Abstract
The management of landfill leachate is challenging, with relatively limited work targeting high-strength leachate. In this study, the performance of the membrane bioreactor (MBR) and sequencing batch reactor (SBR) technologies are compared in treating high-strength landfill leachate. The MBR exhibited a superior performance with removal efficiencies exceeding 95% for BOD5, TN, and NH3 and an improvement on SBR efficiencies ranging between 21 and 34%. The coupled experimental results contribute in filling a gap toward improving the management of high-strength landfill leachate and providing comparative guidelines or selection criteria and limitations for MBR and SBR applications.
Implications
While the sequencing batch reactor (SBR) technology offers some flexibility in terms of cycle time and sequence, its performance is constrained when considering landfill leachate associated with significant variations in quality and quantity. Combining membrane separation and biodegradation processes or the membrane bioreactor (MBR) technology improved removal efficiencies significantly. In the context of leachate management using the MBR technology, more efforts have targeted low-strength leachate with limited attempts at moderate to high strength leachate. In this study, the SBR and MBR technologies were tested under different operating conditions to compare and evaluate their feasibility for the management of high-strength leachate from a full-scale operating landfill. Such a comparison has not been reported for high-strength leachate.
Introduction
Controlled landfilling remains the most economic and commonly practiced method for municipal solid waste (MSW) disposal, with leachate generation being an inevitable consequence. Leachate is formed when the refuse moisture content exceeds its field capacity, which is defined as the maximum moisture that is retained in a porous medium without producing downward percolation. The quantity of leachate formed at a landfill is influenced by factors that contribute to landfill moisture (such as rainfall, snowmelt, groundwater intrusion, initial moisture content, irrigation, recirculation, liquid waste co-disposal, and refuse decomposition) and those that affect moisture distribution within the landfill (such as refuse age and pretreatment, compaction, permeability, particle size, density, settlement, vegetation, cover, sidewall and liner material, and gas and heat generation and transport) (El-Fadel et al., Citation1997, Citation1999, Citation2000, Citation2002, Citation2003). Similarly, the leachate quality is affected by several factors, such as the refuse soluble content, as well as by-products of chemical and biological processes within the landfill. Leachate quality can exhibit considerable spatial and temporal variations depending on site operations, refuse characteristics (composition and age), and internal landfill processes requiring varied management options. For this purpose, several options can be adopted, including discharge into sewer systems for subsequent treatment with municipal wastewater (Çeçen and Çakiroğlu, Citation2001; Trabelsi et al., 2011; Puszczało et al., Citation2010), recirculation (Rodríguez et al., 2004; Sethi et al., Citation2013), evaporation followed by sludge disposal (Afsharnia et al., Citation2012), and on-site treatment and disposal (Bodzek et al., Citation2006; Nurisepehr et al., Citation2012).
Historically, various physical, biological, and chemical processes have been applied for leachate management, depending on its quality, discharge requirements, and financial constraints (Renou et al., Citation2008). In this context, the sequencing batch reactor (SBR) technology is a development of the conventional activated-sludge process whereby the treatment steps are combined into a single basin or tank, often referred to as an activated sludge process occurring in time rather than in space (Singh and Srivastava, Citation2011). The technology offers a distinctive flexibility in terms of cycle time and sequence (Monclús et al., Citation2009). Thus the SBR technology can be desirable when considering landfill leachate associated with significant variations in quality and quantity.
More recently, combining membrane separation and biodegradation processes led to the development of the membrane bioreactor (MBR) technology, which is increasingly recognized as the process of choice for the treatment of wastewater streams containing complex and recalcitrant compounds (Bilad et al., Citation2011; Ahmed and Lan, Citation2012). An MBR can be considered as a conventional activated sludge system with efficient membrane filtration that holds small pore sizes of less than 0.1 μm (Santos and Judd, Citation2010). In the context of leachate management using the MBR technology, more efforts have targeted low-strength leachate (chemical oxygen demand [COD]: 1970–2430, 1391–3977, and 1200–1600 mg L–1; Laitinen et al., Citation2006; Tsilogeorgis et al., Citation2008; Zhang et al., 2013) with fewer attempts at moderate to high strength leachate (COD: 4113–9257, 10,435–31,318, and 16,360 mg L–1; Xiu-Fen et al., Citation2011; Bai et al., Citation2011; Campagna et al., Citation2013). In general, considerably high biochemical oxygen demand (BOD) removals (90–99%) were attained with the MBR technology irrespective of experimental conditions and leachate maturity. In contrast, the efficiency of the MBR in removing COD varied significantly from as low as 25% to as high as >98% (Puszczało et al., Citation2010, Aloui et al., Citation2009, Jia et al., Citation2009; Jakopović, et al., Citation2008; Chen and Liu, Citation2006). Further work has been reported regarding the suitability and viability of the MBR technology for the treatment of leachate mixed with municipal wastewater (Ahmed and Lan, Citation2012), although potential biological inhibition and plant overloading are of concern in this context (Çeçen and Aktaş, Citation2004). In this study, the SBR and MBR technologies were tested under different operating conditions to evaluate their feasibility for the management of high-strength leachate from a full-scale operating landfill.
Material and Methods
Leachate characterization and infeed preparation
Leachate was collected from a landfill located in the Bekaa valley (33.83° N, 35.91° E) at 70 km east of Beirut, Lebanon. The landfill site occupies ˜180,000 m2 at an altitude of ˜1000 m above mean sea level. The facility receives ˜200 tons per day of MSW with ˜70% as organic food waste. Samples of leachate were first analyzed for a wide range of indicators using standard methods for the examination of water and wastewater () (APHA Citation2005). The results defined the main indicators to be used in the evaluation of the performance of the experimental systems, whereby those present at significantly high levels (BOD5, COD, total nitrogen [TN], ammonia [NH3-N]) were selected as performance indicators. Following the initial screening, a larger volume of leachate (from the landfill and the evaporation pond) was collected and transported to the laboratory using 20-L containers, which were stored at 4ºC to feed the system at a later stage. Selected indicators (volatile suspended solids [VSS], BOD5, COD, TN, NH3-N) were monitored periodically to evaluate the systems’ performance.
Table 1. Screening of leachate quality
MBR setup
The experimental setup of the MBR system consisted of an anoxic tank followed by an aeration tank integrated with an ultrafiltration (UF) unit to clarify the effluent. The UF membrane ZW-10 was provided by ZENON and has a contact area of ˜10 ft2 (0.929 m2) divided in several hollow-fiber membranes with nominal pore size of 0.03 μm, a dry weight of ˜4.2 lb (1.9 kg), and a wet weight of ˜4.6 lb (2.1 kg). The membrane was kept in its original package soaked with glycerine until the experimental program was initiated.
The system components included a Plexiglas tank for housing the membrane with a capacity of ˜20 L preceded by two larger plastic process tanks with the first one utilized as an anoxic tank and the second as an aeration tank (). A pressure sensor was connected to a Sensotec digital display with an accuracy of ± 0.05% to record pressure variations in the membrane and to define the backwash frequency for periodic cleaning. The system was equipped with several pumps with variable speed and reverse operation modes to ensure continuous flow: a permeate and backpulse pump (Masterflex series 7554-20; Cole-Parmer, US) with a flow range of 0–60 L hr–1; a recirculation pump (Masterflex series 7524; Cole-Parmer, US) with a flow range of 0 to 140 L hr–1; and a feed pump (Masterflex series 7550; Cole-Parmer, US) with a flow range of 0-20 L hr–1. An aeration blower that can provide an airflow of up to 3000 L hr–1 was used to help in scrubbing the membrane, preventing potential fouling, and increasing the backpulsing rate. The aeration was supplied through five air diffusers distributed equidistantly in the aeration tank to provide uniform diffusion in the tank while the temperature was maintained at about 22 ± 1ºC in a temperature-controlled room.
Figure 1. Experimental setup of the MBR system. (a) Infeed/raw leachate; (b) Mixer; (c) Anoxic tank; (d) Aeration tank; (e) Air diffusers; (f) Air blower; (g) Pressure sensor; (h) Permeate/Backpulse pump; (i) Hollow fiber MBR; (j) Recirculation, 5-10Q; (k) Return sludge; (l) Drain; (m) Permeate tank, (s) Sampling location.
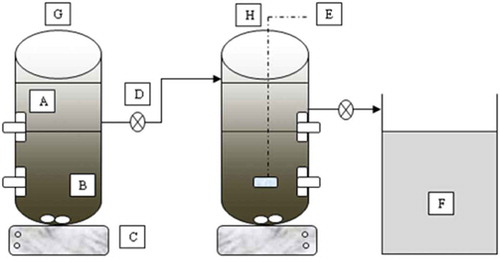
The tanks were first filled with the influent leachate while opening the aeration valves in the aerobic tank, turning on the mixer in the anoxic tank at a low speed (˜100 rpm), and recirculating the flow between all tanks without feed or permeation. This was recorded as phase 1 of the experimental program and was intended for the acclimation of bacteria. The bacterial activity was observed through the temporal increase of mixed liquor volatile suspended solids (MLVSS) in the aerobic tank. The second phase required increasing the influent flow rate. During each phase, the system was operated without sludge wastage while changing several parameters to maintain a reasonable variation in the transmembrane pressure and a constant flux. summarizes the experimental program with the duration and range of parameters applied. Parameter variation was based primarily on the observed flow rate. For instance, when the flow increased in the permeation, the backpulse frequency and scour airflow were increased to maintain a constant flow and clean the membrane. Membrane cleaning was conducted periodically to help reduce fouling and maintain a constant membrane flux. This was achieved by reversing the flow through the membrane using the temporarily stored permeate. Based on the recommendation of the manufacturer (ZENON), periodic chemical cleaning was performed on a need basis using sodium hypochlorite diluted with water at a concentration of 250 mg L–1 for a minimum of 3 backpulses.
Table 2. MBR experimental program
SBR setup
The bench-scale SBR experimental setup () comprised two cylindrical tanks of 17.4 cm diameter, 40 cm depth, and a total volume of 9.5 L each. The first tank served as the leachate feeding container, while the second tank served as the SBR. An air diffuser was installed at the base of the cylinder to maintain the dissolved oxygen (DO) level between 6 and 8 mg L–1 during the aerated phases.
Figure 2. Experimental setup of the SBR system. (a) Fill volume; (b) Settled sludge; (c) Magnetic mixers; (d) Peristaltic pump; (e) Air inflow; (f) Effluent tank; (g) Feeding tank; (h) SBR.
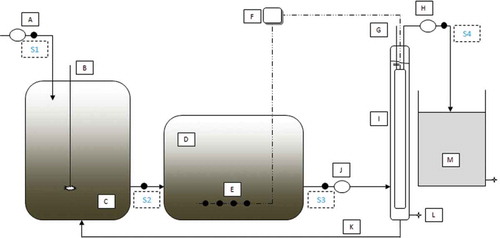
Bacterial inoculum was obtained from the aeration tank of the MBR setup for biomass acclimation, which was initiated by allowing 5.2 L of the seed to settle in the reactor for 1 hr, and taking 2.6 L of the supernatant and replacing it with an equal amount of raw leachate. The reactor was then operated under continuous mixing and aeration for 21 days, during which settling, decantation, and replacement with leachate were performed every 2 or 3 days. Several scenarios were tested (), in a temperature-controlled room at 22 ± 1ºC, with an effective volume of 5.2 L, and an influent/effluent volume of 2.6 L. The first three experiments served as starting trials to evaluate the performance of the SBR at low HRT under variable aeration patterns of the reacting phase, whereas the last two experiments targeted the assessment of the effect of longer HRTs with different aeration patterns as well as the effect of pH adjustment.
Table 3. SBR experimental program
Results and Discussion
Leachate characterization
compares the leachate characteristics with national standards for discharge in water bodies (Ministry of Environment [MoE], 2001) as well as with the EPA recommended limits for reclaimed water for irrigation (short term use), and the FAO Recommended maximum concentrations of trace elements in irrigation waters. The limits were exceeded for most parameters except metals, which exhibited low levels, indicating that metal treatment was deemed unnecessary prior to the bioreactor. Low metal concentrations can be attributed to the type of waste received at the landfill, that is, municipal waste with a high fraction of food waste (up to 70%) (Tatsi and Zouboulis, Citation2002).
Ammonia levels in the landfill leachate were high, ranging from 1800 to 4700 mg L–1, which could be attributed to the high percentage of food waste deposited in the landfill, yet these levels were comparable to and at times lower than reported concentrations in nearby Mediterranean regions (Clement, Citation1995; Loizidou et al., Citation1992; Tsonis, Citation1998; Tatsi and Zouboulis, Citation2002). Similarly, high levels of COD (9520–17,760 mg L–1), BOD5 (4890–10,935 mg L–1), and TN (2200–5250 mg L–1) were recorded, and hence these parameters were selected as the main indicators in the performance assessment of the experimental systems.
SBR experiments
The SBR removal efficiency reached 84.6% for BOD, 46.7% for COD, 71.4% for NH3, and 72.5% for TN, resulting in considerably low levels of contaminants in the final effluent (). Nevertheless, the discharge standards of the World Health Organization (WHO) were not attained, necessitating additional treatment for the residual COD and nitrogen concentrations in particular. The effectiveness of the SBR results becomes more pronounced when put in the context of the literature (). For instance, the solid retention time (SRT) in this study varied between 12.9 and 14.1 days, which is on the lower range of literature-reported values of 8 to 80 days (Klimiuc and Kulikowska, Citation2006; Kulikowska et al., Citation2007), although the quality of the leachate used is at times stronger by severalfold than the leachate used in the literature. Similarly, the corresponding hydraulic retention time (HRT) ranged between 0.66 and 6 days, which is also on the lower range of literature-reported values of 0.19 to 20 days (Neczaj et al., Citation2005; Klimiuc and Kulikowska, Citation2006; Kulikowska et al., Citation2007). While the leachate strength (COD: 9095 mg L–1; BOD: 3114 mg L–1; NH3: 1400 mg L–1) was on the higher range of those reported in the literature, similar, and at times better, attenuation was achieved, albeit with the relatively lower HRT and SRT. Zouboulis et al. (Citation2001) improved the SBR performance by adding enzymes, whereby removal rates of COD, BOD, and NH3 increased to 40, 70, and 60%, which were still lower than the efficiencies obtained in this study (47, 82, and 71.4%, respectively). Although they used a notably lower strength leachate (COD: 1396 vs. 9095 mg L–1, and NH3: 579 vs. 1400 mg L–1), Aziz et al. (Citation2011) managed to raise the COD removal from 27 to 50% with the application of the double react–settle scenario. Finally, slightly higher COD removal (≪56.9 vs. 47%) and lower BOD removal (<79.6 vs. 82%) were reported by Guo et al. (Citation2010) while treating young landfill leachate mixed with wastewater corresponding to a significantly lower strength leachate in terms of COD (1625 vs. 9095 mg L–1 in this study) and BOD (619 vs. 3114 mg L–1). When the total equivalent substrate removed is used as an indicator, the efficiency of the treatment adopted in this study becomes more pronounced whereby the load of COD, BOD5, and NH3 removed are higher than those reported in the literature. For instance, the total equivalent COD, BOD5, and ammonia removed were 4365.6, 2553.5, and 999.6 mg/L, respectively, while as low as 211.7, 442, and 5 mg/L were reported in previous studies for those parameters ().
Table 4. SBR removal efficiencies in comparison with literature reported values
MBR experiments
In the MBR experiments, greater than 95% removal efficiencies were attained for all indicators (VSS, BOD5, TN, NH3-N), while for COD the removal efficiency reached 70% (). The relatively high effluent concentrations of COD can be attributed to the presence of non-biodegradable compounds in the leachate. Operation with no sludge wasting is another factor that can contribute to low COD removal, whereby only 50% COD removal was achieved when treating old landfill leachate using an MBR at infinite SRT (Tsilogeorgis et al., Citation2008). Overall average removal efficiencies for various indicators (VSS: 95%; BOD5: 99.5%; COD: 70%; TN: 95%; NH3-N: 96%) were higher or within the range reported in the literature () (BOD5: 85–100%; COD: 38–84%; TN: 43–88%; NH3-N: 31–75%), although the initial concentrations of leachate used in this study (BOD5: 4000–6000 mg L–1; COD: 9000–11,000 mg L–1; TN: 2000–6000 mg L–1; NH3-N: 1800–4000 mg L–1) were generally higher than literature-reported values (BOD5: 100–1300 mg L–1; COD: 235–2456 mg L–1; TN: 240–1141 mg L–1; NH3-N: 780–2180 mg L–1) with the exception of Visvanathan et al. (Citation2007), who used relatively young leachate (BOD/COD = 0.39–0.65) at similar or stronger characteristics for BOD5 (4714–9498 mg L–1) and COD (12,000 m L–1) but lower for NH3-N (1000–1700 mg L–1). Visvanathan et al. (Citation2007) operated at a temperature of 45ºC, which is twofold the temperature used in this study (22ºC), with removal efficiencies similar for BOD5 (99 vs. 99.5%), lower for NH3-N (75 vs. 96%) but higher for COD (79 vs. 70%).
Figure 4. Variation of parameters tested throughout the MBR experimental program. S1: Raw leachate, S2: Effluent from anoxic tank, S3: Effluent from aeration tank, and S4: Effluent from MBR.
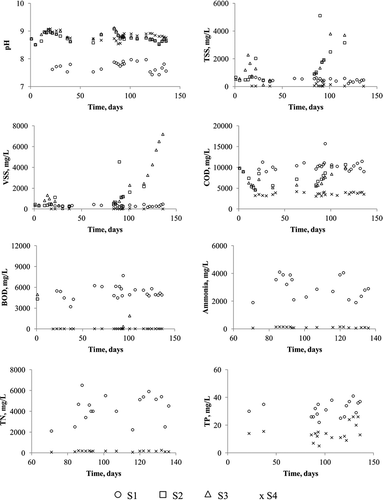
Table 5. MBR removal efficiencies in comparison with literature reported values
MBR vs. SBR
While the SBR technology offers some flexibility in terms of cycle time and sequence, its performance is constrained when considering landfill leachate associated with significant variations in quality and quantity. Combining membrane separation and biodegradation processes or the MBR technology improved removal efficiencies significantly. When comparing the SBR and MBR results in this study, the MBR exhibited significantly better performance in removing BOD, COD, NH3, and TN, with corresponding improvement in removal efficiencies of 21, 33, 34, and 30%, respectively (). This could be attributed to the ability of the MBR to operate at higher SRT and MLVSS levels than those of the SBR. Such high levels of biomass in the MBR allow slowly biodegradable substrates to be consumed in the reactor, which could be explained by eq 1 (Bérubé, Citation2010):
Table 6. Summary of SBR and MBR overall comparative performance
where rS is the rate at which the substrate is consumed (M/L3-T), k is the maximum rate of substrate consumption per unit mass of biomass (1/T), X the concentration of biomass in the bioreactor (M/L3), and kS the half saturation constant for the substrate being consumed by the biomass (M/L3).
Easily biodegradable compounds have a high maximum rate of substrate consumption (k) and hence can be rapidly consumed. By maintaining a high MLVSS level in the reactor, slowly biodegradable compounds can be equally consumed at a fast rate ( eq 1). In MBR systems, the membrane module preserves much of the biomass within the bioreactor, thus allowing for relatively high biomass concentrations. In contrast, high SRTs, accompanied by high MLVSS levels, cannot be maintained in SBR systems because the latter rely on gravity settling for the separation of biomass, which is less effective than the membrane especially at high MLVSS since the sludge loses its ability to settle into a separate layer. The improved performance of the MBR can be attributed to the membrane separation of the mixed liquor from the treated water whereby the fine pores increase the removal of organics relative to gravity settling in the SBR system.
The presence of the membrane for biomass separation is the main difference between SBR and MBR systems. It provides several benefits, including reduced footprint, higher effluent quality, less sludge production, and ease of retrofitting. When operating at full scale, the elimination of phase separation using gravity settling reduces the burden of continuous monitoring of the sludge quality, which otherwise may vary daily, necessitating more process oversight and on-site adjustment in the SBR operation to maintain good settling characteristics for sustaining high effluent quality. Furthermore, for the same quality effluent, the SBR system will inevitably require a tertiary process when treating high-strength wastewater such as leachate, thus increasing the overall capital investment as well as operation and maintenance costs. When constraints associated with footprint and high-quality effluent are relaxed, the SBR may become economically more effective, which is often the case for low-strength wastewater in rural communities.
Summary
The performance of the SBR and MBR technologies for the management of high-strength landfill leachate was demonstrated at laboratory scale using leachate with COD (9000–11,000 mg L–1), BOD5 (4000–6000 mg L–1), TN (2000–6000 mg L–1), and NH3-N (1800–4000 mg L–1) as indicators. While the MBR exhibited higher average removal rates than the SBR (BOD5: 99.5 vs. 82%; COD: 70 vs. 47%; TN: 95 vs. 73%; and NH3-N: 96 vs. 71.4%), post-reatment is invariably required for high-strength leachate, depending on influent levels, discharge standards, or effluent end use. For COD in particular, discharge standards are seldom attained, indicating that depending on the intended use of the leachate, the design of a full-scale treatment facility must account for the non-biodegradable fraction by introducing an additional treatment process to lower the effluent residual COD and attain discharge standards. The comparative assessment assists in technology selection depending on the desired effluent quality in terms of allowable pollution load. The footprint, technical expertise, and membrane lifetime constitute important decision factors to consider when assessing the economic burden of adopting the MBR technology for high strength leachate.
Acknowledgment
Special thanks are extended to the U.S. Agency for International Development for its support of the rehabilitation efforts at the Zahle landfill facility.
Additional information
Notes on contributors
M. El-Fadel
M. El-Fadel is a professor and chairperson, Department of Civil and Environmental Engineering, Faculty of Engineering and Architecture, American University of Beirut. He holds the Dar Al-Handasah (Shair & Partners) Chair in Engineering.
J. Hashisho
J. Hashisho is a graduate student at the Department of Civil and Environmental Engineering, Faculty of Engineering and Architecture, American University of Beirut.
References
- Ahmed, F.N., and C.Q. Lan. 2012. Treatment of landfill leachate using membrane bioreactors: A review. Desalination 287:41–54. doi:10.1016/j.desal.2011.12.012
- Afsharnia, M., A. Torabian, G.R. Mousavi, and M.A. Abduli. 2012. Landfill leachate treatment by sono-evaporation. Desalin. Water Treat. 48(1–3): 344–48. doi:10.1080/19443994.2012.702959
- Ahn, W.Y., M.S. Kang, S.K. Yim, and K.H. Choi. 2002. Advanced landfill leachate treatment using an integrated membrane process. Desalination 149(1–3): 109–14. doi:10.1016/S0011-9164(02)00740-3
- Aloui, F., F. Fki, S. Loukil, and S. Sayadi. 2009. Application of combined membrane biological reactor and electro-oxidation processes for the treatment of landfill leachates. Water Sci. Technol. 60(3): 605–14. doi:10.2166/wst.2009.377
- American Public Health Association (APHA)/American Water Works Association (AWWA)/Water Environment Federation (WEF). 2005. Standard methods for the examination of water and wastewater, 21st ed. Washington, DC: APHA/AWWA/WEF.
- Aziz, S.Q., H.A. Aziz, and M.S. Yusoff. 2011. Powdered activated carbon augmented double react-settle sequencing batch reactor process for treatment of landfill leachate. Desalination 277(1–3):313–20. doi:10.1016/j.desal.2011.04.046
- Bai, Y., H. Wang, J. Cui, G. Li, B. Han, and J. Pi. 2011. A novel anoxic-oxic hybrid membrane bioreator for landfill leachate treatment. Third International Conference on Measuring Technology and Mechatronics Automation, ICMTMA 2011, 1, 183–186. Shanghai, China, January.
- Bérubé, P. 2010. Membrane bioreactors: Theory and applications to wastewater reuse. Sustain. Sci. Eng. 2(C): 255–92. doi:10.1016/S1871-2711(09)00209-8
- Bilad, M.R., P. Declerck, A. Piasecka, L. Vanysacker, X. Yan, and I.F.J. Vankelecom. 2011. Treatment of molasses wastewater in a membrane bioreactor: Influence of membrane pore size. Sep. Purif. Technol. 78(2): 105–12.
- Bodzek, M., E. Łobos-Moysa, and M. Zamorowska. 2006. Removal of organic compounds from municipal landfill leachate in a membrane bioreactor. Desalination 198:16–23. doi:10.1016/j.desal.2006.09.004
- Campagna, M., M. Çakmakci, F. Büşra Yaman, and B. Özkaya. 2013. Molecular weight distribution of a full-scale landfill leachate treatment by membrane bioreactor and nanofiltration membrane. Waste Manage. 33(4): 866–870 doi:10.1016/j.wasman.2012.12.010
- Çeçen, F., and Ö. Aktaş. 2004. Aerobic co-treatment of landfill leachate with domestic wastewater. Environ. Eng. Sci. 21(3): 303–12. doi:10.1089/109287504323066941
- Çeçen, F., and D. Çakiroğlu. 2001. Impact of landfill leachate on the co-treatment of domestic wastewater. Biotechnol. Lett. 23(10): 821–26. doi:10.1023/A:1010317823529
- Chen, S., and J. Liu. 2006. Landfill leachate treatment by MBR: Performance and molecular weight distribution of organic contaminant. Chin. Sci. Bull. 51(23): 2831–38. doi:10.1007/s11434-006-2177-y
- Clement, B. 1995. Physico-chemical characterization of 25 French landfill leachates. Proceedings of Sardinia 95, 5th International Landfill Symposium, CISA, Cagliari. Italy, October, 315–25.
- El-Fadel, M., E. Bou-Zeid, and W. Chahine. 2003. Landfill evolution and treatability assessment of high-strength leachate from MSW with high organic and moisture content. Environ. Stud. Part A 60(6): 603–15. doi:10.1080/0020723032000069187
- El-Fadel, M., E. Bou-Zeid, W. Chahine, and B. Alayli. 2002. Temporal variation of leachate quality from pre-sorted and baled MSW with high organic and moisture content. Waste Manage. 22(3): 269–82. doi:10.1016/S0956-053X(01)00040-X
- El-Fadel, M., and R. Khoury. 2000. Modeling settlement of organic refuse: A critical assessment. Crit. Rev. Environ. Sci. Technol. 30(3): 327–61. doi:10.1080/10643380091184200
- El-Fadel, M. 1999. Leachate recirculation effects on settlement and biodegradation rates in MSW landfills. Environ. Technol. 20(2): 121–34. doi:10.1080/09593332008616802
- El-Fadel, M., A. Findikakis, and J. Leckie. 1997. Modeling leachate generation and transport in solid waste landfills. Environ. Technol. 18(7): 669–86. doi:10.1080/09593331808616586
- Feki, F., F. Aloui, M. Feki, and S. Sayadi. 2009. Electrochemical oxidation post-treatment of landfill leachates treated with membrane bioreactor. Chemosphere 75(2): 256–60. doi:10.1016/j.chemosphere.2008.12.013
- Guo, J.S., A.A. Abbas, Y.P. Chen, Z.P. Liu, F. Fang, and P. Chen. 2010. Treatment of landfill leachate using a combined stripping, fenton, SBR, and coagulation process. J. Hazard. Mater. 178(1–3): 699–705. doi:10.1016/j.jhazmat.2010.01.144
- Ince, M., E. Senturk, G. Onkal Engin, and B. Keskinler. 2010. Further treatment of landfill leachate by nanofiltration and microfiltration–PAC hybrid process. Desalination 255(1–3): 52–60. doi:10.1016/j.desal.2010.01.017
- Jakopović, H.K., M. Matošić, M. Muftić, M. Čurlin, and I. Mijatović. 2008. Treatment of landfill leachate by ozonation, ultrafiltration, nanofiltration and membrane bioreactor. Fres. Environ. Bull. 17(6): 687–95. doi:10.2166/wst.2008.700
- Jia, H., Z. Linsheng, and L. Yuezhong. 2009. Application of UASB-MBR system for landfill leachate treatment. International Conference on Energy and Environment Technology, ICEET 2009. Guilin, Guangxi, October.
- Klimiuc, E., and D. Kulikowska. 2006. Organics removal from landfill leachate and activated sludge production in SBR reactors. Waste Manage. 26: 1140–47. doi:10.1016/j.wasman.2005.09.011
- Kulikowska, D., E. Klimiuk, and A. Drzewicki. 2007. BOD5 and COD removal and sludge production in SBR working with or without anoxic phase. Bioresource Technol. 98(7): 1426–32. doi:10.1016/j.biortech.2006.05.021
- Laitinen, N., A. Luonsi, and J. Vilen. 2006. Landfill leachate treatment with sequencing batch reactor and membrane bioreactor. Desalination 191(1–3): 86–91. doi:10.1016/j.desal.2005.08.012
- Lin, S., and C. Chang. 2000. Treatment of landfill leachate by combined electro-Fenton oxidation and sequencing batch reactor method. Water Res. 34: 4243–49. doi:10.1016/S0043-1354(00)00185-8
- Loizidou, M., E.G. Kapetanios, and A. Papadopoulos. 1992. Assessment of leachate characteristics and its treatability. Fres. Environ. Bull. 1:748–53. doi:10.1080/10934529209375780
- Ministry of Environment. 2001. Standards for discharge into surface waters. Decision 8/1-January, Ministry of Environment, Beirut, Lebanon.
- Monclús, H., S. Puig, M. Coma, A. Bosch, M.D. Balaguer, and J. Colprim. 2009. Nitrogen removal from landfill leachate using the SBR technology. Environ. Technol. 30(3): 283–90. doi:10.1080/09593330802622105
- Neczaj, E., E. Okoniewska, and M. Kacprzak. 2005. Treatment of landfill leachate by sequencing batch reactor. Desalination 185(1–3): 357–62. doi:10.1016/j.desal.2005.04.044
- Nurisepehr, M., S. Jorfi, R.R. Kalantary, H. Akbari, R.D.C. Soltani, and M. Samaei. 2012. Sequencing treatment of landfill leachate using ammonia stripping, fenton oxidation and biological treatment. Waste Manage. Res. 30(9): 883–87. doi:10.1177/0734242X11433526
- Puszczało, E., J. Bohdziewicz, and A. Świerczyńska. 2010. The influence of percentage share of municipal landfil leachates in a mixture with synthetic wastewater on the effectiveness of a treatment process with use of membrane bioreactor. Desalin. Water Treat. 14(1–3): 16–20. doi:10.5004/dwt.2010.1066
- Renou, S., J.G. Givaudan, S. Poulain, F. Dirassouyan, and P. Moulin. 2008. Landfill leachate treatment: Review and opportunity. J. Hazard. Mater. 150:468–93. doi:10.1016/j.jhazmat.2007.09.077
- Rodríguez, D.C., O. Ramírez, and G.P. Mesa. 2011. Behavior of nitrifying and denitrifying bacteria in a sequencing batch reactor for the removal of ammoniacal nitrogen and organic matter. Desalination 273(2–3): 447–52. doi:10.1016/j.desal.2011.01.068
- Santos, A., W. Ma, and S.J. Judd. 2010. Membrane bioreactors: Two decades of research and implementation. Desalination 273(1): 148–54. doi:10.1016/j.desal.2010.07.063
- Sethi, S., N.C. Kothiyal, and A.K. Nema. 2013. Stabilisation of municipal solid waste in bioreactor landfills—An overview. Int. J. Environ. Pollut. 51(1–2): 57–78. doi:10.1504/IJEP.2013.053175
- Singh, M., and R.K. Srivastava. 2011. Sequencing batch reactor technology for biological wastewater treatment: A review. Asia-Pacific J. Chem. Eng. 6(1): 3–13. doi:10.1002/apj.490
- Tatsi, A.A., and A.I. Zouboulis. 2002. A field investigation of the quantity and quality of leachate from a municipal solid waste landfill in a Mediterranean climate (Thessaloniki, Greece). Adv. Environ. Res. 6:207–19. doi:10.1016/S1093-0191(01)00052-1
- Trabelsi, I., S. Salah, and F. Ounaeis. 2013. Coupling short-time sequencing batch reactor and coagulation-settling process for co-treatment of landfill leachate with raw municipal wastewater. Arab. J. Geosci. 6(6): 2071–9.
- Trebouet, D., J.P. Schlumpf, P. Jaouen, and F. Quemeneur. 2001. Stabilized landfill leachate treatment by combined physicochemical-nanofiltration processes. Water Res. 35(12): 2935–42. doi:10.1016/S0043-1354(01)00005-7
- Tsilogeorgis, J., A. Zouboulis, P. Samaras, and D. Zamboulis. 2008 Application of a membrane sequencing batch reactor for landfill leachate treatment. Desalination 221:483–93. doi:10.1016/j.desal.2007.01.109
- Tsonis, S. 1998. Characteristics and treatability of the leachate from the municipal landfill of the city of Patras. Proceedings of Protection and Restoration of the Environment IV, Halkidiki, Greece, July, 667–74.
- Uygur, A., and F. Kargi. 2004. Biological nutrient removal from pre-treated landfill leachate in a sequencing batch reactor. J. Environ. Manage. 71: 9–14. doi:10.1016/j.jenvman.2004.01.002
- Visvanathan, C., M.K. Choudhary, M.T. Montalbo, and V. Jegatheesan. 2007. Landfill leachate treatment using thermophilic membrane bioreactor. Desalination 204(1–3 spec. Iss.): 8–16. doi:10.1016/j.desal.2006.02.028
- Xiu-Fen, L., D. Barnes, and C. Jian. 2011. Performance of struvite precipitation during pretreatment of raw landfill leachate and its biological validation. Environ. Chem. Lett. 9(1): 71–75. doi:10.1007/s10311-009-0248-4
- Zhang, J., S.I. Padmasiri, M. Fitch, B. Norddahl, L. Raskin, and E. Morgenroth. 2007. Influence of cleaning frequency and membrane history on fouling in an anaerobic membrane bioreactor. Desalination 207(1–3): 153–66. doi:10.1016/j.desal.2006.07.009
- Zouboulis, A., M. Loukidou, and K. Christodoulo. 2001. Enzymatic treatment of sanitary landfilled leachate. Chemosphere 44(2001): 1103–8. doi:10.1016/S0045-6535(00)00343-X