Abstract
Incineration flue gas contains polycyclic aromatic hydrocarbons (PAHs) and sulfur dioxide (SO2). The effects of SO2 concentration (0, 350, 750, and 1000 ppm), reaction temperature (160, 200, and 280 °C), and the type of activated carbon fibers (ACFs) on the removal of SO2 and PAHs by ACFs were examined in this study. A fluidized bed incinerator was used to simulate practical incineration flue gas. It was found that the presence of SO2 in the incineration flue gas could drastically decrease removal of PAHs because of competitive adsorption. The effect of rise in the reaction temperature from 160 to 280 °C on removal of PAHs was greater than that on SO2 removal at an SO2 concentration of 750 ppm. Among the three ACFs studied, ACF-B, with the highest microporous volume, highest O content, and the tightest structure, was the best adsorbent for removing SO2 and PAHs when these gases coexisted in the incineration flue gas.
Implications
Simultaneous adsorption of sulfur dioxide (SO2) and polycyclic aromatic hydrocarbons (PAHs) emitted from incineration flue gas onto activated carbon fibers (ACFs) meant to devise a new technique showed that the presence of SO2 in the incineration flue gas leads to a drastic decrease in removal of PAHs because of competitive adsorption. Reaction temperature had a greater influence on PAHs removal than on SO2 removal. ACF-B, with the highest microporous volume, highest O content, and tightest structure among the three studied ACFs, was found to be the best adsorbent for removing SO2 and PAHs.
Introduction
Incineration is the main technique for treating municipal solid waste in Taiwan. Some pollutants, including organic compounds, sulfur dioxide (SO2), heavy metals, and fly ash, may be emitted during incineration. To meet the stringent emission standards for various contaminants in Taiwan, a spray dryer integrated with a bag filter is used conventionally as an air pollution control device (APCD) for incinerators, and powdered activated carbon is injected into flue gas before it enters the bag filter in order to remove the organic compounds. However, the spray dryer suffers from some disadvantages, such as blockage of the spray nozzle and production of sludge. Therefore, it is important to devise a new technique to replace the conventional APCD for incinerators.
Adsorption techniques are widely used to remove organic compounds from industrial waste gas streams, and powdered activated carbon, activated carbon fibers (ACFs), aluminum oxide, silica gel, and zeolite are commonly used adsorbents. Among these adsorbents, ACFs offer many advantages, including higher adsorption and desorption rates, much higher surface areas, a uniform micropore structure, and a lower pressure drop (Fuertes et al., Citation2003; Huang et al., Citation2003; Lillo-Ródenas et al., Citation2005; Muñiz et al., Citation2000; Park and Kim, Citation2001; Yang et al., Citation2011). The use of ACFs for removing organic compounds from waste gas streams has been extensively studied, indicating that the removal efficiencies of organic compounds are affected by the specific surface area, pore size distribution, pore volume, and surface functional groups of the ACFs and by vapor pressure, molecular size, and molecular weight of organic compounds (Das et al., Citation2004; Gaur et al., Citation2006; Lin et al., Citation2013; Liu, Citation2006; Liu et al., Citation2012; Mangun et al., Citation2001; Yi et al., Citation2009).
However, most species of the organic compounds explored in previous studies are volatile organic compounds (VOCs), such as benzene, toluene, and xylene (Das et al., Citation2004; Gaur et al., Citation2006; Huang et al., Citation2003; Lin et al., Citation2013; Liu et al., Citation2012; Yi et al., Citation2009). The 16 species of polycyclic aromatic hydrocarbons (PAHs) listed by the U.S. Environmental Protection Agency (EPA) as priority pollutants are rarely discussed in research of ACFs (Liu, Citation2006). In this study, a fluidized bed incinerator was used to simulate practical incineration flue gas.
Incineration flue gas contains PAHs and SO2. The presence of SO2 in the incineration flue gas could affect the removal efficiency and adsorption capacity of PAHs. Liu (Citation2006) previously determined that the removal efficiency of PAHs from incineration flue gas using ACFs exceeded 90%. Previous research has shown that SO2 could be effectively removed by ACFs (Daley et al., Citation1997; Ling et al., Citation1999; Liu, Citation2008). For example, Liu et al. (Citation2012) investigated the simultaneous removal of toluene and SO2, and reported that SO2 inhibited the adsorption of toluene on ACFs and the removal of toluene decreased with an increase in the concentration of SO2. In this study, the simultaneous removal of PAHs and SO2 from incineration flue gas and the effect of SO2 on the removal of PAHs were examined at different SO2 concentrations (0, 350, 750, and 1000 ppm), reaction temperatures (160, 200, and 280 °C), and for three types of ACFs. Three adsorption temperatures, 160, 200, and 280 °C, were investigated because the temperature of flue gas in the APCD is 200 °C (Chi et al., Citation2006).
Experimental
Experimental procedure
The three commercial ACFs used in this experiment were provided by Taiwan Carbon Technology Co., Ltd., in Taiwan. Polyacrylonitrile (PAN) was used as a precursor in the production of these three ACFs. The thicknesses of ACF-A, ACF-B, and ACF-C were 0.4, 1.2, and 0.4 mm, respectively. The densities of ACF-A, ACF-B, and ACF-C were 0.22, 0.07, and 0.29 g·cm−3, respectively. The ACFs were cleaned using deionized water and then dried in an oven at 105 °C for 24 hr before the experiment was conducted. Thereafter, they were loaded into the ACF adsorber. The height and inner diameter of the ACF adsorber were 150 and 80 mm, respectively.
The laboratory-scale fluidized bed incinerator integrated with an ACF adsorber used in this study is shown in . The combustion chamber was heated to the desired temperature, 800 °C, by electrical heaters, and air entered the incinerator at a flow rate of 60 L·min−1. When the temperature reached a steady state, polypropylene (PP) granules and sulfur enclosed in a small polyethylene (PE) bag was thrown into the incinerator at the rate of one sample per 15 sec to simulate the flue gas. Subsequently, the ACF adsorber was turned on and sampling was initiated as described in the next paragraph. Detailed compositions of feedstock and operating parameters can be seen in .
Figure 1. Experimental system of fluidized-bed incinerator and activated carbon fibers (ACFs) adsorber: (1) air compressor; (2) flowmeter; (3) combustion chamber; (4) electrical heater; (5) thermal feedback controller; (6) thermocouple; (7) feeder; (8) ACF adsorber; (9) sampling; (10) induced fan.
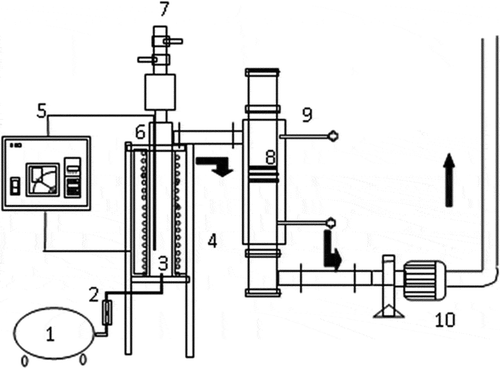
Table 1. Composition of feedstocks and operating conditions
Sampling and analytical methods
To estimate the removal efficiency of PAHs and SO2, the flue gas was sampled before entering and after exiting the ACF adsorber. The sampling time periods for SO2 after and before the passage of the flue gas through the ACF adsorber were 2–5 and 6–9 min, respectively. A flue gas analyzer (model 2800P; IMR Environmental Equipment Inc., Florida, USA) was used to determine the concentration of SO2. Before the experiments, the analyzer was calibrated with standard gases. The accuracy and resolution of the SO2 analyzer were 5% and 1 ppm, respectively. The concentration range for the SO2 monitor was 0–4000 ppm (Liu, Citation2008).
PAHs were sampled using the EPA Modified Method 5. The sampling time periods for PAHs after and before the passage of the flue gas through the ACF adsorber were 10–15 and 15–20 min, respectively. The sampling flow rate for PAHs was 10 L·min−1. The removal efficiency of PAHs was defined as follows:
Characterization of ACFs
The Brunauer-Emmett-Teller (BET) surface area and porosities of various ACFs were determined using an ASAP 2020 vacuum volumetric sorption instrument (Micromeritics Instrument Co., Georgia, USA) from the N2 adsorption-desorption isotherms at 77 K. Before N2 sorption analysis, the samples were preheated at 473 K for degassing and cooled at room temperature under vacuum conditions (Lin et al., Citation2013). The volume of the micropores (pores of size <2 nm) was calculated using the t-plot method. The mesopore volumes (pores of size 2–50 nm) were calculated by subtracting the micropore volumes from the total pore volume at P/P0 = 0.98. The surface functional groups of the ACFs were identified using a Fourier-transform infrared (FTIR) spectrometer (Digilab FTX350; American Digilab, Massachusetts, USA). The detailed FTIR analysis procedure is described in Liu (Citation2008). Additionally, the contents of C, N, O, and H on the ACFs were determined using an elemental analyzer (EA) (Elementar vario EL III; Hanau, Germany). A Zeiss Axioplan 2 microscope (Jena, Germany) equipped with an AxioCam HR color charge-coupled device camera (Jena, Germany) was used to analyze the photomicrographs of the ACFs.
Results and Discussion
Characterization of ACFs
The detailed specific surface area and porosity of the three ACFs, determined through N2 sorption analysis, can be viewed in . The three ACFs have similar specific surface area and total pore volume. Whereas the micropore and mesopore volumes of ACF-A and ACF-C are similar, the micropore and mesopore volumes of ACF-B are higher and lower, respectively, than the similar corresponding volumes of ACF-A and ACF-C.
Table 2. Specific surface area and porosity of activated carbon fibers (ACFs) by N2 isotherms
The FTIR spectra of the three ACFs can be viewed in . The spectra show peaks in the range 900–1300 cm−1, which correspond to both –C–O stretching and –O–H bending modes, and at ˜1600 cm−1, which corresponds to the –C=O groups (Fanning and Vannice, Citation1993; Gaur et al., Citation2006; Lin et al., Citation2013). Bands at ˜2380 cm−1 and at ˜3800 cm−1 in the FTIR spectrum of ACF-A are assigned to the ketene group (=C=O) and the nitrogen species, respectively (Gaur et al., Citation2006; Tsuji and Shiraishi, Citation1997). lists the elemental analysis of the three ACFs and indicates that the O content of the three ACFs follows the order of ACF-B > ACF-C > ACF-A.
Table 3. Element content of the three activated carbon fibers (ACFs) by an elemental analyzer
It is clear from that the knitted structure of ACF-B is tighter than that of ACF-A and ACF-C, which might affect its efficiency in removing SO2 and PAHs. SO2 and PAHs can be absorbed onto fly ash, and ACFs with tighter structures are very efficient at removing fly ash. In addition to the tightness of ACF knitted structures, the pore volume and surface functional groups of ACFs may affect the adsorption capacities and mechanisms of SO2 and PAHs on ACFs. Other than type of ACFs, the presence of SO2 in the incineration flue gas can decrease PAH removal drastically because of competitive adsorption. Additionally, reaction temperature may also affect the adsorption capacities and mechanisms of SO2 and PAHs on ACFs. Additional detail regarding the effects of SO2 concentration, reaction temperature, and the type of ACFs on the simultaneous removal of SO2 and PAHs will be provided below.
Effect of SO2 concentration on PAH removal
The effects of SO2 concentration on the removal efficiencies of SO2 and PAHs are apparent in . The results indicate that the removal efficiency of PAHs is 90% in the absence of SO2. When the concentration of SO2 is 350 ppm, the removal efficiencies of SO2 and PAHs are 92% and 33%, respectively. The presence of SO2 in the incineration flue gas leads to a drastic decrease in removal of PAHs because of competitive adsorption. These results are in agreement with those of Liu et al. (Citation2012), which reported that the presence of SO2 decreased the removal of toluene because the polarity and the dipole moment of SO2 were stronger than those of toluene. Hence, SO2 first adsorbs onto a polar or basic adsorption site and then oxidizes to SO3 in the presence of O2, which prevents the PAH removal efficiency from increasing.
Figure 5. Effects of SO2 concentration on the removal efficiencies of SO2 and PAHs (reaction condition: reaction temperature = 200 °C, ACF type: ACF-A).
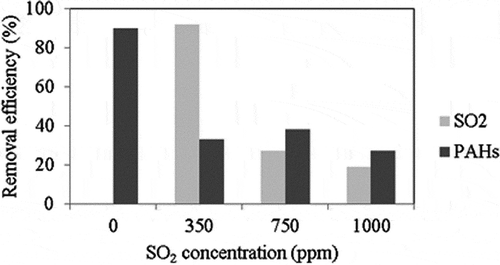
shows that the removal efficiency of SO2 decreases markedly when the concentration of SO2 increases to 750 ppm, and beyond this concentration no changes are apparent in removal of PAHs with increasing SO2 concentration. This may occur because SO2 was first adsorbed by ACFs when SO2 and PAHs coexisted in the incineration flue gas and the effect of SO2 concentration on SO2 removal was greater than that on PAH removal. Further, when the concentration of SO2 became very high, the molecules of SO2 collided with each other, thereby reducing the number of opportunities for SO2 not only to diffuse from the surface of the ACFs into the pores in the ACFs, but also to be adsorbed onto the ACFs (Liu, Citation2008). As a result, the removal efficiency of SO2 decreased with increasing SO2 concentration.
Effect of reaction temperature on SO2 and PAH removal
The effects of reaction temperature on the removal efficiencies of SO2 and PAHs are shown in . SO2 removal is unaffected by an increase in temperature from 160 to 280 °C at an SO2 concentration of 750 ppm. Liu (Citation2008) previously reported that an increase in reaction temperature from 150 to 260 °C had a considerable effect on SO2 removal using ACFs modified with HNO3 at an SO2 concentration of 200 ppm, with the highest SO2 removal efficiency observed of 80% at 200 °C. In this study, changes in the reaction temperature had less of an influence on SO2 removal at a high SO2 concentration (750 ppm) than at a low SO2 concentration (200 ppm). This may be so because the removal efficiency of SO2 at a high SO2 concentration of 750 ppm (<30%) was too low to be affected by the reaction temperature.
Figure 6. Effects of reaction temperature on the removal efficiencies of SO2 and PAHs (reaction condition: SO2 concentration = 750 ppm, ACF type: ACF-A).
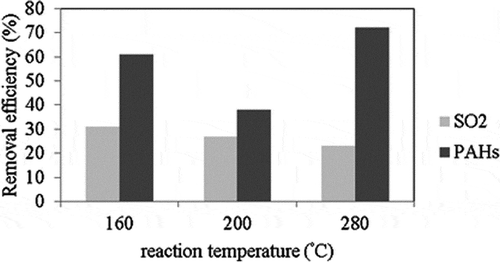
The results also show that the removal efficiency of PAHs follows the order of 280 °C > 160 °C > 200 °C. The concentration of two-ring PAHs (naphthalene) is much higher than the concentrations of other PAH species in incineration flue gas. The boiling point of naphthalene is 218 °C, which is close to 200 °C (Liu, Citation2006). Thus, the naphthalene is removed by ACFs by condensation at 160 °C. However, the condensation of naphthalene on the ACF surface would block the pores on the ACF surface, thus decreasing the PAH removal. Further, because of the blocking of the pores on the ACF surface, the micropores inside the ACFs cannot be completely utilized during the adsorption process. Therefore, 280 °C was the optimum temperature for the adsorption of PAHs on ACF-A in this study. Reaction temperature had a greater influence on PAH removal than on SO2 removal when SO2 and PAHs coexisted in the incineration flue gas. Liu (Citation2006) previously confirmed that the adsorption of PAHs on ACFs is attributed to condensation and physical adsorption, whereas the adsorption process between PAHs and fly ash is attributed to chemical adsorption.
Effect of type of ACFs on SO2 and PAH removal
shows the effects of different types of ACFs on the removal efficiencies of SO2 and PAHs at 200 °C, because in practice the temperature of flue gas entered into the APCD of incinerators is 200 °C. Removal efficiencies of SO2 and PAHs when ACF-A was used as the adsorbent were similar to the corresponding values when ACF-C was used. When ACF-B was used as the adsorbent, the removal efficiencies of SO2 and PAHs increased to 54% and 89%, respectively. Removal efficiencies of SO2 and PAHs were the highest when ACF-B was used, which is in accordance with the order of micropore volume of the ACFs (see ). confirms that the three ACFs have similar specific surface area and total pore volume. It can therefore be concluded that the removal of SO2 and PAHs depends mainly on the micropore volume of the ACFs reported in this study.
Figure 7. Effects of different ACF types on the removal efficiencies of SO2 and PAHs (reaction condition: SO2 concentration = 750 ppm, reaction temperature = 200 °C).
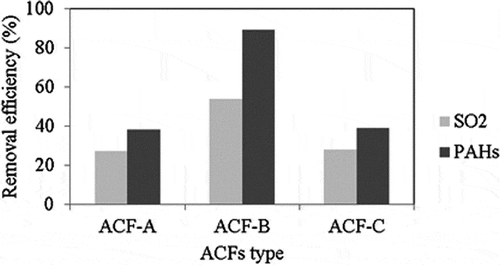
Liu (Citation2006) previously confirmed that micropore volume plays an important role in determining the removal of gaseous PAHs when the concentration of PAHs is low and the BET surface area is sufficient for PAH removal. Kisamori and Mochida (Citation1994) previously demonstrated that a certain amount of CO2 was released by the decomposition of O functional groups at 200 °C, thus reducing the amount of active sites but increasing the mesopore volume and, in turn, increasing the storage capacity of H2SO4 on the ACFs. As can be seen in , the O content of ACF-B was the highest among the three ACFs.
It is possible to simultaneously remove fly ash and PAHs from incinerator flue gas by using ACFs because PAHs can be condensed or adsorbed on fly ash and ACFs are very efficient in removing fly ash. Further, since ACF-B has the tightest knitted structure among the three ACFs, fly ash can be separated effectively by ACF-B. Thus, the removal of the PAHs, which are adsorbed on fly ash, will improve because of the tight structure of ACF-B. Thus, ACF-B, with the highest microporous volume, highest O content, and tightest structure among the three ACFs, was the best adsorbent for removing SO2 and PAHs when they coexisted in incineration flue gas.
Conclusions
The simultaneous removal of PAHs and SO2 from incineration flue gas was examined in this study. The effect of SO2 on the removal of PAHs and the effects of reaction temperature and type of ACFs on removal of SO2 and PAHs were determined. The results indicate that the removal efficiency of PAHs by using ACF-A was 90% in the absence of SO2. When the concentration of SO2 was 350 ppm, the removal efficiencies of SO2 and PAHs by using ACF-A were 92% and 33%, respectively. The presence of SO2 in the incineration flue gas can decrease the removal of PAHs drastically because of competitive adsorption onto the ACFs. However, removal of PAHs was insignificant when the concentration of SO2 increased from 350 ppm to greater than 750 ppm (SO2 concentration up to 1000 ppm were examined). Moreover, the variation in SO2 removal was insignificant at an SO2 concentration of 750 ppm when the temperature increased from 160 to 280 °C, and the removal efficiency of PAHs followed the order 280 °C > 160 °C > 200 °C. ACF-B, with the highest microporous volume, highest O content, and tightest structure among the three studied ACFs, was found to be the best adsorbent for removing SO2 and PAHs when these gases coexisted in incineration flue gas.
Funding
The authors thank the National Science Council of the Republic of China (Taiwan) for financially supporting this research under contract no. NSC 96-2211-E-131-019-MY3.
Additional information
Notes on contributors
Zhen-Shu Liu
Zhen-Shu Liu is an associate professor, Wen-Kai Li was a postgraduate student, and Ming-Jui Hung is an assistant professor in the Department of Safety, Health and Environmental Engineering at the Ming Chi University of Technology, New Taipei City, Taiwan.
Wen-Kai Li
Zhen-Shu Liu is an associate professor, Wen-Kai Li was a postgraduate student, and Ming-Jui Hung is an assistant professor in the Department of Safety, Health and Environmental Engineering at the Ming Chi University of Technology, New Taipei City, Taiwan.
Ming-Jui Hung
Zhen-Shu Liu is an associate professor, Wen-Kai Li was a postgraduate student, and Ming-Jui Hung is an assistant professor in the Department of Safety, Health and Environmental Engineering at the Ming Chi University of Technology, New Taipei City, Taiwan.
References
- Chi, K.H., S.H. Chang, C.H. Huang, H.C. Huang, and M.B. Chang. 2006. Partitioning and removal of dioxin-like congeners in flue gases treated with activated carbon adsorption. Chemosphere 64:1489–1498. doi:10.1016/j.chemosphere.2005.12.072
- Daley, M.A., C.L. Mangun, J.A. Debarr, S. Riha, A.A. Lizzio, G.L. Donnals, and J. Economy. 1997. Adsorption of SO2 onto oxidized and heat-treated activated carbon fibers (ACFs). Carbon 35:411–417. doi:10.1016/S0008-6223(97)89612-1
- Das, D., V. Gaur, and N. Verma. 2004. Removal of volatile organic compound by activated carbon fiber. Carbon 42:2949–2962. doi:10.1016/j.carbon.2004.07.008
- Fanning, P.E., and M.A. Vannice. 1993. A DRIFTS study of the formation of surface groups on carbon by oxidation. Carbon 31:721–730. doi:10.1016/0008-6223(93)90009-Y
- Fuertes, A.B., G. Marban, and D.M. Nevskaia. 2003. Adsorption of volatile organic compounds by means of activated carbon fibre-based monoliths. Carbon 41:87–96. doi:10.1016/S0008-6223(02)00274-9
- Gaur, V., A. Sharma, and N. Verma. 2006. Preparation and characterization of ACF for the adsorption of BTX and SO2. Chem. Eng. Process. 45:1–13. doi:10.1016/j.cep.2005.04.006
- Huang, Z.H., F. Kang, K.M. Liang, and J. Hao. 2003. Breakthrough of methyethylketone and benzene vapors in activated carbon fiber beds. J. Hazard. Mater. B98:107–115. doi:10.1016/S0304-3894(02)00284-4
- Kisamori, S., and I. Mochida. 1994. Roles of surface oxygen groups on poly(acrylonitrile)-based active carbon fibers in SO2 adsorption. Langmuir 10:1241–1245. doi:10.1021/la00016a043
- Lillo-Ródenas, M.A., D. Cazorla-Amorós, and A. Linares-Solano. 2005. Behaviour of activated carbons with different pore size distributions and surface oxygen groups for benzene and toluene adsorption at low concentrations. Carbon 43:1758–1767. doi:10.1016/j.carbon.2005.02.023
- Lin, C.L., Y.H. Cheng, Z.S. Liu, and J.Y. Chen. 2013. Adsorption and oxidation of high concentration toluene with activated carbon fibers. J. Porous Mater. 20:883–889. doi:10.1007/s10934-012-9665-z
- Ling, L., K. Li, L. Liu, S. Miyamoto, Y. Korai, S. Kawano, and I. Mochida. 1999. Removal of SO2 over ethylene tar pitch and cellulose based activated carbon fibers. Carbon 37:499–504. doi:10.1016/S0008-6223(98)00219-X
- Liu, Z.H., J.R. Qiu, H. Liu, Z.Q. Tan, Z.Q. Yan, M.I. Zhang, H.C. Zeng, and H. Yang. 2012. Effects of SO2 and NO on removal of VOCs from simulated flue gas by using activated carbon fibers at low temperatures. J. Fuel Chem. Technol. 40:93–99. doi:10.1016/S1872-5813(12)60008-5
- Liu, Z.S. 2006. Control of PAHs from incineration by activated carbon fibers. J. Environ. Eng. 132:463–469. doi:10.1061/(ASCE)0733-9372(2006)132:5(463)
- Liu, Z.S. 2008. Adsorption of SO2 and NO from incineration flue gas onto activated carbon fibers. Waste Manage. 28:2329–2335. doi:10.1016/j.wasman.2007.10.013
- Mangun, C.L., K.R. Benak, J. Economy, and K.L. Foster. 2001. Surface chemistry, pore size and adsorption properties of activated carbon fibers and precursors treated with ammonia. Carbon 39:1809–1820. doi:10.1016/S0008-6223(00)00319-5
- Muñiz, J., G. Marbán, and A.B. Fuertes. 2000. Low temperature selective catalytic reduction of NO over modified activated carbon fibres. Appl. Catal. B 27:27–36. doi:10.1016/S0926-3373(00)00134-X
- Park, S.J., and K.D. Kim. 2001. Influence of activation temperature on adsorption characteristics of activated carbon fiber composites. Carbon 39:1741–1746. doi:10.1016/S0008-6223(00)00305-5
- Tsuji, K., and I. Shiraishi. 1997. Combined desulfurization, denitrification and reduction of air toxics using activated coke. Fuel 76:549–553. doi:10.1016/S0016-2361(97)00010-0
- Yang, Y., M.H. Zhou, G. Xue, and Z.X. Jiang. 2011. Preparation and characterization of PAN-based ultra-fine activated carbon fiber adsorbent. J. Porous Mater. 18:379–387. doi:10.1007/s10934-010-9388-y
- Yi, F.Y., X.D. Lin, and S.X. Chen. 2009. Adsorption of VOC on modified activated carbon fiber. J. Porous Mater. 16:521–526. doi:10.1007/s10934-008-9228-5