Abstract
Multispectral photoacoustic instruments are commonly used to measure aerosol and nitrogen dioxide (NO2) light absorption coefficients to determine the radiation budget of the atmosphere. Here a new photoacoustic system is developed to explore the effect of photolysis on the measured signal in a multispectral photoacoustic spectrometer. In this system, a 405-nm laser is used primarily as light source for photolysis. Additionally, a well-overlapped 532-nm laser, modulated at the resonant frequency of the photoacoustic instrument, is used to probe the NO2 concentration. As a result, the photolysis effect at 405 nm can be observed by the photoacoustic instrument through the 532-nm laser. This work determines an 11% reduction of the photoacoustic signal caused by the photolysis effect for typical conditions, which needs to be taken into account when calibrating multispectral photoacoustic spectrometers with NO2.
Multispectral photoacoustic instruments are commonly used to measure aerosol and nitrogen dioxide (NO2) light absorption coefficients to determine the radiation budget of the atmosphere. A 405-nm laser is often used in these multispectral photoacoustic instruments. Although NO2 absorbs strongly at 405 nm, it also has a strong photolysis pathway that is accessible by light of the same wavelength. Photolysis reduces the photoacoustic signal, necessitating special care when interpreting photoacoustic measurements. This paper offers a method for the multispectral photoacoustic instrument user to quantify the influence of the 405-nm NO2 photolysis effect on the photoacoustic signal.
Introduction
The optical properties of aerosol and gases are important factors for atmospheric radiation transfer. They affect the lifetime of clouds and the global and regional radiation balance and they dominate the uncertainties in total global radiative forcing (CitationHorvath, 1993; CitationMoosmüller et al., 2009; CitationLohmann and Feichter, 2005). Specifically, nitrogen dioxide (NO2) light absorption can play an important role in the ultraviolet (UV) and visible radiation budget of the atmosphere (Intergovernmental Panel on Climate Change [CitationIPCC], 2007; CitationVasilKov et al., 2009). Photoacoustic measurements can be used for the sensitive characterization of NO2 absorption coefficients and concentrations (e.g., CitationTerhune and Anderson, 1977; CitationSlezak et al., 2003); in addition, NO2 is commonly used to calibrate photoacoustic measurements of aerosol light absorption (CitationAdams et al., 1989; CitationArnott et al., 2000). For all of these applications, absorption and photolysis of NO2 need to be better understood.
The photolysis reaction can be separated into three distinct wavelength regions based on the behavior of quantum yield (molecules photon−1) and photolysis mechanism as a function of wavelength (CitationJones and Bayes, 1973). The first region is the short wavelength region (295 nm < λ < 398 nm) where the photon energy is higher than the photodissociation energy (398 nm for reaction represented by Equationeq 1) with a primary quantum yield of unity. The mechanism, which is described by Equationeq 1 and Equationeq 2, predicts a quantum yield of 2 for nitric oxide (NO) formation, which is close to the values observed (CitationLeighton, 1961).
In the second wavelength region (398 nm < λ < 430 nm), the photon energy is less than the dissociation energy and the quantum yield falls off rapidly with wavelength. Because very few of the NO2 molecules are vibrationally excited, most of their internal energy is rotational energy. The photolysis in this spectral region has been attributed to the utilization of rotational energy and thermal energy (transferred from molecular collision) to make up the difference between dissociation energy and photon energy (CitationJones and Bayes, 1973; CitationCreel and Ross, 1976). As the wavelength is increased beyond about 430 nm, into the third wavelength region (430 nm < λ < 630 nm), internal energy and relative kinetic energy cannot offer sufficient additional energy to break the NO2 molecule. In this region, photolysis is caused by the reaction of an electronically excited NO2 molecule with a ground state NO2 molecule, as shown in Equationeq 3 (Norrrish, 1929a, 1929b), or by an alternative reaction shown in Equationeq 4 (CitationFord and Jaffe, 1963).
The photoacoustic effect can be used to quantify the transfer of radiative energy to heat, in many cases enabling the direct measurement of absorption coefficients (CitationMoosmüller et al., 2009). Photoacoustic instruments have been used since 1973 to study photolysis of NO2 by comparing extinction and photoacoustic spectra of NO2 (CitationHarshbarger and Robin, 1973). The original system developed by Harshbarger and Robin () used a 4-kW xenon lamp as the light source, a chopper to modulate the incident light, a monochromator for wavelengh scanning, a microphone, preamplifier, and lock-in amplifier to obtain the acoustic signal, and a radiometer to measure the optical power of the monochrometer output beam. As shown in , the general features of the two spectra look quite similar between 4100 Å (410 nm) and 7000 Å (700 nm). However, below 410 nm, the spectrophone (i.e., photoacoustic) spectrum shows a sharp drop in signal strength that is not observed in the optical spectrum. This abrupt loss of part of the photoacoustic signal is due to NO2 photolysis. Harshbarger and Robin's research did not quantify the photolysis effect, although their work demonstrated that photoacoustic instrument can be used in the measurement and understanding of photolysis.
Figure 2. Comparison of the optical extinction and spectrophone (i.e., photoacoustic) spectra of NO2 at 1333 Pa pressure (Harshbarger et al., 1973).
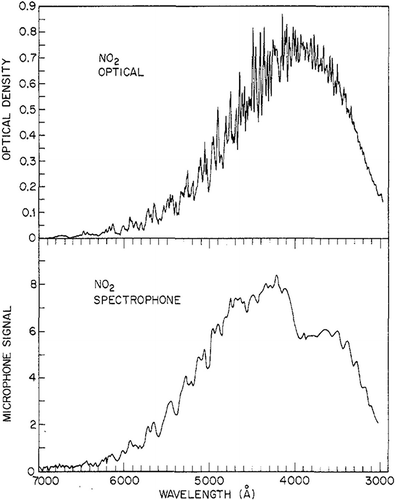
Further research has been done by CitationLewis (2007), who quantified the photolysis effect of NO2 in association with the calibration of a photoacoustic instrument at 405 nm, as shown in The ratio between the extinction and apparent photoacoustic absorption coefficients was used to quantify the photolysis effect of NO2, since at this wavelength extinction equals absorption, because Rayleigh scattering is negligible when compared with extinction. The apparent photoacoustic absorption coefficient is significantly lower than the extinction coefficient because part of the laser energy is used to break NO2 molecules rather than to heat the surrounding air. However, for a multispectral photoacoustic instrument, this research is not sufficient to quantify the photolysis of NO2, as explained at the end of the following paragraph.
Figure 3. NO2 gas calibration results at 405 nm from CitationLewis (2007).
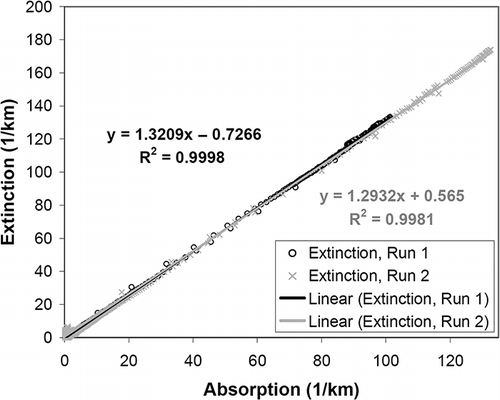
Multispectral photoacoustic instruments and wide-spectral-range photoacoustic spectrometers are now available and are used to measure light absorption by aerosol and gases (CitationHaisch et al., 2012; CitationAjtai et al., 2010; CitationLewis et al., 2008). A 405-nm laser is often included in the multispectral photoacoustic instruments. For example, a dual-wavelength photoacoustic instrument operating at 405 and 870 nm was used during the 2006 Fire Lab at Missoula Experiment to measure light scattering and absorption coefficients by smoke from the combustion of a variety of biomass fuels (CitationLewis et al., 2008), a three-wavelength (405, 532, and 781 nm) photoacoustic instrument was used for studying optical-chemical-microphysical relationships and for closure studies (CitationFlowers et al., 2010), and a four-wavelength (266, 355, 532, and 1064 nm) photoacoustic instrument was used for intercomparisan with a multiwavelength aethalometer under suburban, wintry conditions (CitationAjtai et al., 2011). Because the NO2 photolysis effect around 400 nm is strong (CitationJones and Bayes, 1973), it needs to be accounted for when calibrating multispectral photoacoustic instruments with NO2. Although NO2 photolysis does not prevent those multispectral photoacoustic instruments from being used for NO2 light absorption measurements, the performance of the NO2 light absorption measurements must be quantified and improved by characterization of the photolysis effect. Lewis’ (2007) results are not sufficient to quantify the photolysis effect for multispectral photoacoustic instruments because they were obtained at a single wavelength. In multispectral photoacoustic instruments, multiple lasers are generally operated simultaneously at different modulation frequencies and the acoustic signals are separated according modulation frequency using fast Fourier transform. As a reference, shows the absorption spectrum in terms of absorption cross-section of NO2. In such instruments, coupled photolysis, which is caused by multiple laser wavelengths and the coactions of them with multiphoton absorption (CitationHakala et al., 1974), has effects on acoustic signals from all lasers. Although the photoacoustic signal from each laser can be calibrated individually by using Lewis’ method to correct for the photolysis effect caused by that laser itself, the coupled photolysis also needs to be taken into account.
Figure 4. Absorption cross-section spectrum of NO2 at 294 K (CitationVandaele, 1998).
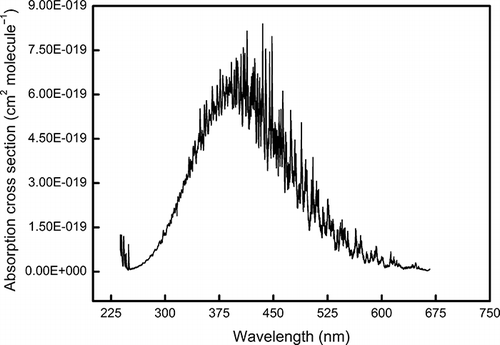
This paper describes a novel photoacoustic system to quantify the photolysis effect in NO2 light absorption measurements using multispectral photoacoustic instruments.
Experimental Setup
The experimental setup shown in consists of two lasers, a photoacoustic resonator, a piezoelectric transducer, data acquisition electronics, and a computer. The piezoelectric transducer is used to determine the resonant frequency of the photoacoustic resonator. Beams of a 532-nm laser (123 mW) and a 405-nm laser (180 mW) are sent through the photoacoustic resonator to generate the microphone signals used as input for two lock-in amplifiers at the modulation frequency of the two laser beams. Lock-in amplifier 1 controls a chopper that modulates the 532-nm laser at the resonant frequency of the photoacoustic instrument, lock-in amplifier 2 controls modulation of the 405-nm laser. We can also manually modulate the 405-nm laser at very low modulation frequency (lower than 0.1 Hz). The laser power for each laser is measured by inserting a power meter during instrument calibration (not shown in ).
Figure 5. Schematic diagram of the photoacoustic instrument, including two lasers, chopper, photoacoustic resonator with microphone and piezoelectric transducer, two lock-in amplifiers, and personal computer (PC) (color figure available online).
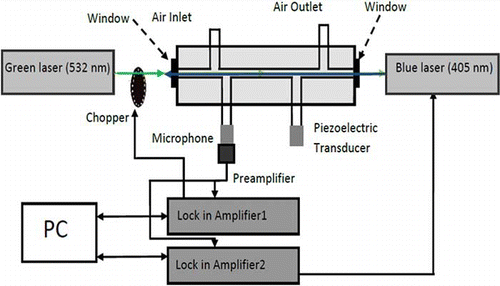
Light absorption of NO2 at 532 nm can be detected using the microphone because the 532-nm laser is chopped at the resonant frequency of the photoacoustic instrument. Light absorption of NO2 at 405 nm does not produce a detectable photoacoustic signal because the modulation frequency of the 405-nm laser is far away from the acoustic resonance frequency. When the 405-nm laser is turned on, it causes NO2 photolysis, which changes the absorption of the 532-nm laser beam because the two laser beams are well overlapped, as shown schematically in This change in absorption of the 532-nm radiation can clearly be observed in the changed microphone signal.
Calibration of the Photoacoustic Instrument at 532 nm
The system is calibrated at 532 nm by using sufficiently high concentrations of NO2 in the resonator to obtain both the extinction (from gas transmissivity) and absorption (photoacoustic measurement) coefficients. A photodiode is placed to measure the transmissivity during the calibration. Absorption is sufficiently large that Rayleigh scattering can be ignored. The system was calibrated using Beer's Law,
Figure 6. NO2 calibration of the photoacoustic instrument at 532 nm. For NO2, the absorption coefficient is equal to the extinction coefficient. The measurement with the uncalibrated instrument (shown as gray solid line) is adjusted by multiplying with a constant, yielding the calibrated absorption coefficient, shown as gray dashed line.
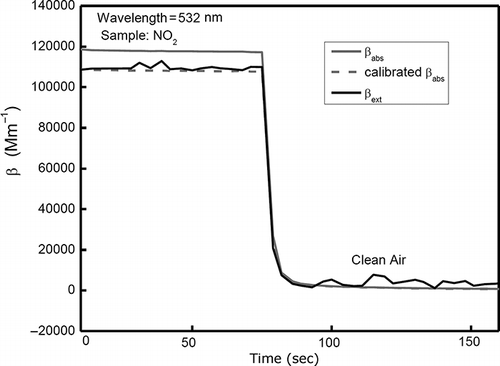
Measurements
Monitoring 405 nm NO2 photolysis with photoacoustic measurements at 532 nm
Once the photoacoustic system is calibrated at 532 nm, the photodiode used during the calibration is removed and the 405-nm laser is setup to be the photolysis light source, as shown in A sample with 330 ppm of NO2 in air is introduced into the photoacoustic instrument continually with a flow rate of 1 liter per minute. The absorption coefficient of the sample in the photoacoustic instrument decreases due to photolysis when turning on the 405-nm laser and returns to the original value after turning off the 405-nm laser, once the sample in the resonator has been completely replaced by the continuous flow. After the 405-nm laser is turned on, it takes ∼4 sec to obtain dynamic equilibrium between photolytic destruction of NO2 and replacement with undepleted NO2, as shown in The absorption coefficient increases when the 405-nm laser is turned off and decreases when the 405-nm laser is turned on due to NO2 photolysis at 405 nm.
Figure 7. Short-term measurement of the sample absorption coefficient at 532 nm with 405-nm laser on and off.
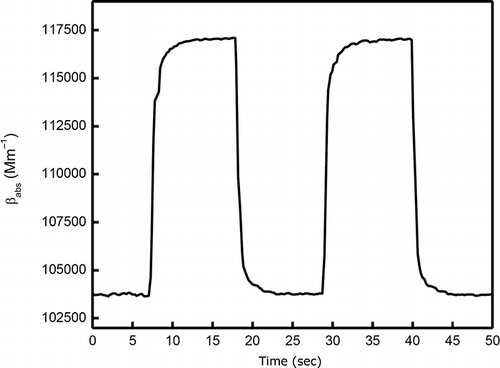
A more long-term measurement is shown in , with two dashed lines indicating the absorption coefficient of the sample at two different equilibrium states. The change of the sample absorption coefficient at 532 nm can be observed clearly from the photoacoustic signal while switching on and off the 405-nm laser manually. The difference in absorption coefficient between the two equilibrium states is ∼11%.
Figure 8. Photolysis of NO2 caused by the 405-nm laser. The line shows the change of the sample absorption coefficient at 532 nm due to the photolysis of NO2 caused by the 405-nm laser with 123 mW laser power. The difference between absorption coefficients and NO2 concentrations with the 405-nm laser turned on and off is ∼11%.
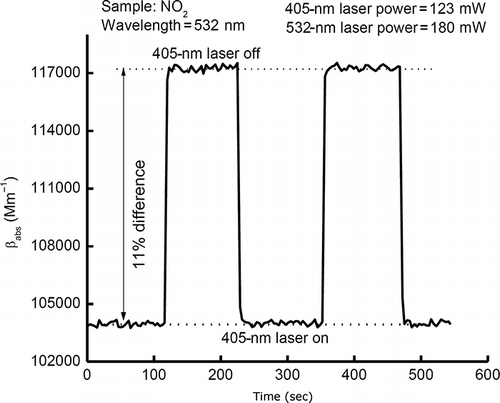
Multispectral photoacoustic instruments are operated at the resonance frequency of their acoustic resonator, which for our instruments is ∼1500 Hz. To determine the effect of NO2 photolysis on the operation of a dual-wavelength photoacoustic instrument operating at 405 and 532 nm, the 405-nm laser in our system is modulated at a high frequency (i.e., 1000 Hz) and the change of absorption of the continuously flowing sample is observed with the 532-nm laser. As observed in , the 532-nm light absorption coefficient measured with the 405-nm laser, power-modulated at 1000 Hz, is about the average of the absorption coefficients measured with the 405-nm laser turned off (i.e., the highest values of ∼80,000 Mm−1 shown in ) and that with the 405-nm laser continuously operating (i.e., the lowest values of ∼73,000 Mm−1 shown in ). When our setup is operated with the 405-nm laser modulation frequency larger than 20 Hz, the absorption coefficient is very stable. The reduction in 532-nm absorption and NO2 concentration is solely dependent on the average power of the 405-nm laser.
Monitoring 532-nm-induced NO2 concentration change with photoacoustic measurements at 405 nm
After exchanging the roles of the 405-nm laser and the 532-nm laser, the 532-nm laser is turned on and off, while the 405-nm laser is used for photoacoustic monitoring of the NO2 concentration. The resulting change in sample absorption coefficient between the 532-nm laser turned on and off was ∼2%, as shown in The 532-nm wavelength is located in the third photolysis region, as discussed in Introduction. It is commonly assumed that the quantum yield for NO production from NO2 photolysis is lower than 1% at 532 nm and is ∼30% at 405 nm (CitationJones and Bayes, 1973; CitationCreel and Ross, 1976), which wouldn't explain the strong signal seen in
Figure 10. Change of sample light absorption coefficient measured at 405 nm. The difference between 405-nm absorption coefficients with the 532-nm laser turned on and off is ∼2%.
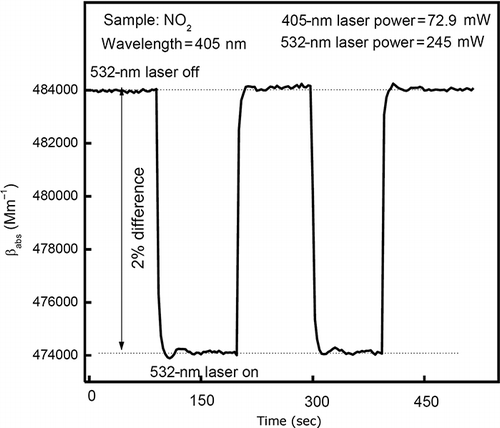
Further qualitative information comes from measurements from an NO analyzer (Thermo 42C NO-NO2-NOX Analyzer) that was connected to the output of the photoacoustic resonator to measure NO concentrations created by photolysis of NO2. For a sample gas containing 330 ppm NO2 in air, the 405-nm photolysis of NO2 is sufficiently strong that the analyzer is saturated when only the 405-nm laser is turned on, as shown in
Figure 11. NO concentration change measured by the NO analyzer. Photolysis of NO2 at 405 nm was sufficiently strong that the NO analyzer was saturated at its upper limit of 20,000 ppb by the NO produced.
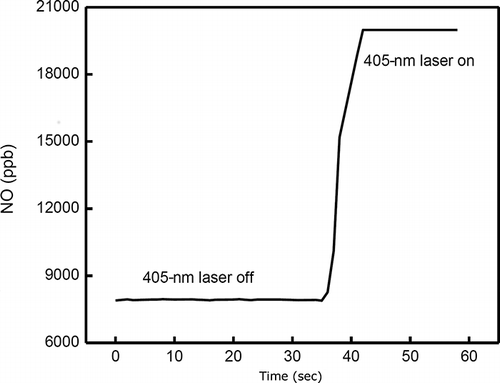
The same method was used to check if there is any photolysis of NO2 at 532 nm by turning the 532 nm on and off (only the 532-nm laser is used). The NO analyzer did not show a meaningful signal of NO concentration. It is speculated that the 2% concentration difference () may be caused by the following:
1. | Multiphoton absorption and photolysis involving coactions of the 405- and 532-nm radiation. Multiphoton absorption and photolysis phenomena for NO2 have been previously demonstrated by others (CitationHakala et al., 1974). | ||||
2. | Sequential photon absorption (rather than simultaneous multiphoton absorption). Some intermediate states are sufficiently long lived, for example, a relaxation time as high as 4 µsec has been reported for the exited state 2B2 (CitationKalkman and Kesteren, 2008). | ||||
3. | Temperature effects influence the implicit photochemistry within the laser beams. Absorption cross-section and photolysis yields are affected by temperature (CitationBurrows et al., 1998; CitationRoehl, 1994). When the 532-nm laser is turned on, the temperature within the laser beam increases, thereby increasing the absorption cross-section and photolysis yield and consequently lowering the NO2 concentration in the laser beam. |
Discussion
Two main sources of error need to be considered for high-sensitivity photoacoustic detection of NO2. First, due to photolysis and photochemical reaction (CitationJones and Bayes, 1973), ozone can be produced in the resonant cavity of the photoacoustic instrument. The absorption of ozone at 532 nm will increase the photoacoustic signal and decrease the measured photolysis effect. Because the ozone absorption cross-section is about 50 times smaller than that of nitrogen dioxide (CitationOrphal, 2003), this decrease is small when the ozone concentration is low. Second is the relaxation effect of the measured molecules, reported by Kalkman and Kesteren (2008) in a high-sensitivity photoacoustic detection of NO2 at 444 nm. Their research shows that in detection of NO2 in N2, the relaxation does not affect the results. However, a signal decrease is observed when oxygen is mixed into the gas mixture. The relaxation time can be described by the phase shift of photoacoustic signal. The signal is directly proportional to the cos θ (CitationMoosmüller et al., 2009), where θ is the phase shift of photoacoustic signal.
In our research, the phase shift of photoacoustic signal that represents the concentration of NO2 is measured. An 8° phase shift at 532 nm and an 11° phase shift at 405 nm were observed. These phase shifts are relatively small. The effect on the measured concentration is less than 1.2%.
Conclusion
A new method is developed to quantify the photolysis effect in NO2 using multispectral photoacoustic instruments and the effect on calibrating such instruments with NO2. A photoacoustic instrument with continuous flow is used to measure the NO2 photolysis effect using two overlapped lasers. In this experiment, which simulates the operation of multispectral photoacoustic instruments, a maximum 11% reduction of the 532-nm photoacoustic signal is due to the photolysis effect observed at 405 nm, and a 2% reduction of the 405-nm photoacoustic signal is observed due to the simultaneous presence of 532-nm radiation. For typical operating conditions of a multispectral photoacoustic instrument, half of these reductions are expected because of the high-frequency modulation and 50% duty cycle of the laser. The reduced photoacoustic signal at 405 nm due to the presence of the 532-nm beam is not fully understood but may be due to multiphoton processes involving simultaneous 405- and 532-nm radiation.
Acknowledgment
This paper is based upon work supported by NASA EPSCoR under Cooperative Agreement No. NNX10AR89A, by NASA ROSES under grant no. NNX11AB79G, and by the National Science Foundation under grant no. AGS-1040046. Helpful discussions with Dean Venables are gratefully acknowledged.
References
- Adams , K.M. , Davis , L.I. Jr. , Japar , S.M. and Pierson , W.R. 1989 . Real-time, in situ measurement of atmospheric optical absorption in the visible via photoacoustic spectroscopy—II. Validation for atmospheric elemental carbon aerosol . Atmos. Environ. , 23 : 693 – 700 . doi: 10.1016/0004-6981(89)90017-6
- Ajtai , T. , Filep , Á. , Schnaiter , M. , Linke , C. , Vragel , M. , Bozóki , Z. , Szabó , G. and Leisner , T. 2010 . A novel multi-wavelength photoacoustic spectrometer for the measurement of the UV-Vis-NIR spectral absorption coefficient of atmospheric aerosols . J. Aerosol Sci. , 41 : 1020 – 1029 . doi: 10.1016/j.jaerosci.2010.07.008
- Ajtai , T. , Filep , Á. , Utry , N, , Schnaiter , M. , Linke , C. , Bozóki , Z. , Szabo , G. and Leisner , T. 2011 . Inter-comparison of optical absorption coefficients of atmospheric aerosols determined by a multi-wavelength photoacoustic spectrometer and an aethalometer under sub-urban wintry conditions . J. Aerosol Sci. , 42 : 859 – 866 . doi: 10.1016/j.jaerosci.2011.07.008
- Arnott , W. P. , Moosmüller , H. and Walker , J. W. 2000 . Nitrogen dioxide and kerosene-flame soot calibration of photoacoustic instruments for measurement of light absorption by aerosols . Rev. Sci. Instrum. , 71 : 4545 – 4552 . doi: 10.1063/1.1322585
- Burrows , J. P. , Dehn , A. , Dehn , B. , Himmelmann , S. , Richter , A. , Voigt , S. and Orphal , J. 1998 . Atmospheric remote sensing reference data from GOME: Part 1. Temperature-dependent absorption cross section of NO2 in the 231–794 nm range . J. Quant. Spectroc. Radiat. Transfer , 60 : 1025 – 1031 . doi: 10.1016/S0022-4073(97)00197-0
- Creel , C. L. and Ross , J. 1976 . Photodissociation of NO2 in the region 458–630 nm . J. Chem. Phys. , 64 : 3560 – 3565 . doi: 10.1063/1.432725
- Flowers , B. A. , Dubey , M. K. , Mazzoleni , C. , Stone , E. A. , Schauer , J. J. , Kim , S.-W. and Yoon , S. C. 2010 . Optical-chemical-microphysical relationships and closure studies for mixed carbonaceous aerosols observed at Jeju Island; 3-laser photoacoustic spectrometer, particle sizing, and filter analysis . Atmos. Chem. Phys. , 10 : 10387 – 10389 . doi: 10.5194/acp-10-10387-2010
- Ford , H. W. and Jaffe , S. 1963 . Photolysis of nitrogen dioxide at 3660 and 4047 Å at 25°C . J. Chem. Phys. , 38 : 2935 – 2942 . doi: 10.1063/1.1733623
- Hakala , D. , Harteck , P. and Reeves , R. R. 1974 . Dual photon effects in nitrogen dioxide photolysis . J. Phys. Chem. , 78 : 1583 – 1586 . doi: 10.1021/j100609a003
- Haisch , C. , Menzenbach , P. , Bladt , H. and Niessner , R. 2012 . A wide spectral range photoacoustic aerosol absorption spectrometer . Anal. Chem. , 84 : 8941 – 8945 . doi: 10.1021/ac302194u
- Harshbarger , W. R. and Robin , M. B. 1973 . The opto-acoustic effect: Revival of an old technique for molecular spectroscopy . Acc. Chem. Res. , 6 : 329 – 334 . doi: 10.1021/ar50070a001
- Horvath , H. 1993 . Atmospheric light absorption—A review . Atmos. Environ. , 27 : 293 – 317 . doi: 10.1016/0960-1686(93)90104-7
- Intergovernmental Panel on Climate Change . 2007 . IPCC Fourth Assessment Report: Climate Change 2007 . Working Group I Report “The Physical Science Basis. ,
- Jones , I. T. N. and Bayes , K. D. 1973 . Photolysis of nitrogen dioxide . J. Chem. Phys. , 59 : 4836 – 4844 . doi: 10.1063/1.1680696
- Kalkman , J. and Van Kesteren , H.W. 2008 . Relaxation effects and high sensitivity photoacoustic detection of NO2 with a blue laser diode . Appl. Phys. B , 90 : 197 – 200 . doi: 10.1007/s00340-007-2895-0
- Leighton , P.A. 1961 . Photochemistry of Air Pollution , 52 – 59 . New York : Academic Press .
- Lewis , K. A. 2007 . “ Development of a dual-wavelength photoacoustic instrument for measurement of light absorption and scattering by aerosol and gases. Ph.D. dissertation ” . In Atmospheric Science Program, Department of Physics , Reno, Reno , NV : University of Nevada .
- Lewis , K. , Arnott , W. P. , Moosmüller , H. and Wold , C. E. 2008 . Strong spectral variation of biomass smoke light absorption and single scattering albedo observed with a novel dual-wavelength photoacoustic instrument . J. Geophys. Res. , 113 : D16203 doi: 1029/2007JD009669
- Lohmann , U. and Feichter , J. 2005 . Global indirect aerosol effects: A review . Atmos. Chem. Phys. , 5 : 715 – 737 . doi: 10.5194/acp-5-715-2005
- Moosmüller , H. , Chakrabarty , R. K. and Arnott , W. P. 2009 . Aerosol light absorption and its measurement: A review . J. Quant. Spectrosc. Radiat. Transfer , 110 : 844 – 878 . doi: 10.1016/j.jqsrt.2009.02.035
- Norrish , R. G. W. 1929a . CLIV.—Photochemical equilibrium in nitrogen peroxide. Part II. The dependence of quantum efficiency on wavelength . J. Chem. Soc. , 1929 : 1158 – 1169 . doi: 10.1039/jr9290001158
- Norrish , R. G. W. 1929b . CCXI.—Photochemical equilibrium in nitrogen peroxide. Part IV. The relation between fluorescence and photochemical action . J. Chem. Soc. , 1929 : 1611 – 1621 . doi: 10.1039/jr9290001611
- Roehl , C. M. , Orlando , J. J. , Tyndall , G. S. , Shetter , R. E. , Vásquez , G. J. , Cantrell , C. A. and Galvert , J. G. 1994 . Temperature dependence of the quantum yields for the photolysis of NO2 near the dissociation limit . J. Phys. Chem. , 98 : 7837 – 7843 . doi: 10.1021/j100083a015
- Slezak , V. , Codnia , J. , Peuriot , A. L. and Santiago , G. 2003 . Resonant photoacoustic detection of NO2 traces with a Q-switched green laser . Rev. Sci. Instrum. , 74 : 516 – 518 . doi: 10.1063/1.1518570
- Terhune , R. W. and Anderson , J. E. 1977 . Spectrophone measurements of the absorption of visible light by aerosols in the atmosphere . Opt. Lett. , 1 : 70 – 72 . doi: 10.1364/OL.1.000070
- Solomon, S., D. Qin, M. Manning, R.B. Alley, T. Berntsen, N.L. Bindoff, Z. Chen, A. Chidthaisong, J.M. Gregory, G.C. Hegerl, et al. 2007. Technical Summary. In Climate Change 2007: The Physical Science Basis. Contribution of Working Group I to the Fourth Assessment Report of the Intergovernmental Panel on Climate Change, eds. S. Solomon, D. Qin, M. Manning, Z. Chen, M. Marquis, K.B. Averyt, M. Tignor and H.L. Miller. Cambridge, UK: Cambridge University Press
- Orphal , J. 2003 . A critical review of the absorption cross-sections of O3 and NO2 in the ultraviolet and visible . J. Photochem. Photobiol. A Chem. , 157 : 185 – 209 . doi: 10.1016/S1010-6030(03)00061-3
- Vandaele , A. C. , Hermans , C. , Simon , P. C. , Carleer , M. , Cloin , R. , Fally , S. , Mérienne , M. F. , Jenouvirier , A. and Coquart , B. 1998 . Measurements of the NO2 absorption cross-section from 42000 cm−1 to 10000 cm−1 (238–1000 nm) at 220 K and 294 K . J. Quant. Spectrosc. Radiat. Transfer , 59 : 171 – 184 . doi: 10.1016/S0022-4073(97)00168-4
- VasilKov , A. P. , Joiner , J. , Oreopoulos , L. , Gleason , J. F. , VeefKind , P. , Bucsela , E. , Celarier , E. A. , Spurr , R. J. D. and Platnick , S. 2009 . Impact of tropospheric nitrogen dioxide on the regional radiation budget . Atmos. Chem. Phys. , 9 : 6389 – 6400 . doi: 10.5194/acp-9-6389-2009