Abstract
High-temperature particle control (HTPC) using a ceramic filter is a dust collection method without inefficient cooling and reheating of flue gas treatment; thus, its use is expected to improve the energy recovery efficiency of municipal solid waste incinerators (MSWIs). However, there are concerns regarding de novo synthesis and a decrease in the adsorptive removal efficiency of dioxins (DXNs) at approximately 300°C. In this study, the effect of natural and activated acid clays on the decomposition of monochlorobenzene (MCB), one of the organochlorine compounds in MSW flue gas, was investigated. From the results of MCB removal tests at 30–300°C, the clays were classified as adsorption, decomposition, and low removal types. More than half of the clays (four kinds of natural acid clays and two kinds of activated acid clays) were of the decomposition type. In addition, the presence of Cl atoms detached from MCB was confirmed by washing the clay used in the MCB removal test at 300°C. Activated acid clay was expected to have high dechlorination performance because of its proton-rich-composition, but only two clays were classed as decomposition type. Conversely, all the natural acid clays used in this work were of the decomposition type, which contained relatively higher di- and trivalent metal oxides such as Al2O3, Fe2O3, MgO, and CaO. These metal oxides might contribute to the catalytic dechlorination of MCB at 300°C. Therefore, natural and activated acid clays can be used as alternatives for activated carbon at 300°C to remove organochloride compounds such as DXNs. Their utilization is expected to mitigate the latent risks related to the adoption of HTPC, and also to contribute to the improvement of energy recovery efficiency of MSWI.
Implications
The effect of natural and activated acid clays on MCB decomposition was investigated to evaluate their suitability as materials for the removal of organochlorine compounds, such as DXNs, from MSWI flue gas at approximately 300°C. More than half of the clays used in this study showed the decomposition characteristics of MCB. The presence of Cl atoms in the clay used in the MCB removal test at 300°C proved the occurrence of MCB decomposition. The results of this study suggest a novel flue gas treatment method to establish high-energy efficient MSWI systems.
Introduction
With increasing concerns regarding global warming, waste-to-energy processing is being promoted to secure alternative energy sources. In this respect, improvement of energy recovery efficiency is a significant concern in the operation of municipal solid waste incinerators, (MSWIs). There are two approaches to enhancing the energy recovery efficiency of MSWIs. The first is to increase the amount of recovered heat or electricity, and the second is to increase the net efficiency by modifying or changing the processes that cause inefficient energy consumption in these operations. shows a typical MSWI flue gas treatment system, which is composed of a quenching chamber, dry scrubber, fabric filter, and selective catalytic reduction (SCR) reactor. The fabric filter is a common dust collection device but the feed flue gas should be cooled to approximately 150°C because the filter material has low thermal resistance. This lower temperature is also advantageous in minimizing the de novo synthesis of dioxins (DXNs) and in increasing the removal efficiency of DXNs using an activated carbon spray. However, the exit gas from the fabric filter is often reheated to approximately 210°C to activate the catalyst in the SCR reactor for NOx removal. This cooling and reheating is one of the inefficient operations in flue gas treatment.
As shown in , high-temperature particle control (HTPC) using a ceramic filter is a potential alternative and may eliminate the need for this inefficient cooling and reheating (Saxena et al., Citation1982; Reijnen and Van Brakel, Citation1984; Alvin, Citation1996; Heidenreich, Citation2013). However, HTPC at approximately 300°C might lead to the de novo synthesis of DXNs along with a decrease in the adsorptive removal efficiency of DXNs. In addition, extra treatments, such as thermal decomposition under a nitrogen atmosphere (Hagenmaier treatment) or melting, may be required to reduce the increased DXNs concentration in fly ash (Weber et al., Citation2002a; Weber et al., Citation2002b). Finally, there might be a risk of spontaneous ignition of activated carbon used for DXNs adsorption, as a result of its heat accumulation. As a countermeasure, using catalysts, such as V2O5-WO3/TiO2, is possible to decompose DXNs at 200–400°C, but catalysts based on transition metal oxides are expensive (Kanda et al., Citation1998; Stoll et al., Citation2001; Sugishima and Kobayashi, Citation2001; Yang et al., Citation2008). Therefore, it is necessary to develop a cheap and thermally stable material for DXNs removal at 300°C and, if possible, to perform this removal by decomposition rather than adsorption.
Natural acid clay is a clay mineral compounded with montmorillonite (Al2O3·4SiO2·nH2O). It has a porous structure and is acidic because its anions are substituted by protons during eluviations from the clay structure by weathering in the natural environment (Hirokawa, Citation1980; Komadel, Citation2003; Hussin et al., Citation2011). In addition, activated acid clay can be produced by hot acid treatment of natural acid clay using sulfuric acid to enhance its physicochemical properties (Hirokawa, Citation1980; Komadel, Citation2003; Pushpaletha et al., Citation2005; Ugochukwu et al., Citation2013). Both natural and activated acid clays are commonly used for bleaching of fat and oils, purification of petroleum, and as absorbents. From their catalytic property based on Si/Al structure, metal oxides, protons, and large specific surface area, the potential use of these clays as DXNs removal material is promising.
Furubayashi et al. (Citation2002) investigated the adsorptive removal of 1,2,3,4-tetrachlorinated dibenzo-p-dioxin (T4CDD) at 150°C using a lab-scale reactor and various inorganic adsorbents such as perlite, diatomaceous silica, slaked lime, zeolite, sepiolite, bentonite, natural acid clay, and activated acid clay. They reported that activated clay showed the highest T4CDD removal efficiency of these inorganic adsorbents. Ando et al. (Citation2009) tested a dry spray of activated acid clay to remove DXNs in the flue gas generated from an MSWI facility that had a capacity of 350 tons/d. They noted that the DXNs concentration was less than 0.1 ng-TEQ/N-m3 at the exit of the fabric filter. Gao et al. (Citation2010) investigated the vapor-phase sorption of hexachlorobenzene (HCB) on montmorillonite at 250°C and observed that the sorption capacity of HCB was equal to that of activated carbon at 330°C.
Despite several studies on the adsorptive removal of DXNs or organochlorine compounds using natural and activated acid clays, no research has focused on their effect on the decomposition of these compounds. In this study, 10 types of natural and activated acid clays were examined to determine their decomposition effect on mono-chlorobenzene (MCB). First, a physicochemical analysis of natural and activated acid clays was performed to determine the MCB removal mechanism. MCB was chosen as the organochlorine compound because it has only one C–Cl bond affected by dechlorination reaction. This makes it easy to determine whether the MCB is decomposed by the dechlorination reaction. Ninomiya et al. (Citation2000) used MCB to evaluate the performance of a DXNs catalyst and noted that bonding distances and the electronic states of C–Cl bonding of MCB and DXNs were similar to each other, according to quantum chemical calculations. MCB removal rates by natural and activated acid clays were determined at three different temperatures. The dechlorination of MCB was investigated by washing the clay used in the MCB removal test.
Materials and Methods
Four types of natural acid clay (AC1–AC4) and six types of activated acid clay (AC5–AC10) produced in Japan were used in this study. The pH, elemental composition, and surface characteristics of the clays were investigated to determine the relationship between their physicochemical properties and the MCB removal characteristics. The pH of the clay was determined using the soil analysis method proposed by the Japanese Ministry of Agriculture, Forestry and Fisheries (Citation2008): 20 g of clay was immersed in a flask containing 100 mL of distilled water, which was capped and shaken for 30 min at 200 rpm. After settling the mixture for 30 min, the pH of the supernatant was measured. The elemental composition (SiO2, Al2O3, Fe2O3, MgO, CaO, Na2O, K2O, and TiO2) was obtained by sequential x-ray fluorescence analysis (MagiX PRO, PANalytical Co.). The BET surface area and the pore volume were determined by surface area and pore size analysis (Autosorb 6, Quantachrome Co.).
shows the apparatus used for the MCB removal tests. Standard MCB gas was generated using a Permeater (PD-1B, GASTEC Co.). The airflow rate was set to 500 mL/min to vaporize MCB at a constant concentration of 38.5 ± 7.9 μg/L. A 1.5-g sample of clay was added to a stainless reactor (ø 13 mm × 72 mm). The lower and upper spaces of the reactor were filled with a glass fiber filter (GA-100, ADVANTEC Co.) and glass beads to support the clay layer. The temperature of the reactor was low (30–60°C), medium (120–150°C), or high (300°C). The MCB gas was injected into the reactor and retained there for 40 min. An experimental time of 40 min was used to accord with the residence time of dust on the fabric filter from deposition to dislodgement by a pulse jet in a real flue gas treatment system.
To determine the inlet and outlet MCB gas concentrations, MCB gas was absorbed by two absorption bottles containing 50 mL n-hexane every 10 min for 40 min. The MCB concentration in hexane was measured by gas chromatography–mass spectrometry (G-5000M, Hitachi Co.) using the internal standard method. The MCB removal rate (ŋ) was calculated using eq 1:
To determine whether the dechlorination of MCB occurred at a given temperature, the presence of Cl atoms detached from MCB was investigated in the absorption bottle containing 50 mL distilled water and in the clay used for MCB removal in the reactor. Compared to the previous MCB removal test, this experiment was performed with an MCB concentration more than 20 times higher (0.86 ± 0.17 mg/L) and with an experimental time that was 3 times longer (120 min) using 5 g clay. Taking the detection limit of chloride ion in aqueous solutions into account, the experiment with higher MCB concentration is advantageous to obtain conclusive evidence of the dechlorination of MCB. Clay was added to the reactor (ø 19 mm × 123 mm) and MCB was injected into the reactor at 150°C or 300°C. A chlorine gas absorption bottle containing 50 mL distilled water and MCB absorption bottles containing 50 mL n-hexane were placed at the exit from the reactor in that order. After 120 min of MCB removal test, the reactor was cooled to room temperature and all the clay was collected for a washing test. Washing was performed using 50 mL distilled water (liquid to solid ratio = 10) agitation at 200 rpm for 6 hr. The mixture was filtered using a 0.45-μm pore size membrane filter and the filtrate was adjusted to 50 mL. As a control test, virgin clay was washed and filtered in the same manner. The concentration of chloride ions in the filtrate was measured by anion chromatography (AS-8020, TOSOH Co.).
Results and Discussion
Physicochemical properties of natural and activated acid clays
shows the physicochemical properties of the four natural acid clays (AC1–AC4) and six activated acid clays (AC5–AC10) used in this study. The pH values of the activated acid clays ranged from 2.75 to 4.53, which are lower than those of the natural clays. These low pH values indicate the high proton capacity of the activated acid clay. There was no significant difference in metal composition between the natural and activated acid clays. However, the natural acid clays contained a relatively higher amount of di- and trivalent metal oxides such as Al2O3, Fe2O3, MgO, and CaO. Hot acid treatment led to the removal of Al, Fe, Mg, and Ca from the activated acid clays, whereas their specific surface areas and pore volumes increased to 100–261 m2/g and 0.25–0.45 cm3/g, respectively, except for AC10.
Table 1. Physicochemical properties of natural and activated acid clays
Blank MCB removal test without clay at each evaluated temperature
Prior to the MCB removal tests in the presence of clay, MCB gas was injected into the reactor, filled with only glass beads and a glass fiber filter, to confirm whether its adsorption or thermal decomposition occurred at 120°C and 300°C. As the results show, when the inlet MCB gas concentration was 33.8 ± 0.6 μg/L, the outlet MCB gas concentrations were 33.4 μg/L at 120°C and 32.7 μg/L at 300°C. The inlet and the outlet MCB concentrations were similar. Therefore, adsorption or the thermal decomposition of MCB did not occur between 120 and 300°C.
MCB removal by natural and activated acid clay at each temperature
and show the MCB removal rates by natural and activated acid clays every 10 min at low (30–60°C), medium (120–150°C), and high (300°C) temperatures. The MCB removal rates were highest for the first 10 min and then decreased with time. Except for AC10, all the clays showed high MCB removal rates at low temperature, which was considered to be the adsorptive removal of MCB. Differences in the MCB removal rates between the clays were observed in the experiments at medium and high temperatures.
Figure 3. MCB removal rates of natural acid clays for each temperature: (a) AC1, (b) AC2, (c) AC3, (d) AC4.
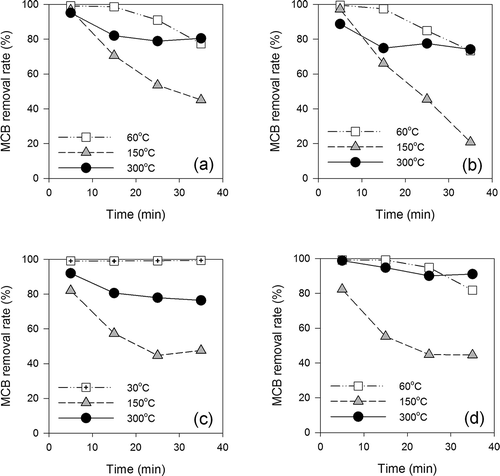
Figure 4. MCB removal rates of activated acid clays for each temperature: (a) AC5, (b) AC6, (c) AC7, (d) AC8, (e) AC9, (f) AC10.
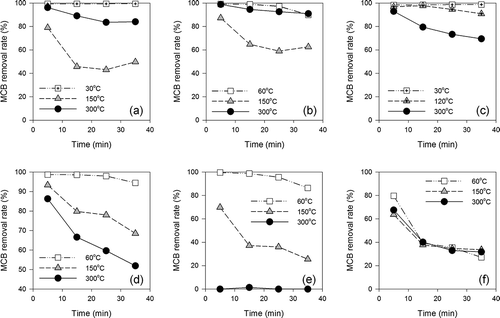
As shown in Figures 4c–4e , AC7–AC9 gave a higher MCB removal rate at lower temperature. This is typical of adsorptive removal. Adsorption is an exothermic reaction and hence MCB removal might decrease as the temperature increases. On the other hand, AC1–AC6 gave higher MCB removal rates at 300°C compared to those at 120 or 150°C ( and and ). This indicates the possibility of MCB decomposition by a catalytic reaction. Finally, AC10 showed low removal rates (<40%) except for the first 10 min, and no differences in MCB removal rates were observed for AC10 under the three different temperature conditions ().
On the basis of the results of average MCB removal rates for 40 min in low, medium, and high temperature conditions, the acid clays and activated clays were categorized into three groups, as shown in . Group I is the “decomposition type” and includes all natural acid clays (AC1–AC4) and two types of activated acid clays (AC5 and AC6). Group II is the “adsorption type” and includes three kinds of activated acid clays (AC7–AC9). Group III contains only AC10 and is defined as “low removal effect (low MCB removal rate regardless of temperature).” The activated acid clay was expected to have high dechlorination performance because of its proton-rich composition, but only two clays were classed as decomposition type. Conversely, all the natural acid clays used in this work were of the decomposition type.
Confirmation of MCB dechlorination by natural and activated acid clays
AC4 (natural acid clay) and AC5 (activated acid clay), which belong to Group I, were chosen to examine MCB removal by dechlorination. As already mentioned, the experiment was performed using 5 g clay with an MCB concentration more than 20 times higher (0.86 ± 0.17 mg/L) and an experimental time more than 3 times longer (120 min) than the MCB removal tests described earlier. The Cl contents of distilled water in the adsorption bottle and clay in the reactor were determined after the MCB removal test. The MCB dechlorination rate was calculated using the mass balance of Cl on a molar basis because 1 mole of detached Cl atoms corresponds to 1 mole of dechlorinated MCB.
As a result, chloride ions were not detected in the adsorption bottle, but were mainly detected in the filtrates obtained from washing the clay used in the MCB removal test. The Cl atoms detached from MCB might form chloride by reaction with the metals in the clay. As shown in , about 18% and 16% of the feed MBC was dechlorinated by AC4 and AC5, respectively, at 300 °C. Conversely, little or no Cl was detected in the filtrate from clays used for MBC removal tests at 150°C. This indicates that the dechlorination of MCB occurred at 300°C. However, the MCB removal rates at 300°C were significantly lower than the previous results obtained at the low MCB feed concentrations in the earlier described experiments. The amount of MCB dechlorinated per 1 g of clay and the dechlorination rates are as follows.
Table 2. The MCB dechlorination rate in AC4 (natural acid clay) and AC5 (activated acid clay)
0.6 mg/g-AC4 (ηAC4 = 94%) and 0.5 mg/g-AC5 (ηAC5 = 88%) at a low MCB feed concentration (38.5 ± 7.9 μg/L) with 1.5 g clay for 40 min.
1.3 mg/g-AC4 (ηAC4 = 18%) and 1.2 mg/g-AC5 (ηAC5 = 16%) at a high MCB feed concentration (0.86 ± 0.17 mg/L) with 5 g clay for 120 min.
A comparison of the two results reveals that the absolute amount of MCB dechlorinated per gram clay increased in the latter experiment, although the dechlorination rates decreased in the latter experiment. The low dechlorination rates were caused by the increased load of MCB per gram clay because of the higher MCB feed concentration and the longer reaction time.
Conclusion
Ten types of natural and activated acid clays were tested to confirm their MCB removal effects and mechanisms. On the basis of the MCB removal behavior from 30°C to 300°C, the clays were divided into adsorption, decomposition, and low removal effect types. More than half the clays (four natural acid clays and two activated acid clays) were classified as decomposition type. Cl atoms detached from MBC were confirmed to be present in the clay, which is evidence for the dechlorination of MCB at 300°C. Di- and trivalent metal oxides such as Al2O3, Fe2O3, MgO, and CaO might contribute to the dechlorination of MCB at 300°C. In this regard, the behavior of the Cl atom detached from MCB and its form (e.g., metal chloride) in clay should be investigated in future studies.
Natural and activated acid clays of the decomposition type are an alternative to activated carbon for the removal of DXNs or organochlorine compounds by dechlorination at 300°C. The latent risks related to the adoption of HTPC, for example, the de novo synthesis of DXNs, the decrease in adsorptive removal efficiency of DXNs, and extra treatment of fly ash to lower the concentration of DXNs, can be mitigated by using natural and activated acid clays of the decomposition type. As these clays are inorganic materials, safe use at 300°C is also expected. HTPC using natural and activated clay is an option for flue gas treatment to improve the energy recovery efficiency of MSWIs.
Additional information
Notes on contributors
In-Hee Hwang
In-Hee Hwang is an assistant professor, Shigetoshi Takahashi is a Ph.D. student, Takayuki Matsuo is a technician, and Toshihiko Matsuto is a professor at the Division of Environmental Engineering, Hokkaido University, Sapporo, Japan.
Shigetoshi Takahashi
In-Hee Hwang is an assistant professor, Shigetoshi Takahashi is a Ph.D. student, Takayuki Matsuo is a technician, and Toshihiko Matsuto is a professor at the Division of Environmental Engineering, Hokkaido University, Sapporo, Japan.
Takayuki Matsuo
In-Hee Hwang is an assistant professor, Shigetoshi Takahashi is a Ph.D. student, Takayuki Matsuo is a technician, and Toshihiko Matsuto is a professor at the Division of Environmental Engineering, Hokkaido University, Sapporo, Japan.
Toshihiko Matsuto
In-Hee Hwang is an assistant professor, Shigetoshi Takahashi is a Ph.D. student, Takayuki Matsuo is a technician, and Toshihiko Matsuto is a professor at the Division of Environmental Engineering, Hokkaido University, Sapporo, Japan.
References
- Ando, R., Y. Maeda, and R. Nishimura. 2009. About the performance examination of the dry process effluent gas disposal of effluent gas system generated from a Chinese city incinerator. Environ. Conserv. Eng. 38(2): 126–30. (in Japanese)
- Alvin, M. A. 1996. Advanced ceramic materials for use in high-temperature particulate removal systems. Ind. Eng. Chem. Res. 35:3384–98. doi:10.1021/ie960128i
- Furubayashi, M., R. Shinohara, and K. Nagai. 2002. Study on dioxin removal efficiency of various adsorbents. Kagaku Kougaku Ronbunshu 28:273–79. (Japanese) doi:10.1252/kakoronbunshu.28.273
- Gao, Y., H. Zhang, and J. Chen. 2010. Vapor-phase sorption of hexachlorobenzene on typical municipal solid waste (MSW) incineration fly ash, clay minerals and activated carbon. Chemosphere 81:1012–17. doi:10.1016/j.chemosphere.2010.09.008
- Heidenreich, S. 2013. Hot gas filtration—A review. Fuel 104:83–94. doi:10.1016/j.fuel.2012.07.059
- Hirokawa, A. 1980. Characteristics and applications of the acid-treated “acid clay. ” Nendokagaku 20:99–106. (in Japanese)
- Hussin, F., M. K. Aroua, and W. M. A. W. Daud. 2011. Textural characteristics, surface chemistry and activation of bleaching earth: A review. Chem. Eng. J. 170:90–106. doi:10.1016/j.cej.2011.03.065
- Japanese Ministry of Agriculture Forestry and Fisheries. 2008. Soil analysis method. http://www.maff.go.jp/j/seisan/kankyo/hozen_type/h_sehi_kizyun/pdf/manual1.pdf ( accessed May 2011).
- Kanda, N., I. Tanaka, T. Inaba, M. Itaya, and M. Yamamoto. 1998. Decomposition of Chlorinated Aromatic Compound by Catalytic Oxidation. Proceedings of 8th Kankyo Kougaki Sougou Symposium of Nihon Kikai Gakkai, Kawasaki, July 8–10, 182–84. (in Japanese)
- Komadel, P. 2003. Chemically modified smectites. Clay Miner. 38:127–38. doi:10.1180/0009855033810083
- Ninomiya, I., K. Ueda, K. Tanaka, M. Jige, H. Amimoto, and K. Tai. 2000. Removal of dioxins in flue gas from waste incinerators by catalytic oxidative process. Haikibutugakkaishi 11: 307–13. (in Japanese) doi:10.3985/jswme.11.307
- Pushpaletha, P., S. Rugmini, and M. Lalithambika. 2005. Correlation between surface properties and catalytic activity of clay catalyst. Appl. Clay Sci. 30:141–53. doi:10.1016/j.clay.2005.03.011
- Reijnen, K., and J. Van Brakel. 1984. Gas cleaning at high temperatures and high pressures: A review. Power Technol. 40:81–111. doi:10.1016/0032-5910(84)85057-3
- Saxena, S. C., R. F. Henry, and W. F. Podolski. 1982. Technology status of particulate removal from high-temperature high-pressure combustion gases. Aerosol Sci. Technol. 1:235–57. doi:10.1080/02786828208958591
- Stoll, M., J. Furrer, H. Seifert, G. Schaub, and D. Unruh. 2001. Effects of flue gas composition on the catalytic destruction of chlorinated aromatic compounds with a V-oxide catalyst. Waste Manage. 21:457–63. doi:10.1016/S0956-053X(00)00129-X
- Sugishima, N., and M. Kobayashi. 2001. Development of dioxins decomposition catalyst. Syokubai 43:559–64. (in Japanese)
- Ugochukwu, U. C., M. D. Jones, I. M. Head, D. A. C. Manning, and C. I. Fialips. 2013. Effect of acid activated clay minerals on biodegradation of crude oil hydrocarbons. Int. Biodeter. Biodegr. http://dx.doi.org/10.1016/j.ibiod.2013.10.018
- Weber, R., K. Nagai, J. Nishino, H. Shiraishi, M. Ishida, T. Takagusa, K. Konndo, and M. Hiraoka. 2002. Effects of selected metal oxides on the dechlorination and destruction of PCDD and PCDF. Chemosphere 46:1247–53. doi:10.1016/S0045-6535(01)00269-7
- Weber, R., T. Takasuga, K. Nagai, H. Shiraishi, T. Sakurai, T. Matsuda, and M. Hiraoka. 2002. Dechlorination and destruction of PCDD, PCDF and PCB on selected fly ash from municipal waste incineration. Chemosphere 46:1255–62. doi:10.1016/S0045-6535(01)00268-5
- Yang, C. C., S. H. Chang, B. Z. Hong, K. H. Chi, and M. B. Chang. 2008. Innovative PCDD/F-containing gas stream generating system applied in catalytic decomposition of gaseous dioxins over V2O5-WO3/TiO2-based catalysts. Chemosphere 73:890–95. doi:10.1016/j.chemosphere.2008.07.027