Abstract
The purpose of this study is to quantify the thermal conductivity of sewage sludge related to reaction temperature for the optimal design of a thermal hydrolysis reactor. We continuously quantified the thermal conductivity of dewatered sludge related to the reaction temperature. As the reaction temperature increased, the dewatered sludge is thermally liquefied under high temperature and pressure by the thermal hydrolysis reaction. Therefore, the bound water in the sludge cells comes out as free water, which changes the dewatered sludge from a solid phase to slurry in a liquid phase. As a result, the thermal conductivity of the sludge was more than 2.64 times lower than that of the water at 20℃. However, above 200℃, it became 0.704 W/m•°C, which is about 4% higher than that of water. As a result, the change in physical properties due to thermal hydrolysis appears to be an important factor for heat transfer efficiency.
Implications
The thermal conductivity of dewatered sludge is an important factor the optimal design of a thermal hydrolysis reactor. The dewatered sludge is thermally liquefied under high temperature and pressure by the thermal hydrolysis reaction. The liquid phase slurry has a higher thermal conductivity than pure water.
Introduction
Sewage sludge generated from sewage treatment plants is increasing every year. Technology to treat the sludge safely in the land is required because ocean dumping of sewage sludge is prohibited. The treatment of sewage sludge can be classified into two parts: recycling of sewage sludge and reduction of sewage sludge.
Recycling technology includes fuel and soil covering, aggregate, and carbonization. Sludge reduction technology includes different physicochemical technologies (Qdegaard, Citation2004; Kelly, Citation2006) and biological treatment (Barr et al., Citation2008). However, to reduce the moisture content by 40% for recycling sewage sludge, considerable energy is consumed and cost-effective treatment is required. Many technologies using ozone, supersonic waves, microwaves, and thermal hydrolysis have been applied to reduce the moisture content after breaking the sludge cells in order to reduce the energy.
Sewage sludge has the potential to be utilized as a valuable resource rather than be discarded as a waste. Sewage sludge is rich in organic carbon, and so it may be utilized as a cheap but valuable resource for the production of volatile fatty acids (VFAs). It is widely applied as a means to convert organic waste into substrates for many bioprocesses of nutrient removal of wastewater (Bougrier et al., Citation2007, Citation2008; Kampas et al., Citation2007) and biopolymer production (Chua et al., Citation2003). It has also been used to increase methane production by increasing digestion efficiency in an anaerobic digestion tank (Bougrier et al., Citation2006; Keymer et al., Citation2013).
In this study, we used a thermal hydrolysis reaction (Neyens and Baeyens, Citation2003; Shana et al., Citation2013; Morgan-Sagastume et al., Citation2011) to transfer the bound water inside cells to free water by applying a heat-chemical method to break into the sludge cell without evaporating the moisture in the cell.
Mechanically dewatered sewage sludge of solid state contains about 80% moisture, including free water and bound water. Therefore, a heat source should be supplied to the sludge under optimum conditions from the outside in the proposed heat-chemical reaction method. The sludge temperature increases slowly because the heat is transferred by conduction in the case of solid sewage sludge. To increase the temperature faster, a higher outside temperature is required, resulting in carbonization of the sludge due to high temperature. Therefore, the thermal hydrolysis reaction tank and optimal heat source should be designed efficiently, and the heat source should be increased related to the reaction temperature as the bound water is transformed into free water (Neyens and Baeyens, Citation2003; Bougrier et al., Citation2008).
Heat transfer characteristics of dewatered sludge were determined to provide basic data for the optimal design of a reaction tank using apparatus that was custom made for this study. Our measuring system was calibrated using standard water. The heat transfer was measured continuously related to the sludge temperature difference to obtain the value of thermal conductivity. The thermal conductivity function was determined related to the reaction temperature for the optimal design of a thermal hydrolysis reactor.
Experimental
Experimental setup
Our thermal conductivity measuring apparatus is shown in . The apparatus was designed and built to determine the heat transfer characteristics of sewage sludge at high temperatures and high pressures related to the reaction temperature. The apparatus includes a reaction tank, a heat supply, and measurement and control components. The reaction tank was manufactured using stainless steel to resist a temperature of 250 °C and high pressure of 50 bar. A 3-L Teflon vessel was installed in the tank to minimize the heat loss, and to give an adiabatic condition.
Two circular heat convection baffles were installed between thermocouples to decrease heat convention during the thermal hydrolysis reaction of the dewatered sludge. The heat supply consists of an electric heater surrounded by a heating block. The heater was installed in the center of reactor to transfer the heat linearly, in the radial direction of the sludge. A ceramic ball was installed at the upper and lower heating block to minimize heat loss due to the heat transfer.
We measured the temperature and pressure continuously. Six K-type thermocouples were installed to measure the sludge temperature in the reactor (one [T_H] for the center of the reactor and five for the radial directions from the center), as shown in . Five thermocouples were installed in the radial direction: two (T1-1 and T1-2) 40 mm from the center, one (T2) 70 mm from the center, and the other two (T3-1 and T3-2) 100 mm from the center. Two pairs of thermocouples, located at 40 mm and 100 mm, were installed to determine whether heat is transferred linearly in the radial directions.
Calculation of thermal conductivity
The principle of measuring thermal conductivity as the sewage sludge temperature increases is based on Fourier’s law (Fourier, Citation1955; Incropera and Dewitt, Citation1996) as follows. Specific heat flow between two materials with area A (m2), distance Δx (m), and temperature difference ΔT (°C) is given by the following equation when heat ΔQ (W·sec) is transferred during Δt (sec):
Experimental methods
In this study, sewage sludge from the sewage treatment plant in Gwangju, Republic of Korea, was dewatered using a centrifugal dryer. The physicochemical characteristics of sewage sludge are summarized in . A total 1.8 kg of sewage sludge was filled evenly in the 3-L teflon vessel to measure the thermal conductivity. The sludge hydrolysis reaction occurred with a temperature increase, and the sludge was changed from the solid phase to the liquid phase. During this period, heat transfer occurred by conduction and convection simultaneously. To resist heat transfer due to convection, a ring-type convection baffles of yellow copper with an inner diameter of 110 mm, an outer diameter of 180 mm, a height of 50 mm, and a width of 0.5 mm was installed, as shown in .
Table 1. Physicochemical characteristics of sewage sludge
A 380-W electric heater supplied heat continuously into the sludge layer to increase its temperature. To solve the problem of carbonization when dewatered sludge contacts the heating block due to the abrupt temperature increase of the heating block and low thermal conduction, power was controlled automatically to disconnect when reaching a heating block temperature of 230 °C, and to reconnect at a temperature of 220 °C. The same energy put into the standard material (water) was put into the sludge. The temperature, pressure, and input power of the reactor were monitored at 1-min intervals. Experimental reference conditions were found through pretests. These conditions were as follows: reaction time 6 hr, final pressure 18 bar in 3-L Teflon vessel. At this time, the final pressure is higher than vapor pressure of 16 bar due to the volatile gases at a temperature of 200 °C.
During hydrolysis, thermal conductivity was calculated based on the temperature difference between the center and radial position of the reactor. The temperature difference (ΔT) between the center of the heating block and the special point in the radial direction was used. The thermal conductivity measuring apparatus was calibrated by measuring the temperature difference of water as the standard material (Kirillov, Citation2006; Ramires et al., Citation1995) between the center point and radial directional locations, related to reaction temperature. By applying the same method used with the water to the sludge, the thermal conductivity of sewage sludge was determined using eq 3.
Results and Discussion
Physical properties of organic waste due to thermal hydrolysis reaction
shows the change in physical properties due to the thermal hydrolysis reaction temperature (Han et al., Citation2012). As shown in the figure, physical properties were changed related to the reaction temperature when dewatered sewage sludge was placed in the reactor and heat was supplied from the outside. This change was obtained due to the fact that the inner temperature and pressure of the closed reactor were increased by the vapor pressure as the reaction temperature increased. The bound water was transformed to free water and came out as the cell wall of the dewatered sludge was destroyed. The physical property of the sewage sludge was changed into an almost-liquid-phase slurry when the reaction temperature of sludge was increased to above 190 °C.
Heat transfer characteristics of water and sludge
shows the temperature difference profiles of water and dewatered sludge related to reaction temperature. The figure shows the heat transfer characteristics of water and sewage sludge using the water conductivity as the standard material. The reaction temperature difference between the center and each position to the radial directions T1, T2, and T3 was monitored continuously. shows the temperature difference of the water related to radial position as the reaction temperature increased. The temperature differences (ΔT) between the center of the heating block and radial positions (T1, T2, and T3) were monitored as the reaction temperature increased. As the reaction temperature increased, the temperature difference between the center and each position decreased because the heat transfer efficiency increased due to the activated energy of water molecule as the reaction temperature increased. The temperature differences were not the same related to radial positions (thermocouples T1, T2, and T3) because the heat was transferred partly by convection.
Figure 3. Temperature difference profiles of water and dewatered sludge versus reaction temperature: (a) water; (b) dewatered sludge.
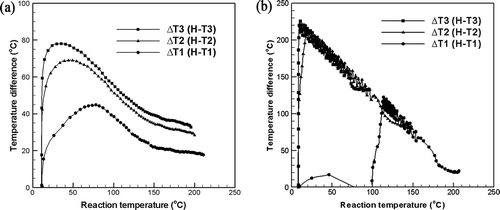
shows the heat transfer characteristics of the sewage sludge measured by the same method as used for the water. Unlike the water, the temperature difference between the center and radial direction position in the sludge was large (over 200 °C) because the heat transfer from the center of the heating block in the radial direction was small. However, as the reaction temperature increased, the bound water of the sludge cell wall was transformed to free water due to the thermal hydrolysis reaction 9; Han et al., Citation2012). Therefore, as this free water filled the void in the solid sludge and increased the heat conduction of the sludge, the temperature difference between the center and radial position decreased abruptly as the reaction temperature increased. The temperature difference between the center and radial direction position decreased linearly versus the reaction temperature because the heat transfer was accomplished mainly by conduction, with little influence from natural convection.
Comparison of heat transfer between the water and sludge
shows the temperature difference between the center and radial direction position, and the required power related to the reaction temperature, for the water and sludge. shows the temperature differences between the central heating block (H) and three radial direction positions (T1, T2, and T3) as a linear relationship. The reaction temperature for the water and sludge increased with time. The reaction temperature was divided into three sections to obtain a linear function of the temperature differences with high correlation (R). The reaction temperature was divided into three ranges to obtain the linear function of the temperature differences between the central heating block (H) and three radial direction positions (T1, T2, and T3): from ambient to 60 °C, from 60 to 150 °C, and from 150 to 210 °C. The temperature differences (H-T3) between the central heating block (H) and the radial direction 100 mm position (T3) were applied for the range from ambient to 60 °C. The temperature differences (H-T2) between the central heating block (H) and the radial direction 70 mm position (T2) were applied for the range from 60 to 150 °C. The temperature differences (H-T1) between the central heating block (H) and the radial direction 40 mm position (T1) were applied for the range from 150 to 210 °C.
Figure 4. Comparison of the temperature differences (ΔT) of water and dewatered sludge: (a) temperature difference (°C); (b) integrated electric power (kWhr).
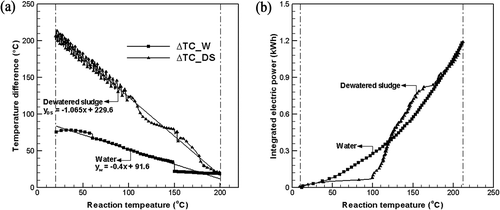
As shown in , at the initial stage, the temperature difference (H-T3) between the center and the most remote position in the radial direction for the sewage sludge was 208.3 °C, representing that a heat transfer of sewage sludge was 2.64 times lower than that of the water having the temperature difference of 78.9 °C. However, as the reaction temperature increased, heat transfer efficiency increased due to the thermal hydrolysis reaction. For a reaction temperature above 190 °C, the heat transfer efficiency of the dewatered sewage sludge was almost the same as that of the water (see ). The linear equations for the temperature difference with the reaction temperature are as follows:
shows the input power for the water and dewatered sludge. The final cumulative power was the same for the two materials. Although the input power increased linearly as the reaction temperature increased for the water, the input power increased very slowly until a reaction temperature of 100 °C was reached, and increased abruptly above 100 °C for the dewatered sludge. The dewatered sludge exhibited the solid phase as shown in , and the heat transfer rate was very low, resulting in low input power. Therefore, the change in the physical property is shown as the important factor for heat transfer efficiency.
Comparison of thermal conductivities between water and sludge
shows the thermal conductivities of water and dewatered sludge as the reaction temperature increases. In , thermal conductivity of water is the reported value (Kirillov, Citation2006; Ramires et al., Citation1995), and the thermal conductivity of sludge was determined using eq 3 with the temperature difference value from . Heat transfer was accomplished by conduction (not by convection) because a convection resister plate was installed in the reaction container.
The thermal conductivity of the water increased for a reaction temperature below 100 °C (Ramires et al., Citation1995), and maintained a constant value at the reaction temperature from 100 to 150 °C while decreasing at temperatures above 150 °C. Although the thermal conductivity of the dewatered sludge showed a low value of 0.230 W/m·°C at the initial stage, as shown in , the thermal conductivity increased rapidly from 130 °C as the bound water changed into free water. The thermal conductivity of sludge was 0.704 W/m·°C, which was 4% higher than that of the water when the dewatered sludge changed into liquid slurry as shown in , due to the thermal hydrolysis reaction above 200 °C.
The liquid-phase slurry had a higher thermal conductivity than pure water because it contained more than 15% of solid particles, which have higher heat transfer efficiency.
Thermal conductivity function of sludge
shows the thermal conductivities measured and estimated by the numerical equation for the optimal design of a thermal hydrolysis reactor. The reaction temperature was divided into three areas to obtain a consistent equation with a measured value. The consistency of the calculated function was in the range from 86.4% to 103.7%, representing an average value of 99%. The derived functions are given by eqs 6–8:
Conclusion
The thermal conductivity of dewatered sludge is an important factor for the optimal design of a thermal hydrolysis reactor. The conductivity related to reaction temperature was determined after measuring heat transfer continuously in a pilot reactor. A thermal conductivity function was derived to obtain boundary conditions of numerical simulation for the optimal design of a thermal hydrolysis reactor. Based on our results, our conclusions are as follows:
As the free water was transformed from the bound water of the dewatered sludge cell and came out to fill the void inside the solid sludge, the heat transfer efficiency increased. At the initial stage, the temperature difference (H-T3) between the center and the most remote place in the radial direction for the sewage sludge was 208.3 °C, which represents that heat transfer efficiency is 2.64 times lower for sewage sludge compared with water having a temperature difference of 78.9 °C. However, as the reaction temperature increased, the heat transfer efficiency was increased by the thermal hydrolysis reaction. For reaction temperatures above 190 °C, the heat transfer efficiency of dewatered sewage sludge was almost the same as that of water (see ).
Although input power increased linearly as the reaction temperature increased for the water, the input power increased very slowly until reaching a reaction temperature of 100 °C, and increased abruptly above 100 °C for the dewatered sludge. The change in physical properties due to thermal hydrolysis appears to be an important factor for heat transfer efficiency.
The thermal conductivity of the dewatered sludge was low of 0.230 W/m·°C at the initial stage of the reaction. The value increased to 0.704 W/m·°C, which was 4% higher than that of water when the dewatered sludge changed into liquid-state slurry at a reaction temperature above 200 °C. This is due to the fact that solid particles of sludge increase the heat transfer more than the water.
The thermal conductivity function related to reaction temperature was derived to give the boundary condition for the optimal design of the thermal hydrolysis reactor. The consistency of the calculated function was in the range of 86.4–103.7%, representing an average value of 99%.
Funding
This research was carried out with the financial resources of the Ministry of Agriculture, Food and Rural Affairs, and with the support of Korea Institute & Evaluation for Technology in Food, Agriculture, Forestry & Fisheries. The authors appreciate their valuable support.
Additional information
Notes on contributors
Hyoung Woon Song
Hyoung-Woon Song is a principal researcher, and Seong Kuk Han and Hee Suk Jung are senior researchers at Institute for Advanced Engineering, Yongin, Republic of Korea.
Keum Joo Park
Keum Joo Park is a professor at Sunchon National University.
Seong Kuk Han
Hyoung-Woon Song is a principal researcher, and Seong Kuk Han and Hee Suk Jung are senior researchers at Institute for Advanced Engineering, Yongin, Republic of Korea.
Hee Suk Jung
Hyoung-Woon Song is a principal researcher, and Seong Kuk Han and Hee Suk Jung are senior researchers at Institute for Advanced Engineering, Yongin, Republic of Korea.
References
- Barr, K.G., D.O. Solley, D.J. Starrenburg, and R.G. Lewis. 2008. Evaluation, selection and initial performance of a large scale centralised biosolids facility at Oxley Creek water reclamation plant. Water Sci. Technol. 57:1579–1586. doi:10.2166/wst.2008.041
- Bougrier, C., J.P. Delgenes, and H. Carrere. 2006. Combination of thermal treatments and anaerobic digestion to reduce sewasge sludge quantity and improve biogas yield. Process Saf. Environ. Protect. 84(B4):280–284. doi:10.1205/psep.05162
- Bougrier, C., J.P. Delgenes, and H. Carrere. 2007. Impacts of thermal pre-treatments on the semi-continuous anaerobic digestion of waste activated sludge. Biochem. Eng. J. 34:20–27. doi:10.1016/j.bej.2006.11.013
- Bougrier, C., J.P. Delgenes, and H. Carrere. 2008. Effects of thermal treatments on five different waste activated sludge samples solubilisation: Physical properties and anaerobic digestion. Chem. Eng. J. 139:236–244. doi:10.1016/j.cej.2007.07.099
- Chua, A.S.M., H. Takabatake, H. Satoh, and T. Mino. 2003. Production of polyhydroxyalkanoates (PHA) by activated sludge treating municipal wastewater: Effect of pH, sludge retention time (SRT), and acetate concentration in the influent. Water Resour. 37:3602–3611. doi:10.1016/S0043-1354(03)00252-5
- Fourier. J. 1955. The Analytical Theory of Heat. New York: Dover Publications.
- Han, S.K., H.W. Song, C.S. Choi, H. Kim, and S.E. Lee. 2012. Physicochemical properties of sewage sludge related to thermal hydrolysis reaction temperature. J. Korea Soc. Waste Manage. 29:414–420.
- Incropera, F.P., and D.P. Dewitt. 1996. Fundamentals of Heat and Mass Transfer. Hoboken, NJ: John Wiley & Sons.
- Kampas, P., S.A. Parsons, P. Pearce, S. Ledoux, P. Vale, J. Churchley, and E. Cartmell. 2007. Mechanical sludge disintegration for the production of carbon source for biological nutrient removal. Water Resour. 41: 1734–1742. doi:10.1016/j.watres.2006.12.044
- Kelly, H.D. 2006. Emerging processes in biosolids treatment. J. Environ. Eng. Sci. 5:175–186. doi:10.1139/s05-025
- Keymer, P., I. Ruffell, S. Pratt, and P. Lant. 2013. High pressure thermal hydrolysis as pre-treatment to increase the methane yield during anaerobic digestion of microalgae. Bioresour. Technol. 131:128–133. doi:10.1016/j.biortech.2012.12.125
- Kirillov, P.L. 2006. Thermophysical Properties of Materials for Nuclear Engineering. Obninsk, Russia: Institute for Heat and Mass Transfer in Nuclear Power Plants.
- Morgan-Sagastume, F., S. Pratt, A. Karlsson, D. Cirne, P. Lant, and A. Werker. 2011. Production of volatile fatty acids by fermentation of waste activated sludge pre-treated in full-scale thermal hydrolysis plants. Bioresour. Technol. 102:3089–2097. doi:10.1016/j.biortech.2010.10.054
- Neyens, E., and J. Baeyens. 2003. A review of thermal sludge pre-treatment processes to improve dewaterability. J. Hazard. Mater. 98(B):51–67. doi:10.1016/S0304-3894(02)00320-5
- Qdegaard, H. 2004. Sludge minimization technologies—An overview. Water Sci. Technol. 49:31–40.
- Ramires, M.L.V., C.A. Nieto De Castro, Y. Nagasaka, A. Nagashima, M.J. Assael, and W.A. Wakeham. 1995. Standard Reference Data for the Thermal Conductivity of Water, 1377–1381. Zurich: IUPAC.
- Shana, A., S. Ouki, A. Asaadi, P. Pearce, and G. Mancini. 2013. The impact of intermediate thermal hydrolysis on the degradation kinetics of carbohydrates in sewage sludge. Bioresour. Technol. 137:239–244. doi:10.1016/j.biortech.2013.03.121