Abstract
Exhaust gas particle and ion size distributions were measured from an off-road diesel engine complying with the European Stage IIIB emission standard. The measurements were performed at idling and low load conditions on an engine dynamometer. Nucleation-mode particles dominated the diesel exhaust particle number emissions at idle load. The nonvolatile nucleation-mode geometric mean diameter was detected at 10 nm or below. The nonvolatile nucleation-mode charge state implied that it has evolved through a highly ionizing environment before emission from the engine. The determined charging probabilities were 10.0 ± 2.2% for negative and 8.0 ± 2.0% for positive polarity particles. The nonvolatile nucleation particle concentration and size was also shown to be dependent on the lubricant oil composition. The particle emissions were efficiently controlled with a partial filter or with partial filter + selective catalytic reduction (SCR) combination. The particle number removal efficiencies of the aftertreatment systems were over 95% for wet total particle number (>3nm) and over 85% for dry particle total number. Nevertheless, the aftertreatment systems’ efficiencies were around 50% for the soot-mode particles. The low-load nonvolatile nucleation particle emissions were also dependent on the engine load, speed, and fuel injection pressure. The low load particle number emissions followed the soot-core trade-off, similar to medium or high operating loads.
Implications:
Idling and low-load diesel engine exhaust emissions affect harmfully the ambient air quality. The low-load particle number emissions are here shown to peak in the 10-nm size range for a modern off-road engine. The particles are electrically charged and nonvolatile and they originate from the combustion process. Tailpipe particle control by open channel filter can remove more than 85% of the nonvolatile 10-nm particles and about 50% of the soot-mode particles, while the fuel injection pressure increase can lead to particle number increase. The study provides a new viewpoint for low-load particle emissions and control.
Introduction
Idling and low-load diesel engine exhaust emissions are harmful to air quality in various environments (Weijers et al., Citation2004; Storey et al., Citation2006; Rasdorf et al., Citation2010). Combustion engines are allowed to idle and are used at low loads during cold weather conditions, at parking and working areas, and in congestion. Thus, engine idling emissions take place in populated areas, and can lead to a substantial inhalation exposure of diesel exhaust components (Tang and Wang, Citation2006; Wheatley and Sadhra, Citation2004).
The idling diesel engine exhaust emission characteristics differ from the exhaust emissions at operating conditions (e.g., Tong, Hung, and Cheung, Citation2000). Carbon monoxide and gaseous hydrocarbon emissions are notable at idle compared to those at operating loads (Tong, Hung, and Cheung, Citation2000; Merritt et al., Citation2005; Brodrick et al., Citation2002). Idle exhaust hydrocarbon emissions contain noncombusted fuel compounds and the volatile organics dominate the particle mass emissions. Soot mass emissions, on the other hand, are often considerably lower at idle than at operating loads (Kinsey et al., Citation2007; Lindgren et al., Citation2011).
Nevertheless, the low soot mass emissions do not directly imply low particle number emissions. The idling diesel particle number size distribution is systematically detected to peak around 10 nm to 20 nm particle size range at a relatively high concentration (Kittelson, Watts, and Johnson, Citation2006; De Filippo and Maricq, Citation2008; Sgro et al., Citation2012; Park et al., Citation2012; Li et al., Citation2008). This so-called nucleation particle mode cannot, however, be directly connected to the volatile organic fraction, but the nucleation-mode particles are shown to include a nonvolatile fraction and an electrical charge (Sgro et al., Citation2012; De Filippo and Maricq, Citation2008). Two different formation routes, namely, hydrocarbon and lubricant metal nucleation, are suggested for the nonvolatile nucleation-mode particles and both of them are considered to take place in the engine cylinder (De Filippo and Maricq, Citation2008; Abdul-Khalek et al., Citation1998a).
Here idle and low-load particle number emissions are presented for a Stage IIIB off-road diesel engine. First the idle particle emission physical characteristics are presented, and then the effects of tailpipe aftertreatment and fuel injection pressure adjustment on the particle emission are studied. Last, a quick view on the effect of small engine speed and torque changes at low loads is taken. The study aims to provide a new view of the off-road engine idle and low-load particle emission and emission control, and thus to contribute to a development of particle emission control and exposure assessment.
Experimental
Particle and ion size distributions were measured in a dynamometer laboratory from a Stage IIIB, off-road engine exhaust at idling and low-load conditions. The measurements were performed without aftertreatment and with two different tailpipe aftertreatment systems. The particle emission characteristics were studied with particle charge state and volatility measurements. The studied control methods included fuel injection variation in addition to the studied tailpipe aftertreatment systems. At idle load, the effect of two different lubricant oils on the particle emission was compared.
Sampling and measurement equipment
A two-stage partial flow dilution was applied in the study. The first diluter was a porous tube-type diluter (Mikkanen et al., Citation2001). The primary dilution air temperature was 30ºC and the dilution ratio was around 12. The dilution was adjusted with a bypass flow and was determined from the CO2 ratio between the raw and diluted exhaust, with background CO2 removed. The secondary dilution was conducted with an ejector diluter after a residence time chamber, and the total dilution ratio was close to 96. The sampling system was identical to that used in other references (Vaaraslahti et al., Citation2004; Rönkkö et al., Citation2007; Lähde et al., Citation2011).
Following the terminology used in Lähde et al., (Citation2011), we name the size distributions measured directly after the dilution as wet and after an evaporative treatment, such as the thermodenuder described in the following, as dry, nonvolatile, or as a core in case of nonvolatile nucleation mode.
The particle size distributions were measured with two scanning mobility particle sizers (SMPSs) (Wang and Flagan, Citation1989), a nano-SMPS (Chen et al., Citation1998; UCPC 3025A, DMA 3085, TSI, Inc.) and a long-SMPS (DMA 3081, CPC 3775, TSI, Inc.). The measurement size ranges were from 3 nm to 60 nm and from 12 nm to 400 nm for the nano-SMPS and the long-SMPS, respectively, and the scan times were 150 sec for both of them. The losses were corrected for the nano-SMPS only, yet the long-SMPS concentrations were scaled to match the nano-SMPS concentration close to a soot-mode modal diameter. An electrical low pressure impactor (Keskinen et al., Citation1992; Dekati Ltd.) was used to monitor the emission during the measurements.
The particle volatility was studied with a thermodenuder (TD) (Burtscher et al., Citation2001). The TD used was designed for particles with diameter below 30 nm, and a loss correction was applied for the particle size distributions measured after the TD (Heikkilä et al., Citation2009).
Particle charge state was studied by measuring particle size distributions with and without a neutralizer with a differential mobility particle sizer (iDMPS) (Sgro et al., Citation2012). Different ion polarities were measured by changing the polarity of the high-voltage source. The DMA and the CPC in iDMPS were of similar design as those used in nano-SMPS. The sheath and sample flows used were 15 LPM and 1.5 LPM, respectively.
When the ion distribution data are considered, one should note that the mobility diameter or the shape of the number size distribution cannot be extracted from the electrical mobility data in the size range where particles carry multiple charges. In the size range around 10 nm, however, the probability for multiple charging is negligible and the data measured with the DMPS, with or without the neutralizer, can be considered to represent the concentration of the particles with the particular mobility equivalent diameter.
Engine, lubricant, fuel, and driving conditions
The studied research engine was the same as in our previous study (Lähde et al., Citation2011), a 6-cylinder, 7.4-L, intercooled, turbocharged, common-rail injection off-road engine with 16.7:1 compression ratio and a maximum torque of 1119 N-m at 1500 min−1 and a maximum power of 182 kW at 2200 min−1. The engine meets Stage IIIB exhaust emission limits with selective catalytic reduction (SCR) aftertreatment according to the manufacturer. Here also two different after-treatments were studied, namely, a partial filter and partial filter–selective catalytic reduction catalytic converter (SCR) combination. The partial filter was of DOC-POC type (DOC-POC, EcoCat Ltd.), where the diesel oxidation catalyst (DOC) was inserted before the partial-filter section. The filter consisted of corrugated and catalyzed fine metallic mesh screen rolled in a tight cylinder forming open but bendy tortuous channels; see Vakkilainen and Lylykangas (Citation2004).
The results presented here for the partial filter should be considered in the context of engine parameters and the aging of the filter. Although the removal efficiency of the partial filter type used here is shown to remain quite stable even over 300,000 km of use (Lehtoranta et al., Citation2009), there can be changes in the efficiency at the lowest particle size regime. One should also note that if temperature and flow rate (here 140ºC and 170 kg/hr) of exhaust differ from the tests considered here, the efficiency of the partial filter can be different too.
The SCR catalyst was a monolithic catalyst with vanadium loading. In this study, the exhaust gas temperatures were well below 200ºC for all the studied conditions and no urea injection was applied during the measurements.
Two different development-stage lubricants were used in the study. Both of them were modern low-ash, low-sulfur oils. In oil 1 the base oil was purely synthetic, while in oil 2 the base was a mixture of traditional mineral oil and the same synthetic base oil (30–40%) as in oil 1. The sulfur content of the oil 1 was 3530 mg/kg and 6310 mg/kg for the oil 2. The Ca concentrations were 3530 mg/kg and 4820 mg/kg for oil 1 and oil 2, respectively. The P and Zn levels of the two oils were practically identical. A more detailed table of the oil composition and character is presented later, as . The sulfur content of the commercial fuel used as test fuel was 12.5 ppm.
The whole measurement campaign was dedicated for steady-state engine load condition measurements. All the results presented here were measured with a fully warmed-up engine. The steady engine conditions were run between 25 min to 58 min per measured engine condition, so that the engine was stabilized before measurements. The fuel injection variation and the engine torque and speed variation were conducted using small steps.
Results and Discussion
Particle emission characteristics
A bimodal size distribution was measured at idling engine condition for lubricant 1; see . Both wet and dry size distributions were bimodal, while the wet nucleation-mode modal diameter was larger than the dry core modal diameter and similarly the number of the nucleation-mode particles detected at wet basis was higher than the detected core number.
Figure 1. Wet and dry size distributions at idle without an after treatment system, oil 1. The nano-SMPS measurements are shown with large markers and the long-SMPS measurements are shown with smaller markers. Thin vertical lines show the variation of the measured distributions (min-max).
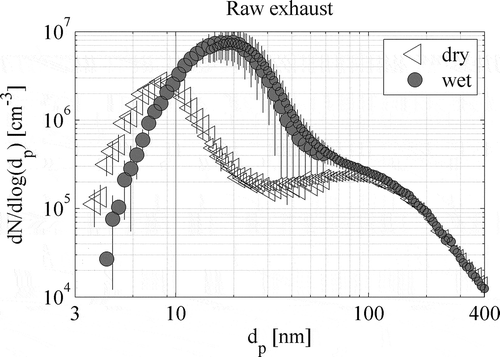
Similar exhaust particle distributions were also detected previously for different types of diesel and gasoline direct injection engines’ exhaust at idle load conditions (Kittelson, Watts, and Johnson, Citation2006; De Filippo and Maricq, Citation2008; Sgro et al., Citation2012; Park et al., Citation2012; Li et al., Citation2008). For example, Kittelson et al. (Citation2006) detected wet nucleation mode and a residue of the nucleation mode after a thermodenuder at idling load conditions from heavy-duty diesel vehicle exhaust. De Filippo and Maricq (Citation2008), on the other hand, detected the core mode from an idling light duty vehicle exhaust and Sgro et al. (Citation2012) from a gasoline direct injection (GDI) engine at idling load conditions. The core was detected in all of these studies without an aftertreatment system, and De Filippo (Citation2008) detected the idle core also after a diesel oxidation catalyst.
The concentration, size, and width of the idle load nucleation mode are shown to vary. In our case, the geometric mean diameter (GMD) of the core mode was around 9 nm, while the wet nucleation mode GMD was around 18 nm. Similarly the dry core number concentration was around while the wet nucleation-mode concentration was close to 3.5
, whereas the geometric standard deviation was around 1.4 for the dry and 1.5 for the wet mode. Sgro et al. (Citation2012) showed for a GDI engine that the core-mode diameter was below 5 nm at high primary dilution ratio (DR) (160) but above 5 nm for low DR (40). The distribution was wider in the low DR than in the high DR case. The number concentration, however, did not change with DR. Similar results for a locomotive engine were shown by Park et al. (Citation2012). De Filippo and Maricq (Citation2008) showed that the core diameter was engine and exhaust gas recirculation dependent but below 10 nm for two studied engines and the wet nucleation-mode modal diameter around or above 10 nm. Also in De Filippo’s study, the wet distribution was wider and the wet nucleation particle concentration higher than the dry concentration. In the study by Kittelson et al. (Citation2006) the measurement size range was limited to 7 nm and only the lowering tail of the nonvolatile nucleation mode was detected there. Also there, the wet nucleation mode was detected close to 20 nm.
In all the studies, the wet nucleation-mode concentration was higher than the dry nucleation-mode concentration. Homogeneous nucleation in the dilution, nevertheless, does not seem to be a probable reason for the difference. The conditions were not favorable for the most obvious candidate, the binary nucleation of water and sulfuric acid. In all of the preceding cases, the fuel sulphur content (FSC) was low: In this study the FSC was 12 ppm, in De Filippo and Maricq (Citation2008) between 9 ppm and 23 ppm, in Sgro et al. (Citation2012) study below 10 ppm, and in Kittelson as low as 1 ppm. Additionally, the measurements were conducted without an aftertreatment system at low exhaust temperatures (in this study the idle exhaust temperature was 140ºC after the turbocharger turbine), thus leading to low SO2 to SO3 conversion and low probability for H2SO4-mediated nucleation (Lemmetty et al., Citation2006, pp. 1596–1604).
More exotic nucleation processes are of course possible, but we offer an explanation that does not involve unidentified processes. We believe that the difference is caused by the limited detection efficiencies of the used instruments and, partly, by the size-dependent losses in the sampling line. Thereby, the difference in the concentration can be explained by particle growth to the size range where the measurement equipment has higher detection efficiency and also particle losses are lower. This would indirectly imply that in diesel vehicles also, a large proportion of the particles is around or below 3 nm particle diameter, similar to that found with the GDI engine (Sgro et al., Citation2012).
In the present study, the wet accumulation-mode number seems also higher than the dry soot-mode number (). This, however, is due to the overlapping of the wet nucleation and the accumulation modes. In both wet and dry size distributions, the smaller particle mode was, nevertheless, clearly dominant mode when the particle number is considered. For further examination, see the distributions measured with aftertreatment systems below. The soot-mode total number concentration was in this study around ; see and later, the inset in .
Particle electrical charge state
The idle nucleation mode was also electrically charged. In (left) the measured dry DMPS distributions with and without neutralizer are shown for both electrical polarities. Both ion polarities with and without neutralizer peaked at the same voltage step, close to 10 nm, although when the log-normal distributions were fitted to the data, the positive ion distributions GMDs were slightly smaller than the negative ion distributions GMDs. The GMDs for neutralized and natural ion distributions were equal for each electrical polarity; see . Interestingly, the total negative natural ion concentration was higher than the positive ion concentration. This differs from the results by De Filippo and Maricq (Citation2008), who reported charge equilibrium between the polarities for the core. The imbalance seems, however, rather expectable as the ion attachment produces a molecular-ion-dependent, biased charge state to particle population (Hoppel and Frick, Citation1986). In fact, the negative and the positive ion distributions differ little from each other also in De Filippo and Maricq (Citation2008; see in the paper). Note that the ion concentrations of have not been corrected for multiple charges. The interference caused by multiply charged soot-mode particles is estimated to be quite small because the ion concentration in the soot mode is lower than in the nucleation mode. Furthermore, the fraction of multiply charged particles at the relevant particle diameters is low (see Maricq, Citation2006).
Figure 2. Left: Dry ion concentrations with (neutralized) and without (ion) neutralizer at idling load condition for two polarities (+, –), oil 1. The vertical lines show variation of the measured distributions. Dashed lines show the lognormal fits for the data. Dilution-corrected data. Right: Calculated charging probability values for the measured diameters (open symbols). The solid lines show the best Boltzmann equilibrium charging fits for the data (FBneg,FBpos). The fitted Boltzmann temperatures were 875 K and 745 K for negative and positive polarities, respectively. The dashed lines show average theoretical (Fuchs, Citation1963; Hoppel and Frick, Citation1986) charging probability values (fn) for the neutralizer, used in the calculation. The thin dashed lines represent variation caused by different ion parameters (Reischl et al., Citation1996).
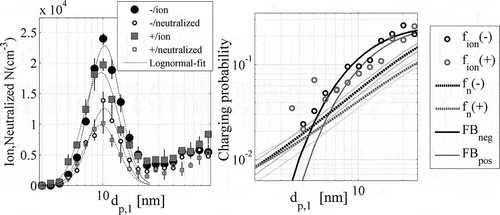
Figure 3. Dry distributions (nano-SMPS) for lubrication oils 1 and 2 at idle from raw exhaust. Long-SMPS distributions are presented in the inset to show the soot-mode distributions.
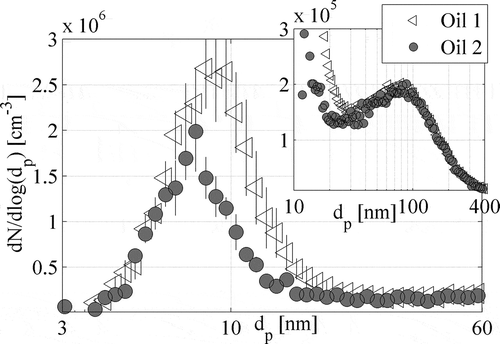
Table 1. Ion average lognormal-distribution parameters (geometric standard deviation GSD, geometric mean diameter GMD, ion concentration N) for both polarities with and without neutralizer (with uncertainties approximated from the data series maximums and minimums), and the calculated charging probabilities,
Figure 4. Right: Dry and wet particle size distributions with partial filter (A) and with partial filter and selective catalytic reduction catalytic converter (SCR) combination (B). Left: Average dry particle removal efficiencies for the aftertreatment systems E, and total particle number removal efficiencies for dry ET(dry) and wet ET(wet) particle emissions, oil 1.
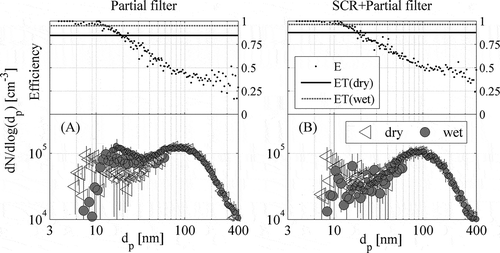
Charging probability and Boltzmann temperature
The natural ion charging probabilities, , were calculated with the equation
, where
is the measured natural ion concentration,
is the measured ion concentration after the neutralizer, and
is the average charging efficiency for the neutralizer, each for
range between 3 nm and 30 nm. The equation was solved separately for both polarities. The natural ion charging probability was solved with the method presented by Fuchs (Citation1963) and later slightly modified by Hoppel and Frick (Citation1986) for a set of different molecular ion mobilities and masses (Reischl et al., Citation1996, ). The thick dashed lines shown in (right) are the calculated neutralizer charging probabilities for both polarities, and the thin dashed lines show the corresponding standard deviations.
The core charge state was also estimated from lognormal-fit parameters, namely, with total concentration and geometric mean diameter. In this way, an average charging probability for the mode can be calculated. The estimation was conducted by substituting the fit total concentrations for the natural ion and neutralized ion distributions to the equation already given, and by calculating the neutralizer average charging probability at the fit geometric mean diameter for both polarities. The core average charging probabilities for the naturally charged ions were 10 2.2% for negative and 8.0
2.0% for positive polarity ().
The Boltzmann temperatures were solved by fitting the Boltzmann charge probability equation to the calculated data, shown by the open circles in (right). The natural ion Boltzmann charge equilibrium temperature was for negative polarity 875 K and for positive polarity 745 K. The temperatures are in line with the temperature estimated for a light-duty diesel vehicle (580ºC; De Filippo and Maricq, Citation2008) or for a GDI engine with air-to-fuel ratio around 1.1 (roughly around 800 K; Sgro et al., Citation2012), but lower than the temperature estimates given for GDI engine with air-to-fuel ratio at 1.2 (close to 2000 K; Sgro et al., Citation2012) Additionally, the estimate is lower than the approximate maximum flame temperatures, around 2000 K, in the combustion engine (Heywood, Citation1989).
The difference between the maximum flame temperatures and the approximated particle charge state Boltzmann temperatures has been already discussed widely in previous studies (De Filippo and Maricq, Citation2008; Sgro et al., Citation2012). The agglomeration consumes the charged fraction of the core faster than the neutral fraction and the Boltzmann temperature decrease can result from this process. This seems a somewhat plausible explanation, as the electrical enhancement factor (Maricq, Citation2006) at exhaust temperature of 413 K for the agglomeration between 10-nm particles with different electrical polarity is around 5 (1, –1) and the enhancement between the different electrical polarity core (10 nm) and soot (70 nm) is 2 when soot has one opposite charge and above 2 for soot with multiple opposite charge. Sgro et al. (Citation2012) also pointed out that the condensation (surface growth) of the charged core particles lowers the approximated charging temperatures. The surface growth can clearly affect the detected Boltzmann temperature as the residence time in the tail pipe has been shown to increase the size of the nucleation mode (Abdul-Khalek et al., Citation1998b). Nevertheless, also the differences in the effective charging process and the presumptions in the Boltzmann theory used as the basis of the temperature approximation can cause the low temperature results. After all, the particle charge state is formed in a rapidly changing ionization atmosphere where different polarity ions can have different attachment coefficients, while the Boltzmann theory assumes identical molecular ions and steady ionization environment.
The charge state of the core mode clearly shows that the core particles have evolved through a highly ionizing environment before the detection, yet little is known about their composition or formation route.
Effect of lubricant
The idle core is nowadays often considered to form through the inception of lubricating oil metal compounds (e.g., Abdul-Khalek et al., Citation1998a; Lee et al., Citation2006). The idea is based on the fact that the soot sink is low for the ash components at idling load, and thus the metals nucleate rather than deposit on the soot surface. Two different oils were used in this study, and differences were detected for the idle load core between the oils; see .
The mode geometric mean diameter and number concentration from lognormal fits for the core data shown in were 8.7 nm and 8.9 cm−3 for oil 1 and 7.6 nm and 5.2
cm−3 for oil 2, respectively. The difference between the core characteristics between the two oils is hard to interpret because of limited information on the lube composition, the core chemistry, and small differences between the oils. As a possible contributor, there is a difference in the calcium concentration between the oils (see ). The nonvolatile nucleation-mode formation has been suggested to be connected specifically to calcium compounds (Abdul-Khalek et al., Citation1998b). The core number concentration is about 40% higher with oil 1 than with oil 2. The difference is similar for the calcium concentrations between the oils (36% more Ca in oil 1 than in oil 2). The total volume calculated from distribution fit of the core mode was approximately 100% higher with oil 1 than with oil 2, which suggests that at least the surface growth of the core particles depends on some other factors than the Ca concentration. Independent on the specific composition of the oils, we can conclude that a distinctive nonvolatile particle mode was detected at 10 nm size range with both oils at idle-load conditions.
Table 2. Test oil specifications
Particle control methods
As the diesel exhaust particle concentrations are relatively high at idling load conditions and the engines are idling often in densely populated areas such as congestions, the emission control should be considered. Two different strategies are commonly adopted for the diesel engine particle control: tailpipe aftertreatment or fuel injection design or, off course, a combination of them.
Here, the low load particle emission concentrations were studied with a partial filter and a combination of a partial filter and a selective catalytic reduction catalytic converter (SCR), and the effects of the fuel injection parameters were tested by varying fuel injection pressure.
Tailpipe aftertreatment system
In , bottom axis, the wet and dry particle distributions measured with partial filter and partial filter–SCR combination are presented. Different from the raw distributions in , the concentrations of the residue of the wet and dry nucleation mode are the same. The size of the wet nucleation mode is still larger than the core, implying condensational growth of nonvolatile particles. The wet and dry soot-mode concentrations are in this case practically identical; this supports the assumption that the detected difference in the wet and dry soot mode in raw exhaust was due to the overlapping of the soot and the nucleation mode.
Both aftertreatment systems reduced the emitted particle number emissions effectively. The wet total particle number (> 3nm) removal efficiencies were 95% for the partial filter and 96% for the SCR + partial filter combination; see (top). The dry particle total number emission removal efficiencies were 85% and 88% for the partial filter and for SCR + partial filter combination, respectively. From the size dependent removal efficiencies in (top), one can see that the efficiency for soot-mode particle removal is around 0.5 for both aftertreatment systems, and thus the soot emissions exist also with the aftertreatment systems. The aftertreatment efficiency is, on the other hand, well above 90% in the below 10 nm size range.
The increased particle removal efficiency in the SCR comes probably from the low exhaust flow rate and consequent diffusion losses in the monoliths. One should note, again, that there was no urea injection in the SCR. The exhaust volume flow rate at the idling load condition with the current engine is around 138 m3/hr (dry air), transforming to a residence time of about 1.6 sec in a tube with 50 cm length, 40 cm diameter. Thus, there is a possibility for diffusion losses at the core size range.
Effect of engine parameters
The effect of the fuel injection pressure (FIP) on the particle emissions was studied with oil 2. The dry particle distributions were measured at 800 rpm engine speed, with 3 different low-torque conditions (4 N-m, 40 N-m, 100 N-m), with two or three FIP (). A trade-off between the soot and the core was detected for all different loads when the FIP was varied; the increase of the FIP induced, simultaneously, a decrease in the soot mode and an increase in the core concentration. At idle, the increase of the FIP from 35 Mpa to 50 Mpa was followed by a 20% decrease in soot number and a 28% increase in core number. At 40 N-m torque, the FIP change from 35 MPa to 50 MPa induced a 29% decrease in soot and a 12% increase in core number. An FIP increase from 35 MPa to 80 MPa was followed by a 42% decrease in soot and a 23% increase in core number.
Thus, the FIP increase alone does not seem an efficient method to control the total particle number (>3 nm) emissions compared to the presented aftertreatment systems. The tailpipe aftertreatment total particle number efficiencies were above 80%, while the increase of the FIP sometimes increased the total particle number. Even for soot the tailpipe aftertreatment was more efficient than the FIP. Yet the fuel injection design could help the aftertreatment systems to remove the particles from the exhaust as the increase in the particle number emission appeared in the size range where the partial filter efficiency was close to 100% and the decrease in the soot size range where efficiency was close to 50%.
NOx emissions increased simultaneously with the FIP. At idle the increase of the FIP from 35 Mpa to 50 Mpa was followed by a 17% increase in NOx (ppm) concentration, and at 40 N-m torque the NOx (ppm) increase was 27% when FIP increased from 35 MPa to 50 MPa whereas a 60% NOx increase was detected between 35 MPa and 80 MPa FIPs. Thus, the increase of the particle number is followed by the increase in NOx concentration, and therefore the blindfolded FIP increase seems a rather poor pollution control method at low loads compared to the presented tailpipe aftertreatment system.
The simultaneous increase in the core concentration and decrease in the soot concentration with increasing FIP suggest some kind of a dependency between the measured concentrations of the two particle types. The simultaneous change of the particle concentrations can take place through the scavenging of the core particles by the soot in engine or tailpipe, but the dependency can also be caused by the change of the soot sink for metals and molecular hydrocarbon species (D’Alessio et al., Citation2005; Miller et al., Citation2007). These explanations assume that the core and the soot form independently. That is, the soot concentration is controlled by a NOx–soot trade-off, and the independently formed core concentration by soot. However, the core can also be suggested to be a residue of a soot fragmentation, if results shown for two stage flames are followed (Merrill et al., Citation2004; Neoh, Howard, and Sarofim, Citation1985). In this explanation, the core formation is directly dependent on soot fragmentation process. In this hypothesis, the late stages of combustion, just when the flame is quenched, would serve as a lean oxidation conditions favorable for fragmentation, and the soot oxidation would result in residue particle core. Nevertheless, the reason for the dependency remains unknown.
In order to get a wider view on the low load particle emission characteristics, the torque and engine speed were also varied at low load regime. In , dry particle size distributions measured with 50 Mpa FIP at 800 rpm and 1000 rpm engine speeds and a few different torques. The increase of the torque induced an increase in the soot mode and a decrease in the core mode at both engine speeds. The particle emission characteristics were also different between the engine speeds. The increase of the engine speed from 800 rpm to 1000 rpm increased soot-mode concentration around 70% at 40 N-m torque and 130% at 100 N-m. A similar trend has been shown previously for particle mass emissions (Khan et al., Citation2006). At the same time, the core concentration decreased by 10% at 40 N-m and dropped close to zero at 100 N-m.
Figure 6. Dry particle size distributions with 50 MPa injection pressure at 800 rpm engine speed with three different torques (left) and at 1000 rpm engine speed with torques (right). Dynamometer torque is given in legends. The dashed black lines present the lognormal fits. Lubricant oil 2.
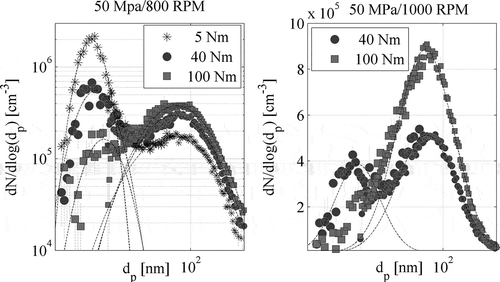
A clear core–soot trade-off was shown here for FIP and engine load variation. A similar trend was shown in our previous study (Lähde et al., Citation2011) at high and medium load. There, however, the core was absent at low load (17%) at all fuel injection pressures even when the soot concentration was low. When combining the results, one must note the effect of the engine speed on the core-soot balance: In this study as well as in the previous study (medium load), the speed decrease induced an increase in the core concentration and a decrease in the soot mode. In the previous study, 17% load was run at 1500 rpm engine speed, and thus the absence of the core mode could result from the combustion process characteristics, dependent on the engine speed.
Summary
Nucleation-mode particles dominated the diesel exhaust particle number emissions at idle load. The nonvolatile nucleation-mode geometric mean diameter was at 10 nm or below. The core charge state implied that the core has evolved through a highly ionizing environment before emission from the engine. The determined charging probabilities were 10 2.2% for negative and 8.0
2.0% for positive polarity. The detected Boltzmann temperatures were for negative polarity 875 K and for positive polarity 745 K. The slightly biased charge state suggests that the core charge state is not equilibrated before the measurement. The core concentration and size were also shown to be dependent on the lubricant oil composition; a connection between the lubricant Ca concentration and the core concentration and size was also suggested. Nevertheless, the dependency between the lubricant and core needs still to be verified.
The core number emissions were also shown to be efficiently controlled with a partial filter or with a partial filter + SCR combination. The aftertreatment systems’ particle number removal efficiencies were higher than 95% for the wet total particle number and higher than 85% for the dry total particle number. Nevertheless, the aftertreatment systems efficiencies were around 50% for the soot-mode particles.
The low-load core emissions were also shown be dependent on the engine load, speed, and fuel injection pressure. The simple fuel injection pressure increase alone seems, however, a rather poor pollution control method as the NOx emissions and total particle number (>3 nm) increased with increasing fuel injection pressure. The low-load particle number emissions followed the soot-core trade-off similar to medium or high operating loads.
The presented results enlighten the particle emission characteristics and control at idle and low-load conditions. The results support the development of the particle control methods and exposure evaluation.
Additional information
Notes on contributors
Tero Lähde
Tero Lähde is a patent examiner at Finnish Patent and Registration Office, Helsinki, Finland.
Annele Virtanen
Annele Virtanen is an associate professor at the University of Eastern Finland, Kuopio, Finland.
Matti Happonen
Matti Happonen is a research engineer at AGCO Power, Inc., Linnavuori, Finland.
Christer Söderström
Christer Söderström works as a research scientist and Matti Kytö is a principal scientist at VTT Technical Research Centre of Finland, VTT, Finland.
Matti Kytö
Christer Söderström works as a research scientist and Matti Kytö is a principal scientist at VTT Technical Research Centre of Finland, VTT, Finland.
Jorma Keskinen
Jorma Keskinen is a professor in the Department of Physics at Tampere University of Technology, Tampere, Finland.
References
- Abdul-Khalek, I. S., D. B. Kittelson, B. R. Graskow, Q. Wei, and F. Brear. 1998a. Diesel exhaust particle size: Measurement issues and trends. SAE Technical Paper Series 980525.
- Abdul-Khalek, I., D. Kittelson, and F. Brear. 1998b. Diesel trap performance: Particle size measurements and trends. SAE Technical Paper Series 982599.
- Bielaczyc, P., J. Keskinen, J. Dzida, R. Sala, T. Ronkko, T. Kinnunen, P. Matilainen, P. Karjalainen, and M. Happonen. 2012. Performance of particle oxidation catalyst and particle formation studies with sulphur containing fuels. SAE Int. J. Fuels Lubricants 5:611–19. doi:10.4271/2012-01-0366
- Burtscher, H., U. Baltensperger, N. Bukowiecki, P. Cohn, C. Häglin, M. Mohr, U. Matter, S. Nyeki, V. Schmatloch, N. Streit, and E. Weingartner. 2001. Separation of volatile and non-volatile aerosol fractions by thermodesorption: instrumental development and applications. Journal of Aerosol Science 32(4):427–442.
- Lehtoranta, K., P. Matilainen, T.-J. J. Kinnunen, J. Heikkilä, T. Rönkkö, J. Keskinen, T. Murtonen. 2009. Diesel particle emission reduction by a particle oxidation catalyst. SAE Technical Paper 2009-01-2705. doi:10.4271/2009-01-2705
- Brodrick, C.-J., H. A. Dwyer, M. Farshchi, D. B. Harris, and F. G. King. 2002. Effects of engine speed and accessory load on idling emissions from heavy-duty diesel truck engines. J. Air Waste Manage. Assoc. 52(9): 1026–31. doi:10.1080/10473289.2002.10470838
- Chen, D. R., D. Y. H. Pui, D. Hummes, H. Fissan, F. R. Quant, and G. J. Sem. 1998. Design and evaluation of a nanometer aerosol differential mobility analyzer (nano-dma). Journal of Aerosol Science 29(5–6):497–509.
- D’Alessio, A., A. C. Barone, R. Cau, A. D’Anna, and P. Minutolo. 2005. Surface deposition and coagulation efficiency of combustion generated nanoparticles in the size range from 1 to 10 nm. Proc. Combustion Inst. 30(2): 2595–603. doi:10.1016/j.proci.2004.08.267
- De Filippo, A., and M. M. Maricq. 2008. Diesel nucleation mode particles: Semivolatile or solid? Environ. Sci. Technol. 42(21): 7957–62. doi:10.1021/es8010332
- Fuchs, N. A. 1963. On the stationary charge distribution on aerosol particles in a bipolar ionic atmosphere. Pure Appl. Geophys. 56(1): 185–93. doi:10.1007/BF01993343
- Heikkilä, J., T. Rönkkö, T. Lähde, M. Lemmetty, A. Arffman, A. Virtanen, J. Keskinen, L. Pirjola, and D. Rothe. 2009. Effect of open channel filter on particle emissions of modern diesel engine. J. Air Waste Manage. Assoc. 59:1148–54. doi:10.3155/1047-3289.59.10.1148
- Heywood, J. B. 1989. Internal Combustion Engine Fundamentals. New York, NY: McGraw-Hill.
- Hoppel, W. A. and G. M. Frick. 1986. Ion-aerosol attachment coefficients and the steady-state charge distribution on aerosols in a bipolar environment. Aerosol Sci. Technol. 5(1): 1–21. doi:10.1080/02786828608959073
- Keskinen, J., K. Pietarinen, M. Lehtimäki. 1992. Electrical low pressure impacto. Journal of Aerosol Science 23: 353–360.
- Khan, A. B. M. S., N. N. Clark, G. J. Thompson, W. S. Wayne, M. Gautam, D. W. Lyon, and D. Hawelti. 2006. Idle emissions from heavy-duty diesel vehicles: Review and recent data. J. Air Waste Manage. Assoc. 56(10): 1404–19. doi:10.1080/10473289.2006.10464551
- Kinsey, J. S., D. C. Williams, Y. Dong, and R. Logan. 2007. Characterization of fine particle and gaseous emissions during school bus idling. Environ. Sci. Technol. 41(14): 4972–79. doi:10.1021/es0625024
- Kittelson, D. B., W. F. Watts, and J. P. Johnson. 2006. On-road and laboratory evaluation of combustion aerosols, Part 1: Summary of diesel engine results. J. Aerosol Sci. 37(8): 913–30. doi:10.1016/j.jaerosci.2005.08.005
- Lähde, T., T. Rönkkö, M. Happonen, C. Söderström, A. Virtanen, A. Solla, M. Kytö, D. Rothe, and J. Keskinen. 2011. Effect of fuel injection pressure on a heavy-duty diesel engine nonvolatile particle emission. Environ. Sci. Technol. 45(6): 2504–09. doi:10.1021/es103431p
- Lee, D., A. Miller, K. Park, and M. R. Zachariah. 2006. Effects of trace metals on particulate matter formation in a diesel engine: Metal contents from ferrocene and lube oil. Int. J. Automotive Technol. 7(6): 667–73.
- Lehtoranta, K., P. Matilainen, T-J. J. Kinnunen J., Heikkilä, T. Rönkkö, J. Keskinen, T. Murtonen. 2009. Diesel particle emission reduction by a particle oxidation catalyst. SAE Technical Paper 2009-01-2705.
- Lemmetty, M., L. Pirjola, J. M. Mäkelä, T. Rönkkö, and J. Keskinen. 2006. Computation of maximum rate of water-sulphuric acid nucleation in diesel exhaust. J. Aerosol Sci. 37(11): 1596–604. doi:10.1016/j.jaerosci.2006.04.003
- Li, X. L., Z. Huang, J.-S. Wang, and J.-H. Wu. 2008. Characteristics of ultrafine particles emitted from a dimethyl ether (DME) engine. Chin. Sci. Bull. 53(2): 304–12. doi:10.1007/s11434-008-0011-4
- Lindgren, M., K. Arrhenius, G. Larsson, L. Bäfver, H. Arvidsson, C. Wetterberg, P.-A. Hansson, and. Rosell. 2011. Analysis of unregulated emissions from an off-road diesel engine during realistic work operations. Atmos. Environ. 45 (30): 5394–98. doi:10.1016/j.atmosenv.2011.06.046
- Maricq, M. M. 2006. On the electrical charge of motor vehicle exhaust particles. J. Aerosol Sci. 37(7): 858–74. doi:10.1016/j.jaerosci.2005.08.003
- Merritt, P. M., V. Ulmet, R. L. McCormick, W. E. Mitchell, and K. J. Baumgard. 2005. Regulated and unregulated exhaust emissions comparison for three Tier II non-road diesel engines operating on ethano–diesel blends. SAE Technical Paper Series 2005-01-2193. doi:10.4271/2005-01-2193
- Mikkanen, P., M. Moisio, J. Keskinen, J. Ristimäki, and M. Marjamäki. 2001. Sampling method for particle measurements of vehicle exhaust. SAE Trans. 2001-01-0219. doi:10.4271/2001-01-0219
- Merrill, C. J., J. S. Lighty, E. G. Eddings, and A. F. Sarofim. 2004. Investigation of the oxidation and fragmentation of soot in a two-stage flat flame burner system utilizing a scanning mobility particle sizer. Abstract. Western States Section of the Combustion Institute Meeting 04S-40.
- Miller, A., G. Ahlstrand, D. Kittelson, and M. Zachariah. 2007. The fate of metal (Fe) during diesel combustion: Morphology, chemistry, and formation pathways of nanoparticles. Combust. Flame 149(1–2): 129–43. doi:10.1016/j.combustflame.2006.12.005
- Neoh, K. G., J. B. Howard, and A. F. Sarofim. 1985. Effect of oxidation on the physical structure of soot. Symp. (Int.) Combust. 20(1): 951–57. doi:10.1016/S0082-0784(85)80584-1
- Park, D., Y. Yoon, S.-B. Kwon, W. Jeong, Y. Cho, and K. Lee. 2012. The effects of operating conditions on particulate matter exhaust from diesel locomotive engines. Sci. Total Environ. 419(0): 76–80. doi:10.1016/j.scitotenv.2012.01.002
- Rasdorf, W., C. Frey, P. Lewis, K. Kim, S. Pang, and S. Abolhassani. 2010. Field procedures for real-world measurements of emissions from diesel construction vehicles. J. Infrastruct. Systems 16(3): 216–25. doi:10.1061/(ASCE)IS.1943-555X.0000027
- Reischl, G. P. J. M. Mäkelä, R. Karch, J. Necid. 1996. Bipolar charging of ultrafine particles in the size range below 10 nm. Journal of Aerosol Science 27: 931–949.
- Rönkkö, T., A. Virtanen, J. Kannosto, J. Keskinen, M. Lappi, and L. Pirjola. 2007. Nucleation mode particles with a nonvolatile core in the exhaust of a heavy-duty diesel vehicle. Environ. Sci. Technol. 41(18): 6384–89. doi:10.1021/es0705339
- Sgro, L. A., A. D’Anna, and P. Minutolo. 2011. Charge fraction distribution of nucleation mode particles: New insight on the particle formation mechanism. Combust. Flame 158(7): 1418–25. doi:10.1016/j.combustflame.2010.r11.010
- Sgro, L. A., P. Sementa, B. M. Vaglieco, G. Rusciano, A. D’Anna, and P. Minutolo. 2012. Investigating the origin of nuclei particles in GDI engine exhausts. Combust. Flame 159(4): 1687–92. doi:10.1016/j.combustflame.2011.12.013
- Storey, J. M. E., J. E. Parks, S. A. Lewis, W. K. Kahl, R. L. Miller, and W. T. Davis. 2006. Characterization of heavy-duty truck air quality impacts by ambient air monitoring at the Watt Road Environmental Laboratory. Oak Ridge, TN: Oak Ridge National Laboratory.
- Tang, U. W., and Z. Wang. 2006. Determining gaseous emission factors and driver’s particle exposures during traffic congestion by vehicle-following measurement techniques. J. Air Waste Manage. Assoc. 56(11): 1532–39. doi:10.1080/10473289.2006.10464567
- Tong, H. Y., W. T. Hung, and C. S. Cheung. 2000. On-road motor vehicle emissions and fuel consumption in urban driving conditions. J. Air Waste Manage. Assoc. 50(4): 543–54. doi:10.1080/10473289.2000.10464041
- Vaaraslahti, K., A. Virtanen, J. Ristimaki, and J. Keskinen. 2004. Nucleation mode formation in heavy-duty diesel exhaust with and without a particulate filter. Environ. Science & Technol. 38(18): 4884–90. doi:10.1021/es0353255
- Vakkilainen, A., and R. Lylykangas. 2004. Particle oxidation catalyst (POC) for diesel vehicles. SAE Technical Paper Series 2004-28-0047. doi:10.4271/2004-28-0047
- Wang, S. C., and R. C. Flagan. 1989. Scanning electrical mobility spectrometer. J. Aerosol Sci. 20(8): 1485–88. doi:10.1016/0021-8502(89)90868-9
- Weijers, E. P., A. Y. Khlystov, G. P. A. Kos, and J. W. Erisman. 2004. Variability of particulate matter concentrations along roads and motorways determined by a moving measurement unit. Atmos. Environ. 38(19): 2993–3002. doi:10.1016/j.atmosenv.2004.02.045
- Wheatley, A. D., and S. Sadhra. 2004. Occupational exposure to diesel exhaust fumes. Ann. Occup. Hyg. 48(4): 369–76. doi:10.1093/annhyg/meh018