. For the same adsorbate, different types of AC had different adsorption capacities. For xylene and toluene, the adsorption capacities had the following order: AC-1 > AC-3 > AC-2. For acetone, they had the following order: AC-3 > AC-2 > AC-1.
Table 4. Adsorption capacity of AC (g(100 g)−1)
Influence of surface groups in AC on adsorption behavior
shows the relationships between surface groups and the adsorption capacity of AC. Carboxyl mainly affected the adsorptive properties of toluene and xylene, whereas the lactone group mainly affected acetone. There was no significant correlation between the phenolic hydroxyl group and the three adsorbates. The contents of the carboxyl and lactone groups showed a negative linear correlation with adsorption capacity. The total acidic groups had a significant influence on the adsorptive properties of acetone, and the total basic groups also had a significant influence. Overall, different groups corresponding to the properties of adsorbates influenced the adsorptive properties of AC differently.
Influence of adsorbate physicalities on adsorption behavior
shows the relationships between the saturated adsorption capacity and the adsorbate molecular weight, concentration, boiling point, saturation vapor pressure, dynamic diameter, and polarity. As shown in , the adsorbate molecular weight, dynamic diameter, concentration, and boiling point had a positive linear correlation, and the coefficient of relation was more than 0.96. Polarity and saturation vapor pressure had a negative linear correlation.
Figure 6. Relationships between adsorbate properties and adsorption capacity of AC: (a) molecular weigh; (b) molecular diameter; (c) polarity index; (d) boiling point; (e) density; (f) vapor pressure.
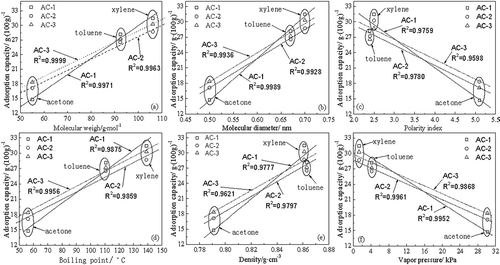
The adsorption of organic vapor to AC is mainly via physical adsorption, which is based on the van der Waals force between the adsorbate and AC. For the same number of molecules, the saturated adsorption capacity increases as the adsorbate molecular weight increases. For a given pore diameter distribution, the dynamic diameter of the adsorbate affects the adsorption capacity. In theory, the adsorbate can be adsorbed if the pore diameter is larger than the adsorbate dynamic diameter, which can be the effective adsorption pore diameter. However, if the pore diameter is many times larger than the adsorbate dynamic diameter, the adsorption capacity is very small, and the pore diameter acts as a channel. The polarity index reflects the intensity of adsorbate polarity. Xylene and toluene are nonpolar molecules, whereas acetone is a polar molecule. AC is a nonpolar molecule, and therefore the affinity between AC and xylene and toluene is strong. As a result, AC easily adsorbs xylene and toluene. The physical adsorption of organic vapor to AC is similar to gas liquefaction and condensation, which are both closely related to boiling point. At higher boiling points, liquefaction and condensation occur more readily, and this increases the adsorption capacity. Liang (CitationLiang, 2008) reported that adsorption was increasingly easy for gaseous substances with higher boiling points because capillary condensation occurred during the adsorption process. Concentration is associated with molecular weight; under the same conditions, higher concentrations indicate greater molecular weights. As mentioned above, adsorption capacity increases with molecular weight. The saturation vapor pressure, which is related to the boiling point and the relative volatility of a fluid mixture, describes the equilibrium of the gas-liquid phase and is one of the most important physical properties of a liquid. AC does not effectively adsorb mixtures with high saturation vapor pressures and low boiling points, and adsorbates with high saturation vapor pressures quickly volatilize. The AC adsorption process is dynamic. Adsorbed vapor can desorb during the adsorption process, making stabilization of volatile adsorbates in pore spaces difficult. This significantly decreases the adsorption capacity of AC for mixtures with high saturation vapor pressures.
Calculation of adsorption energy
(CitationLiang, 2008; CitationCeng et al., 2004; CitationGao et al., 2001; CitationDastgheib et al., 2005; CitationWood, 2001; CitationWu et al., 2002). An adsorption force field exists on the adsorption surface of AC, and during the adsorption process, it generates an adsorption potential that is determined by the adsorbent, the adsorbate, and the distance between the adsorbate molecule and the adsorbent surface. The Dubinin-Radushkevich (D-R) equation adequately captures the adsorption behavior of AC. The characteristic adsorption energy E 0 can be determined by using the D-R equation to linearly fit the adsorption isotherm. The D-R equation has the following form (CitationDubinin, 1989):
A linear correlation of the nitrogen adsorption isotherm for the three types of AC was determined according to the D-R equation, and the relationship between ln2 (P 0/P) and ln W was obtained. As shown in , the slope of the fitting curve was 2.303(RT/βE 0)2, and the affinity coefficient of nitrogen was 0.33. With this information, the characteristic adsorption energies of AC-1, AC-2, and AC-3 were calculated to be 17.18, 17.3, and 16.55 kJ·mol−1, respectively. The affinity coefficients of toluene, acetone, and xylene were 1.28, 0.88, and 1.30, respectively. The adsorption energies are listed in , and these results correspond with experimental results.
Table 5. Adsorption energy of AC (kJ·mol−1)
Conclusions
1. | The surface areas and pore volumes of three types of AC were measured, and the results showed that the pores of these ACs were mainly micropores, but macropores and mesopores were also found. The distribution of pore diameters was wide. Results from infrared spectrometer and Boehm titration tests showed that surface groups in the new AC were mostly free. | ||||
2. | For the same type of AC, the saturation times and adsorption capacities of the three adsorbates had the following order: acetone < toluene < xylene. For different types of AC, the saturation times and adsorption capacities of individual adsorbates were different. | ||||
3. | Different functional groups corresponding to adsorbate properties influenced the adsorptive properties of AC differently. The carboxyl and total basic groups primarily affected the adsorptive properties of toluene and xylene, whereas the lactone and total acidic groups primarily affected the adsorptive properties of acetone. There was no significant correlation between the phenolic hydroxyl group and the adsorbates. | ||||
4. | The saturated adsorption capacity of AC for organic vapor increased linearly as the adsorbate molecular weight, concentration, boiling point, and dynamic diameter increased, but it decreased linearly as the polarity and saturation vapor pressure increased. | ||||
5. | The nitrogen adsorption isotherm was calculated according to the D-R equation before the adsorption energy of AC was calculated. For adsorption onto AC, the adsorption energies of the three adsorbates had the following order: xylene > toluene > acetone. |
Summary
The adsorption behavior of activated carbon based on adsorbate properties was researched. The different groups have different influence on the adsorptive properties of ACs. The adsorption capacity of activated carbon increases with the increase of adsorbate molecular weight, dynamic diameter, boiling point, and density, and that this relationship is linear, and the adsorption capacities and adsorption energies of three kinds of activated carbon for these three adsorbates had the following order: xylene > toluene > acetone.
Acknowledgments
The research is supported by the National Natural Science Foundation of China (20676154, 20976200), the MOST of China APEC Cooperation Funds, and the Fundamental Research Funds for the China Central Universities.
References
- Ao , C.H. and Lee , S.C. 2005 . Indoor air purification by photocatalyst TiO2 immobilized on an activated carbon filter installed in an air cleaner . Chem. Eng. Sci , 60 : 103 – 109 . doi: 10.1016/j.ces.2004.01.073
- Byeon , J.H. , Park , J.H. , Yoon , K.Y. , Ko , B.J. , Ji , J.H. and Hwang , J. 2006 . Removal of volatile organic compounds by spark generated carbon aerosol particles . Carbon , 44 : 2106 – 2108 . doi: 10.1016/j.carbon.2006.03.033
- Ceng , Z.W. , Zeng , H.C. , Zhang , P.Y. , Xu , L.S. and Zhu , Q.L. 2004 . Surface physical and chemical characteristics of PAN-ACF and its adsorption energy . Electric Power Environ. Protect , 20 : 57 – 59 . doi: CNKI:SUN:DLHB.0.2004-03-019
- Chen , J.M. and Zhang , J. 2009 . Application of ASAP2020 specific surface area and porosity analyze . Anal. Instrum , 3 : 61 – 64 . doi: CNKI:SUN:FXYQ.0.2009-03-017
- Chiang , Y.C. , Chiang , P.C. and Huang , C.P. 2001 . Effects of pore structure and temperature on VOC adsorption on activated carbon . Carbon , 39 : 523 – 534 . doi: 10.1016/S0008-6223(00)00161-5
- Cosnier , F. , Celzard , A. , Furdin , G. , égin , D. B , Marêché , J.F. and Barrès , O. 2005 . Hydrophobisation of active carbon surface and effect on the adsorption of water . Carbon , 43 : 2554 – 2563 . doi: 10.1016/j.carbon.2005.05.007
- Daifullah , A.A.M. and Girgis , B.S. 2003 . Impact of surface characteristics of activated carbon on adsorption of BTEX . Colloids Surf. A Physicochem. Eng. Aspects , 214 : 181 – 193 . doi: 10.1016/S0927-7757(02)00392-8
- Dastgheib , S.A. and Karanfil , T. 2005 . The effect of the physical and chemical characteristics of activated carbons on the adsorption energy and affinity coefficient of Dubinin equation . J. Colloid Interface Sci , 292 : 312 – 321 . doi: 10.1016/j.jcis.2005.06.017
- Dubinin , M.M. 1989 . Fundamentals of the theory of adsorption in micropores of carbon adsorbents: Characteristics of their adsorption properties and microporous structures . Carbon , 27 : 457 – 467 . doi: 10.1016/0008-6223(89)90078-X
- Gao , H.S. , Wang , D.H. , Ye , Y.C. and Tan , T.E. 2001 . Modified Polanyi-Dubinin equation to correlate adsorption equilibrium of VOC-water vapor mixtures on activated carbon . J. Chem. Industry Eng. (China) , 52 : 357 – 362 . doi: CNKI:SUN:HGSZ.0.2001-04-015
- Gong , G.Z. , Xie , Q. , Zheng , Y.F. , Ye , S.F. and Chen , Y.F. 2009 . Regulation of pore size distribution in coal-based activated carbon . New Carbon. Mater , 24 : 141 – 145 . doi: 10.1016/S1872-5805(08)60043-8
- Hsieh , C.T. and Chen , J.M. 2002 . Adsorption energy distribution model for VOCs onto activated carbons . J. Colloid Interface Sci , 255 : 248 – 253 . doi: 10.1006/jcis.2002.8668
- Lee , S.W. , Cheon , J.K. , Park , H.J. and Lee , M.G. 2008 . Adsorption characteristics of binary vapors among acetone, MEK, benzene, and toluene . Korean J. Chem. Eng , 25 : 1154 – 1159 . doi: 10.1007/s11814-008-0190-3
- Li , B.Z. , Liu , Z.Y. , Lei , Z.P. and Huang , Z.G. 2010 . Effect of structure and properties of simple aromatic compounds on adsorption behavior of activated carbon . J. Fuel Chem. Technol , 38 : 252 – 256 .
- Liang , D.M. 2008 . China Coal Activated Carbon , Beijing : Chemical Industry Press .
- Lillo-Ródenas , M.A. , Cazorla-Amorós , D. and Linares-Solano , A. 2005 . Behaviour of activated carbons with different pore size distributions and surface oxygen groups for benzene and toluene adsorption at low concentrations . Carbon , 43 : 1758 – 1767 . doi: 10.1016/j.carbon.2005.02.023
- Lillo-Ródenas , M.A. , Fletcher , A.J. , Thomas , K.M. , Cazorla-Amorós , D. and Linares-Solano , A. 2006 . Competitive adsorption of a benzene-toluene mixture on activated carbons at low concentration . Carbon , 44 : 1455 – 1463 . doi: 10.1016/j.carbon.2005.12.001
- Lu , C.Y. and Wey , M.Y. 2007 . Simultaneous removal of VOC and NO by activated carbon impregnated with transition metal catalysts in combustion flue gas . Fuel Process. Technol , 88 : 557 – 567 . doi: 10.1016/j.fuproc.2007.01.004
- Maštovská , K. and Lehotay , S.J. 2004 . Evaluation of common organic solvents for gas chromatographic analysis and stability of multiclass pesticide residues . J. Chromatogr. A , 1040 : 259 – 272 . doi: 10.1016/j.chroma.2004.04.017
- Wood , G.O. 2001 . Affinity coefficients of the Polanyi/Dubinin adsorption isotherm equations: A review with compilations and correlations . Carbon , 39 : 343 – 356 . doi: 10.1016/S0008-6223(00)00128-7
- Wu , J.F. , Strömqvist , M.E. , Claesson , O. , ängmark , I.E. F and Hammarström , L.G. 2002 . A systematic approach for modelling the affinity coefficient in the Dubinin–Radushkevich equation . Carbon , 40 : 2587 – 2596 . doi: 10.1016/S0008-6223(02)00173-2
- Yang , T.Z. , Luo , S.Z. and Xu , Y.S. 2006 . Characterization of pore structure based on N2 adsorption applied to porous materials . Carbon (China) , 1 : 17 – 22 .