Abstract
Total suspended particulate (TSP) samples were seasonally collected at the air exhaust of 15 commercial concentrated animal feeding operations (CAFOs; including swine finishing, swine farrowing, swine gestation, laying hen, and tom turkey) in the U.S. Midwest. The measured TSP concentrations ranged from 0.38 ± 0.04 mg m−3 (swine gestation in summer) to 10.9 ± 3.9 mg m−3 (tom turkey in winter) and were significantly affected by animal species, housing facility type, feeder type (dry or wet), and season. The average particle size of collected TSP samples in terms of mass median equivalent spherical diameter ranged from 14.8 ± 0.5 µm (swine finishing in winter) to 30.5 ± 2.0 µm (tom turkey in summer) and showed a significant seasonal effect. This finding affirmed that particulate matter (PM) released from CAFOs contains a significant portion of large particles. The measured particle size distribution (PSD) and the density of deposited particles (on average 1.65 ± 0.13 g cm−3) were used to estimate the mass fractions of PM10 and PM2.5 (PM ≤10 and ≤2.5 μm, respectively) in the collected TSP. The results showed that the PM10 fractions ranged from 12.7 ± 5.1% (tom turkey) to 21.1 ± 3.2% (swine finishing), whereas the PM2.5 fractions ranged from 3.4 ± 1.9% (tom turkey) to 5.7 ± 3.2% (swine finishing) and were smaller than 9.0% at all visited CAFOs. This study applied a filter-based method for PSD measurement and deposited particles as a surrogate to estimate the TSP’s particle density. The limitations, along with the assumptions adopted during the calculation of PM mass fractions, must be recognized when comparing the findings to other studies.
Implications: The concentration, size, and density of TSP samples varied greatly with animal species, housing facility type, feeder type, and season, suggesting that PM emission data derived from limited measurements may not be readily applied to estimate the overall emission from concentrated animal feeding operations (CAFOs). This study also affirmed that particles released from CAFOs is of relatively high density (~1.65 g cm−3) and with diameter mostly larger than 10 µm, indicating that regular PM abatement devices, such as cyclones, fabric filters, or even a simple downward-facing exhaust duct, may be employed to mitigate the TSP emission with acceptable efficiency.
Introduction
Animal production in the United States has undergone a rapid consolidation process towards fewer but larger feeding operations (Wing and Wolf, Citation2000). Today, a single manure-belt laying hen building can house over a half million birds. This intensive animal production results in usually poor indoor air quality and the release of aerial pollutants, including NH3, H2S, and particulate matter (PM), into the ambient air (Wathes et al., Citation1998; Clantonet al., Citation1999; Bottcher et al., Citation2004). PM released from concentrated animal feeding operations (CAFOs) may act as a carrier of odorants (Lee and Zhang, Citation2008; Yang et al., Citation2014), toxins (e.g., mycotoxin and endotoxin) (Douwes et al., Citation2003; Yang et al., Citation2013), allergens, and microorganisms (e.g., bacteria and fungi) (Hong et al., Citation2012), raising health risks for neighboring residents. PM from CAFOs could also adversely affect the environment, e.g., causing haze (visibility degradation) in rural regions (Hiranuma et al., Citation2008). Potential future emission regulations are being considered by the U.S. Environmental Protection Agency (EPA).
In general, PM emission from a CAFO can be mitigated by (1) adding additives (e.g., lipids) to animal feeds to suppress the aerosolization of feed and litter particles; (2) spraying oil or water droplets in the room air to settle suspended PM; and (3) implementing air abatement devices (e.g., wet scrubber, biofilter, electrostatic precipitator, and cyclone) to remove PM from the exhaust gas (Tan and Zhang, Citation2004). PM concentration, size, and density of the exhaust air from typical CAFOs are essential parameters for the design and operation of most abatement devices (Zhang, Citation2005). However, such relevant information has been, to date, sporadic in the literature.
Several challenges are associated with measuring the size and concentration of PM from CAFOs. One of the best-known is that PM produced at CAFOs has considerably larger particle size (up to 200 µm) than ambient aerosols (Maghirang and Puma, Citation1997; Sweeten et al., Citation1998; Predicala et al., Citation2001; Redwine et al., Citation2002). Most aerosol particle size analyzers were designed for monitoring the particle size distribution (PSD) of ambient aerosols with a diameter typically smaller than 20 µm (McMurry, Citation2000; Baron et al., Citation2011). Their maximum detectable particle size is usually smaller than the largest PM found at CAFOs. Only a few analyzers are capable of measuring large PM at CAFOs, e.g., Aerosizer DSP (TSI Inc., Shoreview, MN; aerodynamic diameter: 0.3–700 µm) (Mitchell and Nagel, Citation1999). However, due to the limited availability of those instruments, they can hardly be applied to every field monitoring effort. Alternatively, an indirect approach has often been used (Wang et al., Citation2005; Buser et al., Citation2008; Wang-Li et al., Citation2013), in which PM was collected on a hydrophobic filter medium (e.g., Teflon-membrane filter) and then resuspended in a liquid medium for PSD determination. This approach exempts the use of aerosol particle size analyzers, thereby offering more flexibility in instrument selection and also simplifying the field operation.
The large particle size of PM at CAFOs also increases the risk of sampler overloading (Zhao et al., Citation2009; Yang et al., Citation2011; Van Ransbeeck et al., Citation2013). Size-selective PM sampling (e.g., PM10 and PM2.5 [PM ≤10 and ≤2.5 μm, respectively]) is achieved by separating and trapping particles with aerodynamic diameter larger than a target cut size (e.g., 10 µm for PM10 collection) from the sampling air stream. This “trapping” capacity is limited in practice, over which the penetration curve of the sampler will shift beyond the acceptable range and the monitoring results will no longer be reliable (Vanderpool et al., Citation2001; Demokritou et al., Citation2004). The dominance of large particles as well as the extremely high PM concentrations at CAFOs, therefore, poses a great challenge to the direct measurement of PMx, where x refers to the PM size of interest. In principle, the mass fraction of PMx can be estimated from the PSD of total suspended particulates (TSP), as shown in eq 1.
where FPMx is the mass fraction of PMx in TSP and is the density function of the mass-based particle size distribution of TSP. Based on the EPA’s definitions, PM10 and PM2.5 refer to particles with an aerodynamic diameter equal to or smaller than 10 and 2.5 µm, respectively. This alternative, indirect approach enables nonconventional but simpler determination of PM10 and PM2.5 mass concentrations and has been used to study PM emissions from various agricultural facilities (Wanjura et al., Citation2005; Wang-Li et al., Citation2013).
In this study, TSP was sampled at the air exhaust of 15 commercial CAFOs in the U.S. Midwest, with its mass concentration determined gravimetrically. A Horiba LA-300 particle sizer (Horiba Group, Edison, NJ) was employed to measure the PSD (in equivalent spherical diameter) of collected TSP samples. To convert equivalent spherical diameter to aerodynamic diameter, the density of deposited particles—as a surrogate for TSP—was determined using gas displacement pycnometry. The influence of animal species, housing facility type, and season on TSP concentration, size, and density was examined through statistical analysis.
Materials and Methods
Sampling description
Field sampling was carried out at five different types of CAFOs (swine finishing, swine farrowing, swine gestation, laying hen, and tom turkey farm production sites), with three farms selected for each type. On a farm, two or three animal buildings were selected for setting up TSP samplers, depending on the building availability, and two to four collocated samplers were installed per building. To examine the seasonality, each farm was visited three times: one in winter, one in spring or fall, and one in summer. The biosecurity rule mandates a 3–10-day interval between visits to different animal farms, which limited the number of field trips. In total, 45 visits were made from September 2007 to December 2008. Since then, little change has taken place to animal housing and management practices in the U.S. Midwest. Thus, we consider the monitoring data sets still representative of current animal farms in this region.
TSP was sampled using the TSP isokinetic sampling nozzle developed at the University of Illinois at Urbana-Champaign (Zhang, Citation2005). To achieve isokinetic sampling, the nozzle inlet was arranged to face and be aligned with the exhaust airstream (note: TSP nozzles were installed upstream of exhaust fans; the air downstream of the fans were highly turbulent and, thus, inappropriate for PM sampling). The installation height of the nozzle depended on the position of the fan, and the spacing of the nozzle to the fan face was typically 0.3–0.5 m, with adjustments made based on the measured air velocity. In the naturally ventilated tom turkey houses where no fans were available, a calm-air sampling protocol (Zhang, Citation2005) was followed with open-face filter cassettes installed near a downwind end door. The sampling period was 22 ± 2 hr during each field trip, and the sampling flow rate (1.2 m3 hr−1) was regulated with a venturi orifice (Wang and Zhang, Citation1999).
PM size and concentration can be affected by many environmental and operational factors, such as animal density, animal age, temperature, moisture, ventilation, feeder type, and manure handling (Duchaine et al., Citation2000). For better data interpretation, those factors were measured and documented during sampling (partly listed in ). Ambient climate conditions were also recorded, as summarized in Table S1.
Table 1. Summary of visited animal farms
Mass concentration measurement
Teflon-membrane filters (part no. P5PJ037; Pall Corp., Ann Arbor, MI) were employed for TSP sample collection. The filters were conditioned in desiccators (<25% relative humidity and 22 ± 2 °C) for 24–48 hr and then weighed on a microbalance (Ag245; Mettler-Toledo International Inc., Columbus, OH) each before and after field sampling. The mass concentration of TSP was calculated as
where, Cp is the TSP mass concentration (mg m−3), Mt is the mass of a filter with collected particles (mg), M0 is the mass of that filter before sampling (mg), Qs is the sampling flow rate (m3 hr−1), ts is the sampling duration time (hr), Tstd is the standard temperature (273.2 K), Ts is the indoor temperature during sampling (K), Ps is the indoor barometric pressure (kPa), and Pstd is the standard barometric pressure (101.325 kPa). A total of 373 filter samples were collected for mass measurement. For each visit, the reported concentration data were the average of all TSP filters taken from multiple buildings.
PSD measurement
PSD measurement was conducted using a Horiba LA-300 particle sizer (Horiba Group). This instrument measures the PSD of suspended particles in a liquid medium with equivalent spherical diameter ranging from 0.1 to 600 µm. It classifies particles into 64 size bins (Table S3) based on the principle of Mie scattering, i.e., the angle and intensity of scattered light are related to the size of particles. A particle refractive index of 1.16 –0.10i (typical of biological materials) was used for PSD calculation, as recommended by the technical manual of the Horiba instrument.
Of the 7–12 filters collected from each visit, only 3 (1 per animal building) were selected for PSD analysis. The rest of filters were submitted for other analyses such as bacterial community composition, antibiotic resistance genes, endotoxin, (1,3)-β-d-glucan, and odorants (Hong et al., Citation2012; Yang et al., Citation2013, Citation2014). The selected filters were extracted by sonication for 20 min with 20 mL of 0.1% (wt/wt) sodium hexametaphosphate (NaPMP) aqueous solution at room temperature. Here, NaPMP served as a stabilizing agent to minimize the sedimentation and flocculation of test particles (Gee and Bauder, Citation1986). It also lowered the surface tension of water, allowing for effective extraction of particles from the filters. To reduce the analysis cost, filter extracts from the same visit were combined to form a composite sample. The sample was tested three to four times, from which an average PSD was derived. For quality assurance, the instrument was calibrated with monodispersed particle size standards (10, 25, 50, 100, and 200 µm; 4000 series, Duke Scientific Corp., Fremont, CA). The calibration included a correction for the measurement bias in the size spread and median diameter of monodispersed particles. Detailed information can be found in Supplemental Material.
The measured PSDs were reported with two variables: mass median diameter (MMD) and geometric standard deviation (GSD). By assuming all sizes of particles had the same density (Buser et al., Citation2008; Wang-Li et al., Citation2013), the volume-based PSD measured by the Horiba was equivalent to mass-based PSD, and MMD and GSD could be calculated as
where d50% is the diameter at 50% cumulative particle volume fraction, fi is the volume frequency of particles in the ith size bin with their median diameter of di, and µ is the volume mean diameter of all particles (eq 5).
Density measurement
The density of particles was measured using an AccuPyc 1330 helium pycnometer (Micromeritics Instrument Corp., Norcross, GA). This instrument offers a high-speed, high-precision (less than or equal to ±0.02%) density measurement but requires the volume of a test material be greater than ~0.5 cm3. In reality, it is impractical to collect enough airborne PM for density measurement. Because in confined swine and poultry buildings, particles deposited on the surface of barn fences and exhaust fans mostly originated from previously suspended TSP, we assume that those deposited particles had the same density as TSP and submitted them as a surrogate for pycnometry analysis.
On the farm, deposited particles were collected from multiple barns and fans and then mixed to form a composite sample. The sample was tested at least three times in the laboratory, with each test offering 10 density readings (at different helium pressures). The average density value over all tests was reported.
Estimation of PM10 and PM2.5 mass fractions
As PM10 and PM2.5 are defined based on aerodynamic diameter, the measured particle size (in equivalent spherical diameter) must be first converted (eqs 6 and 7) to aerodynamic diameter.
For particles with de ≥ 3 µm:
For particles with 3 µm > de ≥ 0.1 µm:
where de is the equivalent spherical diameter, da is the aerodynamic diameter of particles, Cce and Cca are slip correction factors for da and de, χ is the shape factor and was assumed to be 1.0 in accordance with previous studies (Lacey et al., Citation2003; Wang-Li et al., Citation2013), ρ0 is the unit density (1000 kg m−3), ρp is the particle density, and λ is the mean free path of the air (0.066 µm at 101.325 kPa and 20 °C). PM10 and PM2.5 mass fractions were then estimated based on eq 1, i.e., by calculating the cumulative mass fractions of particles no larger than 10 and 2.5 µm, respectively.
Data analysis
The measurement data sets were transformed into their logarithmic forms as they failed the Shapiro-Wilk normality test (P < 0.05). One-way analysis of variance (ANOVA) was employed to examine the effects of animal species (swine and poultry), the type of housing facilities (swine farrowing, swine gestation, swine finishing, laying hen, and tom turkey), and feeder type (dry and wet) on measured TSP concentrations, size, and density. The seasonal effect was assessed using repeated-measures one-way ANOVA. A significance level of α = 0.05 was selected unless otherwise noted. All statistical analyses were performed using SAS 9.2 for Windows (SAS Institute, Cary, NC).
Results and Discussion
TSP mass concentration
Animal species and the type of housing facilities both had a significant effect on measured TSP mass concentrations (P < 0.001; ). Poultry CAFOs had overall higher TSP concentrations than swine CAFOs. The highest TSP concentrations were observed at tom turkey CAFOs (5.56 ± 4.63 mg m−3), which occasionally exceeded 10 mg m−3, whereas the lowest concentrations occurred at swine gestation CAFOs (0.55 ± 0.24 mg m−3).
Figure 1. Total suspended particulate (TSP) concentrations at five types of concentrated animal feeding operations (CAFOs). Data presented are average concentration, and error bar represents standard deviation. The dash lines are the human exposure limits proposed by Dr. Kelly Donham and his colleagues (Donham et al., Citation1995, Citation2000) for swine (2.5 mg m−3) and poultry (2.4 mg m−3) houses, respectively.
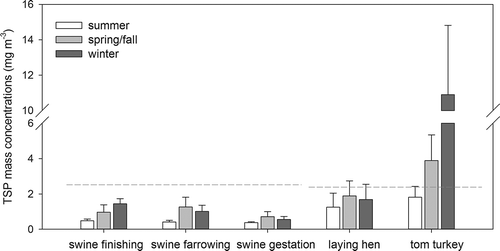
Many factors affecting the TSP concentrations are correlated with housing facility type, such as animal density, animal feed, floor type, manure management, and ventilation design. For instance, the wood shaving-litter floor in tom turkey CAFOs could be a significant PM source. In swine farrowing and gestation CAFOs, sows were confined in metal crates with limited activity, which reduced the mechanical suspension of feed and manure particles.
Seasonality also had a significant effect on the measured TSP concentrations (P < 0.001). The lowest TSP concentrations were observed in summer at all types of CAFOs (), whereas the highest concentrations occurred in winter at swine finishing and tom turkey CAFOs, or in spring/fall at swine farrowing, swine gestation, and laying hen CAFOs. A similar seasonality was reported by Takai et al. (Citation1998) and Wang-Li et al. (Citation2013).
To provide animals a comfortable thermal environment, the ventilation rate (of both natural and mechanical ventilation systems) of most CAFOs is adjusted based on ambient temperature. Although in this study no monitoring of the ventilation rate was attempted, it was evident from the number of exhaust fans in operation and/or the openness of side curtains that all visited animal buildings were ventilated more in summer and less in winter. This may explain the seasonality of measured TSP concentrations. Other factors that may affect the TSP concentration, such as animal density and air moisture, did not exhibit significant seasonal variability or correlations in this sampling campaign.
The TSP concentrations at the visited swine CAFOs were slightly lower than those reported by previous studies (Takai et al., Citation1998; Predicala et al., Citation2001; O’Shaughnessy et al., Citation2002; Bottcher et al., Citation2004), whereas the concentrations at poultry CAFOs were similar to those reported in the literature (Wathes et al., Citation1997; Hinz and Linke, Citation1998; Takai et al., Citation1998; Ellen et al., Citation2000; Redwine et al., Citation2002; Wang-Li et al., Citation2013).
Particle density
Particle density showed a significant effect by the type of housing facilities and season (P < 0.001) but no significant effect by animal species (P = 0.172; ). At all CAFOs, the highest density values were observed in summer. The deposited particles collected at swine farrowing CAFOs exhibited the highest density (1.75 ± 0.15 g cm−3). A relevant observation is that the PM sampled at swine farrowing CAFOs during the same sampling campaign had the highest mineral contents (Yang et al., Citation2011). Minerals and salts usually have a larger density than organics (e.g., limestone: 2.3–2.7 g cm−3 vs. starch: ~1.5 g cm−3). The particle density values presented here were in line with those reported by previous studies (Almuhanna, Citation2007; Jerez, Citation2007; Lee and Zhang, Citation2008).
Particle size
summarizes the PSD analysis results and their comparison with those in the literature. The measured MMDs varied significantly with the type of housing facilities (P < 0.001) and attained the largest values at tom turkey CAFOs (typically >20 µm). With a significant seasonality noted (P = 0.049), the MMDs were largest in summer and smallest in winter () at all visited CAFOs. High ventilation rates in summer reduced the TSP concentrations because of enhanced air dilution; however, they facilitated the suspension and/or resuspension of coarse particles, leading to larger MMDs. Comparatively, low ventilation rates resulted in greater air retention time, which would promote the settling of coarse particles and lead to smaller MMDs in winter. The measured GSDs () showed no significant variability with animal species (P = 0.460), the type of housing facilities (P = 0.179), or season (P = 0.412).
Table 2. Particle size distribution measurements at concentrated animal feeding operations in the United States
Figure 3. Effects of housing facility type and season on the particle size distribution of collected total suspended particulate (TSP) samples: (a) mass median equivalent spherical diameter (MMD) and (b) geometric standard deviation (GSD).
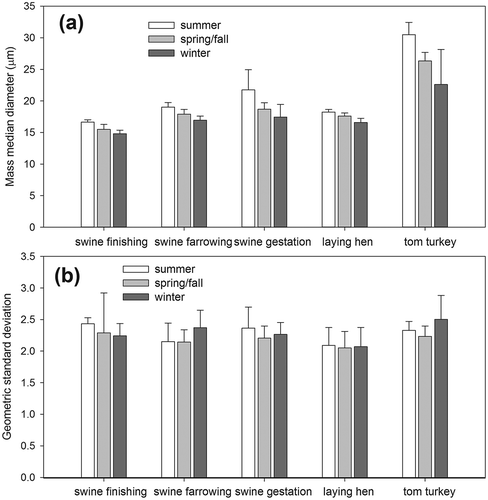
Most measured PSD profiles approximated a single-mode (peak) lognormal distribution. A bimodal lognormal distribution was occasionally observed, with a minor peak occurring between 0.1 and 2 µm (see Figure S3). A similar pattern was recently observed by Van Ransbeeck et al. (Citation2012) using a Grimm particle spectrometer to measure the PSD of PM at a swine finishing CAFO. Their measured PSDs appeared to have smaller MMDs than those in this study, which is likely because of a difference in the instrument’s size detection range (Grimm: 0.25–32 µm vs. Horiba: 0.1–600 µm).
As revealed by the PSD analysis, most sampled TSP particles were of inhalable size, i.e., with aerodynamic diameter smaller than 100 µm. Thus, the measured TSP concentrations were compared with the human exposure limits of inhalable particles (2.5 mg m−3 for swine and 2.4 mg m−3 for poultry operations) proposed by Dr. Kelly Donham and his colleagues (Donham et al., Citation1995, Citation2000). The results showed that only at tom turkey CAFOs did the TSP concentrations at the air exhaust frequently exceed the proposed human threshold limit ().
PM10 and PM2.5 mass fractions
The PM10 fraction (PM10/TSP) differed significantly with housing facility type and season (P < 0.030). The highest fraction was found at swine finishing CAFOs (21.1 ± 3.2%; ), whereas the lowest one was observed at tom turkey CAFOs (12.7 ± 5.1%). Winter was in general associated with higher PM10 fractions than summer. The values reported in the literature ranged from 6% at chicken broiler CAFOs (Redwine et al., Citation2002), 20% at swine CAFOs (Predicala et al., Citation2001), ~33% at a dairy CAFO (Kaasik and Masskimets, Citation2013), to 39% at high-rise laying hen CAFOs (Wang-Li et al., Citation2013). Note that the laying hen CAFOs visited in this study was all manure-belt laying hen houses. It is not unexpected that different ventilation designs and manure management practices would cause differences in PSD between high-rise and manure-belt laying hen facilities.
Table 3. Estimated PM10 and PM2.5 mass fractions in collected TSP samples (average ± standard deviation)
The PM2.5 fraction (PM2.5/TSP) showed a similar seasonality to that of PM10 (). Poultry CAFOs had a lower percentage of fine particles (i.e., PM2.5) than swine CAFOs. The PM2.5 fractions were below 9.0% at all visited CAFOs. This again affirmed that PM released from animal facilities, unlike ambient aerosols, mainly consist of particles larger than 2.5 µm. As most PM monitoring techniques were developed for ambient aerosols (often dominated by fine particles, i.e., PM2.5), there is a need to revisit those techniques for their applicability to agricultural PM assessment.
Effect of feeder type
Animal feeds are considered a major PM source (Curtis et al., Citation1975; Heber et al., Citation1988; Pearson and Sharples, Citation1995) and could account for ~90% of PM in swine buildings (Honey and McQuitty, Citation1979; Donham, Citation1986). In this sampling campaign, animals were fed corn-soybean meal diets (ground or pellets) with two types of feeders used: dry and wet. Animal feeds and water remained separate in the dry feeder system (adopted by swine farrowing, laying hens, and tom turkey CAFOs) but were mixed in the wet feeder system (adopted by swine gestation and finishing CAFOs). In principle, the wet feeder system would suppress the suspension of feed particles, resulting in relatively low PM concentrations (O’Connell et al., Citation2002).
As expected, the measured TSP concentrations were significantly lower at the CAFOs with wet feeders than those with dry feeders (P < 0.001; ). Wet feeders were also associated with significantly lower particle density (P < 0.001), possibly because they reduced the aerosolization of mineral salts (with typically larger density than organics) in animal feeds. The MMDs of collected TSP were significantly smaller at the CAFOs with wet feeders (P = 0.018); the reason is unknown but likely because the mechanical suspension of large feed particles was suppressed. However, cautions should be paid, as animal species and the type of housing facilities could be confounding factors causing the observed difference. To better understand the effect of feeder type, a side-by-side comparison of different feeding systems must be conducted at the same type of animal housing facilities.
Table 4. Effects of feeder type on TSP concentration, particle density, and particle size (average ± standard deviation)
Limitations and suggestions for future research
In this study, deposited particles were selected as a surrogate for TSP, and were assumed to have the same particle density as the latter. Barber et al. (Citation1991) reported that the PM suspended in the air contained more fine particles than deposited particles did; coarse particles, due to their large settling velocity, would be enriched in deposited particles. Coarse and fine particles in CAFOs could originate from different sources (Heber et al., Citation1988) and, therefore, might differ in particle density. However, a later study by Dr. André Aarnink and his colleagues (Ellen et al., Citation2000) found that suspended PM and deposited particles had similar sources and chemical composition, suggesting their similar particle density. The uncertainty of using deposited particles for density measurement should be further assessed. A direct measurement of particle density is possible by simultaneously monitoring the mobility and aerodynamic diameter of particles (DeCarlo et al., Citation2004). But the existing instruments only work for fine and ultrafine particles. How to apply them to large particles in agricultural environments remains a technical challenge.
The PSD analysis by the Horiba involved the extraction of filter-collected particles into an aqueous solution. The transformation of particles on filters, gas/particle partitioning, possible nonuniformity in extraction efficiency, and the disaggregation and aggregation of particles in the solution would cause bias and/or errors to PSD measurement. The uncertainty with the filter-based method should be better assessed in future research. However, unlike ambient aerosols, the PM in the CAFOs consists of predominantly primary particles that originate from the mechanical suspension of animal feeds, manure, and construction materials. It is reasonable to assume that the change in size of those particles over the analysis process is minor, and the measurement still provides useful information for the design and assessment of PM mitigation processes at animal facilities.
Only 15 animal farms were sampled in this study. They were selected mainly based on location (proximity to the university campus), sampling permit (approved by both animal farms and the university), and the availability of animals and houses on the target dates. There lacked a randomized experimental design. In addition, each farm was visited only three times. This limited sampling frequency (due to budget, time, and biosecurity constraints) may raise concerns over the representativeness of the monitoring data set.
The EPA and U.S. Department of Agriculture (USDA) have recently sponsored and organized a number of monitoring projects to determine the emission factors of aerial pollutants from typical CAFOs. Many of those projects entailed a long-term (e.g., 1–2 yr) monitoring plan on PM concentrations using online PM analyzers, such as tapered element oscillating microbalance (TEOM) and beta-attenuation monitor. However, because those analyzers are normally expensive and bulky, most projects were able to target only one to a few animal farms of the same type and, thus, failed to address possible farm-to-farm variations. Obviously, this long-term, limited-farm monitoring strategy and ours (multiple farms but limited sampling frequency) both have their advantages and limitations. How to compare and integrate the results from the two types of monitoring campaigns remains a big challenge and requires future efforts from agricultural engineers, statisticians, and funding agencies.
Characterization of TSP emission from CAFOs will facilitate the design of emission control devices and technologies to meet the requirements of coming regulations. Results observed in this study suggest that TSP emitted from the CAFOs were mostly composed of large particles (MMDs > 15 µm) with relatively high density (>1.5 g cm−3), implying that regular PM abatement devices, such as cyclones, fabric filters, or even a simple downward-facing exhaust duct, may be applied to mitigate the TSP emission with acceptable efficiency. Based on this study, future engineers may consider two stages of PM control devices: a relatively simple and low-cost device targeting large particles (prescreening) and a more complicated one that aims to remove small particles (e.g., PM2.5) generated in the CAFOs.
Conclusions
The concentration and mass median diameter (MMD) of collected TSP samples varied considerably with animal species, housing facility type, season, and feeder type, suggesting that the PM properties or emission data derived from a limited number of measurements may not readily apply to other U.S. CAFOs. Extensive monitoring efforts are needed to account for changes in those factors, so as to reduce the uncertainty in the regional or national PM emission inventories for CAFOs. Using the density (of deposited particles) and particle size data, the mass fractions of PM10 and PM2.5 in TSP were estimated. The estimated PM10 and PM2.5 fractions were on average 16.5 ± 5.0% and 4.4 ± 2.1% at visited CAFOs. Given the fact that TSP samples emitted from CAFOs were composed of mostly large particles with a relatively high Stokes number—which is proportional to particle density and the square of particle diameter (Zhang, Citation2005)—aerodynamic dust abatement devices could be designed to efficiently mitigate the TSP emissions from typical swine and poultry CAFOs.
Acknowledgment
The authors are grateful to all farm workers, managers, and staff for their assistance and cooperation, and Jingwei Su, Steven Ford, Matthew Robert, and Dr. Ted Funk of the University of Illinois at Urbana-Champaign for their help on allocating sampling sites and constructing sampling tools. The authors also wish to thank Dr. Xiaoliang Wang of the Desert Research Institute for providing instructions and FORTRAN codes for PSD data analysis.
Funding
This study was sponsored by a USDA NRI competitive grant (award no. 2006-35112-16671).
Supplemental Material
Supplemental data for this article can be accessed on the publisher’s website.
Supplemental Material
Download Zip (200 KB)Additional information
Funding
Notes on contributors
Xufei Yang
Xufei Yang, Jongmin Lee, and Liangcheng Yang were Ph.D. students of the Department of Agricultural and Biological Engineering at the University of Illinois at Urbana-Champaign. Xufei Yang and Jongmin Lee contributed equally to this work.
Jongmin Lee
Xufei Yang, Jongmin Lee, and Liangcheng Yang were Ph.D. students of the Department of Agricultural and Biological Engineering at the University of Illinois at Urbana-Champaign. Xufei Yang and Jongmin Lee contributed equally to this work.
Yuanhui Zhang
Yuanhui Zhang is the Innoventor Professor in Engineering at the Department of Agricultural and Biological Engineering at the University of Illinois at Urbana-Champaign.
Xinlei Wang
Xinlei Wang is a professor in the Department of Agricultural and Biological Engineering at the University of Illinois at Urbana-Champaign.
Liangcheng Yang
Xufei Yang, Jongmin Lee, and Liangcheng Yang were Ph.D. students of the Department of Agricultural and Biological Engineering at the University of Illinois at Urbana-Champaign. Xufei Yang and Jongmin Lee contributed equally to this work.
References
- Almuhanna, E.A. 2007. Dust control in livestock buildings with electrostatically-charged water spray. Ph.D. dissertation, Kansas State University, Manhattan, Kansas.
- Barber, E.M., J.R. Dawson, V.A. Battams, and R.A.C. Nicol. 1991. Spatial variability of airborne and settled dust in a piggery. J. Agric. Eng. Res. 50:107–127. doi:10.1016/S0021-8634(05)80009-5
- Baron, P.A., M.K. Mazumder, Y.S. Cheng, and T.M. Peters. 2011. Real-time techniques for aerodynamic size measurement. In Aerosol Measurement: Principles, Techniques, and Applications, 3rd ed., ed. P. Kulkarni, P.A. Baron, and K. Willeke, 313–338. Hoboken, NJ: John Wiley & Sons.
- Bottcher, R.W., K.M. Keener, R.D. Munilla, C.M. Williams, and S.S. Schiffman. 2004. Dust and odor emissions from tunnel ventilated swine buildings in North Carolina and comparison of different odor evaluation methods. Appl. Eng. Agric. 20:343–347. doi:10.13031/2013.16064
- Buser, M.D., J.D. Wanjura, D.P. Whitelock, S.C. Capareda, B.W. Shaw, and R.E. Lacey. 2008. Estimating FRM PM10 sampler performance characteristics using particle size analysis and collocated TSP and PM10 samplers: Cotton gins. Trans. ASABE 51:695–702. doi:10.13031/2013.24382
- Clanton, C.J., L.J. Johnston, and R.A. Robinson. 1999. Odor emission from mixtures of ground wine carcass material and liquid swine waste. Appl. Eng. Agric. 15:331–335. doi:10.13031/2013.5786
- Curtis, S.E., J.G. Drummond, D.J. Grunloh, P.B. Lynch, and A.H. Jensen. 1975. Relative and qualitative aspects of aerial bacteria and dust in swine houses. J. Anim. Sci. 41:1512–1520. doi:10.2134/jas1975.4151512x
- DeCarlo, P.F., J.G. Slowik, D.R. Worsnop, P. Davidovits, and J.L. Jimenez. 2004. Particle morphology and density characterization by combined mobility and aerodynamic diameter measurements. Part 1: Theory. Aerosol Sci. Technol. 38:1185–1205. doi:10.1080/027868290903907
- Demokritou, P., S.J. Lee, and P. Koutrakis. 2004. Development and evaluation of a high loading PM2.5 speciation sampler. Aerosol Sci. Technol. 38:111–119. doi:10.1080/02786820490249045
- Donham, K.J. 1986. Hazardous agents in agricultural dusts and methods of evaluation. Am. J. Ind. Med. 10:205–220. doi:10.1002/(ISSN)1097-0274
- Donham, K.J., D. Cumro, S.J. Reynolds, and J.A. Merchant. 2000. Dose-response relationships between occupational aerosol exposures and cross-shift declines of lung function in poultry workers: Recommendations for exposure limits. J. Occup. Environ. Med. 42:260–269. doi:10.1097/00043764-200003000-00006
- Donham, K.J., S.J. Reynolds, P. Whitten, J.A. Merchant, L. Burmeister, and W.J. Popendorf. 1995. Respiratory dysfunction in swine production facility workers: Dose-response relationships of environmental exposures and pulmonary function. Am. J. Ind. Med. 27:405–418. doi:10.1002/(ISSN)1097-0274
- Douwes, J., P. Thorne, N. Pearce, and D. Heederik, 2003. Bioaerosol health effects and exposure assessment: Progress and prospects. Ann. Occup. Hyg. 47:187–200. doi:10.1093/annhyg/meg032
- Duchaine, C., Y. Grimard, and Y. Cormier. 2000. Influence of building maintenance, environmental factors, and seasons on airborne contaminants of swine confinement buildings. Am. Ind. Hyg. Assoc. J. 61:56–63. doi:10.1202/0002-8894(2000)061
- Ellen, H.H., R.W. Bottcher, E. Wachenfelt, and H. Takai. 2000. Dust levels and control methods in poultry houses. J. Agric. Saf. Health 6:275–282. doi:10.13031/2013.1910
- Heber, A.J., M. Stroik, J.M. Faubion, and L.H. Willard. 1988. Size distribution and identification of aerial dust particles in swine finishing buildings. Trans. ASAE 31:882–887. doi:10.13031/2013.30794
- Hinz, T., and S. Linke. 1998. A comprehensive experimental study of aerial pollutants in and emissions from livestock buildings: Part 2. Results. J. Agric. Eng. Res. 70:119–129. doi:10.1006/jaer.1998.0282
- Hiranuma, N., S.D. Brooks, B.W. Auvermann, and R. Littleton. 2008. Using environmental scanning electron microscopy to determine the hygroscopic properties of agricultural aerosols. Atmos. Environ. 42:1983–1994. doi:10.1016/j.atmosenv.2007.12.003
- Honey, L.F., and J.B. McQuitty. 1979. Some physical factors affecting dust concentrations in a swine facility. Can. J. Agric. Eng. 21:9–14.
- Hong, P., X. Li, X. Yang, T. Shinkai, Y. Zhang, X. Wang, and R.I. Mackie. 2012. Monitoring airborne biotic contaminants in the indoor environment of swine and poultry confinement buildings. Environ. Microbiol. 14:1420–1431. doi:10.1111/j.1462-2920.2012.02726.x
- Jerez, S.B. 2007. Airborne pollutant spatial distribution, emission, and ventilation effectiveness for mechanically ventilated livestock buildings. Ph.D. dissertation, University of Illinois at Urbana-Champaign, Urbana-Champaign, Illinois.
- Kaasik, A., and M. Maasikmets. 2013. Concentrations of airborne particulate matter, ammonia and carbon dioxide in large scale uninsulated loose housing cowsheds in Estonia. Biosyst. Eng. 114:223–231. doi:10.1016/j.biosystemseng.2013.01.002
- Gee, G.W., and J.W. Bauder. 1986. Particle-size analysis. In Methods of Soil Analysis, Part 1—Physical and Mineralogical Methods, ed. E.A. Klute, 383–411. Madison, WI: American Society of Agronomy/Soil Science Society of America. doi:10.1089/jam.1999.12.217
- Mitchell, J.P., and M.W. Nagel. 1999. Time-of-flight aerodynamic particle size analyzers: Their use and limitations for the evaluation of medical aerosols. J. Aerosol Med. 12:217–240. doi:10.1089/jam.1999.12.217
- Lacey, R.E., J.S. Redwine, and C.B. Parnell. 2003. Particulate matter and ammonia emission factors for tunnel-ventilated broiler production houses in the southern U.S. Trans. ASAE 46:1203–1214. doi:10.13031/2013.13958
- Lee, J., and Y. Zhang. 2008. Evaluation of gas emissions from animal building dusts using a cylindrical convective chamber. Biosyst. Eng. 99:403–411. doi:10.1016/j.biosystemseng.2007.11.008
- Maghirang, R.G., and M.C. Puma. 1997. Airborne and settled dust levels in a swine house. ASHRAE Trans. 103:309–315.
- McMurry, P.H. 2000. A review of atmospheric aerosol measurements. Atmos. Environ. 34:1959–1999. doi:10.1016/S1352-2310(99)00455-0
- O’Connell, N.E., V.E. Beattie, and R.N. Weatherup. 2002. Influence of feeder type on the performance and behavior of weaned pigs. Livest. Prod. Sci. 74:13–17. doi:10.1016/S0301-6226(01)00283-4
- O’Shaughnessy, P.T., C. Achutan, and A.W. Karsten. 2002. Temporal variation of indoor air quality in enclosed swine confinement building. J. Agric. Saf. Health 8:349–364.
- Pearson, C.C., and T.J. Sharples. 1995. Airborne dust concentrations in livestock buildings and effect of feed. J. Agric. Eng. Res. 60:145–154. doi:10.1006/jaer.1995.1008
- Predicala, B.Z., R.G. Maghirang, S.B. Jerez, J.E. Urban, and R.D. Goodband. 2001. Dust and bioaerosol concentrations in two swine-finishing buildings in Kansas. Trans. ASAE 44:1291–1298. doi:10.13031/2013.6434
- Redwine, J.S., R.E. Lacey, S. Mukhtar, and J.B. Carey. 2002. Concentration and emissions of ammonia and particulate matter in tunnel ventilated broiler houses under summer conditions in Texas. Trans. ASAE 45:1101–1109. doi:10.13031/2013.9943
- Sweeten, J.M., C.B. Parnell, B.W. Shaw, and B.W. Auvermann. 1998. Particle size distribution of cattle feedlot dust emission. Trans. ASAE 41:1477–1481. doi:10.13031/2013.17297
- Takai, H., S. Pedersen, J.O. Johnsen, J.H.M. Metz, P.W.G. Groot Koerkamp, G.H. Uenk, V.R. Phillips, M.R. Holden, R.W. Sneath, J.L. Short, R.P. White, J. Hartung, J. Seedorf, M. Schrőder, K.H. Linkert, and C.M. Wathes. 1998. Concentrations and emissions of airborne dust in livestock buildings in northern Europe. J. Agric. Eng. Res. 70:59–77. doi:10.1006/jaer.1997.0280
- Tan, Z., and Y. Zhang. 2004. A review of effects and control methods of particulate matter in animal indoor environments. J. Air Waste Manage. Assoc. 54:845–854. doi:10.1080/10473289.2004.10470950
- Vanderpool, R.W., T.M. Peters, S. Natarajan, D.B. Gemmill, and R.W. Wiener. 2001. Evaluation of the loading characteristics of the EPA WINS PM2.5 separator. Aerosol Sci. Technol. 34:444–456. doi:10.1080/02786820117739
- Van Ransbeeck, N., H. Van Langenhove, S. Van Weyenberg, D. Maes, and P. Demeyer. 2012. Typical indoor concentrations and emission rates of particulate matter at building level: A case study to setup a measuring strategy for pig fattening facilities. Biosyst. Eng. 111:280–289.doi:10.1016/j.biosystemseng.2011.12.004
- Van Ransbeeck, N., S. Van Weyenberg, H. Van Langenhove, and P. Demeyer. 2013. Indoor concentration measurements of particulate matter at a pig fattening facility: Comparison and equivalence tests with different sampling instruments and measuring techniques. Biosyst. Eng. 115:453–462. doi:10.1016/j.biosystemseng.2013.05.001
- Wang, L.J., J.D. Wanjura, C.B. Parnell, R.E. Lacey, and B.W. Shaw. 2005. Performance characteristics of a low-volume PM10 sampler. Trans. ASABE 48:739–748.
- Wang, X., and Y. Zhang. 1999. Development of a critical airflow venturi for air sampling. J. Agric. Eng. Res. 73:257–264. doi:10.1006/jaer.1999.0414
- Wang-Li, L., Z. Cao, Q. Li, Z. Liu, and D.B. Beasley. 2013. Concentration and particle size distribution of particulate matter inside tunnel-ventilated high-rise layer operation houses. Atmos. Environ. 66:8–16. doi:10.1016/j.atmosenv.2012.03.064
- Wanjura, J.D., M.D. Buser, C.B. Parnell, B.W. Shaw, and R.E. Lacey. 2005. A simulated approach to estimating PM10 and PM2.5 concentrations downwind from cotton gins. Trans. ASABE 48:1919–1925.
- Wathes, C.M., M.R. Holden, R.W. Sneath, R.P. White, and V.R. Phillips. 1997. Concentrations and emission rates of aerial ammonia, nitrous oxide, methane, carbon dioxide, dust, and endotoxin in U.K. broiler and layer houses. Br. Poultry Sci. 38:14–28. doi:10.1080/00071669708417936
- Wathes, C.M., V.R. Phillips, M.R. Holden, R.W. Sneath, J.L. Short, R.P.P. White, J. Hartung, J. Seedorf, M. Schroder, K.H. Linkert, S. Pedersen, H, Taikai, J.O. Johnsen, P.W.G. Groot-Koerkamp, G.H. Uenk, J.H.M. Metz, T. Hinz, V. Caspary, and S. Linke. 1998. Emissions of aerial pollutants in livestock buildings in northern Europe: Overview of a multinational project. J. Agric. Eng. Res. 70:3–9. doi:10.1006/jaer.1998.0278
- Wing, S., and S. Wolf. 2000. Intensive livestock operations, health, and quality of life among eastern North Carolina residents. Environ. Health Perspect. 108:233–238. doi:10.1289/ehp.00108233
- Yang, X., Y. Lorjaroenphon, K.R. Cadwallader, X. Wang, Y. Zhang, and J. Lee. 2014. Analysis of particle-borne odorants emitted from concentrated animal feeding operations. Sci. Total Environ. 490:322–333. doi:10.1016/j.scitotenv.2014.05.026
- Yang, X., X. Wang, Y. Zhang, J. Lee, J. Su, and R.S. Gates. 2011. Characterization of trace elements and ions in PM10 and PM2.5 emitted from animal confinement buildings. Atmos. Environ. 45:7096–7104. doi:10.1016/j.atmosenv.2011.09.037
- Yang, X., X. Wang, Y. Zhang, J. Lee, J. Su, and R.S. Gates. 2013. Monitoring total endotoxin and (1→ 3)-β-d-glucan at the air exhaust of concentrated animal feeding operations. J. Air Waste Manage. Assoc. 63:1190–1198. doi:10.1080/10962247.2013.810556
- Zhang, Y. 2005. Indoor Air Quality Engineering. Boca Raton, FL: CRC Press.
- Zhao, Y., A.J.A. Aarninka, P. Hofschreudera, and P.W.G. Groot Koerkamp. 2009. Evaluation of an impaction and a cyclone pre-separator for sampling high PM10 and PM2.5 concentrations in livestock houses. J. Aerosol Sci. 40:868–878. doi:10.1016/j.jaerosci.2009.06.001