Abstract
Activated carbon (AC) is seldom applied for recovering ketone-based volatile organic compounds because of safety concerns. Adsorption of methyl ethyl ketone (MEK) with AC is a highly exothermic reaction that potentially causes fires in AC beds. Moreover, 2,3-butanediol (BDO) is produced in the desorbed solvent, causing yellowing and odor of the recovered solvent. This study applied a continuous adsorption–desorption apparatus for evaluating the operating capacities and BDO concentration in recovered MEK containing modified and original ACs. AC-1 (TAKETA- G2X) was used as the target for modification. The experimental results indicate that using MgO as the modifier increases the ignition point by 12°C and that applying KNO3 as the modifier reduces the AC ignition point by 28°C (compared with AC-1). The BDO concentration of the desorbed MEK solvent can be reduced by increasing the loading of the modifying agent (Ethanolamine) (Im-1: 3.1 wt%; Im-5: 6.2 wt%). Moreover, applying the AC pretreated with nitrogen (Im-6) as adsorbent significantly reduces the BDO concentration (from 0.123 wt% to 0.073 wt%). Because desorption and purging procedures were performed in N2 atmospheres, the BDO concentrations of the desorbed MEK solvents were relatively low and ranged from 0.032 wt% to 0.043 wt%. When the MEK concentration was reduced to 2000 ppm, lower BDO concentrations (0.012–0.022 wt%) were measured in the recovered MEK solvent. The way to modify activated carbon and a better desorbing sequence to effectively inhibit the oxidation of MEK to BDO are developed. The results obtained indicate that the BDO concentration in the desorbed solvent was lower than the original MEK solvent (0.023 wt%). Different approaches can be applied simultaneously to achieve high inhibition effects; however, carbon adsorption performance may be negatively affected.
Implications: The study is motivated to improve the quality of recovered solvent and reduce fire hazards, particularly when AC is applied for adsorbing a ketone-based solvent (e.g., MEK). The experimental results indicate that the BDO concentration in the recovered solvent can be reduced and the ignition point of AC can be increased by modifying the AC with an appropriate agent.
Introduction
Large amounts of organic solvents including ketone-based organic solvents (e.g., methyl ethyl ketone [MEK]) are used in various industrial processes, and most industries apply granular activated carbon (GAC) or activated carbon fabric (ACF) to recover volatile organic compounds (VOCs). Part of this study is based on dry polyurethane (PU) synthetic leather process exhaust; toluene and MEK are the major solvents used. MEK is selected as the ketone-containing solvent in this study. Numerous studies on the adsorption of VOCs with activated carbon (AC) have been conducted (Sun et al., Citation2012; Gnesdilov et al., Citation2007; Lou et al., 2006; Bansal and Goyal, Citation2005; Khan and Ghoshal, Citation2000; Popescu et al., Citation2001; Ruthven and Wiley, 1984), and most of the studies have applied fixed-bed adsorption systems (Lee et al., Citation2007; Kim et al., Citation2002). In addition, studies on recovering VOCs by using ACF have been reported (Liu et al., Citation2012; Huang et al., Citation2003; Lordgooei et al., Citation1998; Fuertes et al., Citation2003). The specific surface area of ACFs ranges from 700 m2/g to 2400 m2/g, which provides larger surface areas compared with commonly used GAC (Lou et al., 2006). Mass transfer of vapors to and from an ACF is enhanced because of its small nominal fiber diameter (10–12 μm). The physical properties of ACFs provide rapid adsorption and high adsorption capacities for a wide range of organic vapors (Huang et al., Citation2003; Lordgooei et al., Citation1998; Lo et al., Citation2002; Fuertes et al., Citation2003). In field application, steam is commonly applied as the heat source for regenerating AC (Shivaji et al., 2012; Duan et al., Citation2014). Hence, ketone-containing or ester-containing solvents tend to entail hydrolysis and oxidation problems in the environment containing substantial water vapor and oxygen, influencing the quality of recovered solvents and the performance of AC (Shah et al., Citation2014; Chiang et al., Citation2002).
Oxidation, decomposition, and polymerization tend to occur when MEK and other ketones are adsorbed with AC. Oxidation and decomposition reactions are exothermic (Shah et al., Citation2014; Chiang et al., Citation2002). During the oxidation process, peroxides such as 2,3-butanedione (BDO) are generated, causing increased temperature and even fire on the carbon bed. Moreover, BDO is a yellow to yellow-green and odor-causing substance. BDO generation deteriorates the quality of recovered methyl ethyl ketone (MEK) solvent and possibly causes AC fires (Gen and Ikuo, Citation2002). Studies on MEK adsorption characteristics have examined the problem of AC bed fires induced by the polymerization or catalysis of organic matter caused by exothermic reactions when ketone-based VOCs were adsorbed with AC (Sotelo et al., Citation2004; Uguina et al., Citation2005; Lee et al., Citation2008; Tai and Lee, Citation2007; Chiang et al., Citation1999).
Regarding the measurement of the catalytic capacity of AC, MEK is primarily used as the adsorbate. When MEK is adsorbed by AC, an exothermic oxidation reaction occurs and substantial heat is released (Gen and Ikuo, Citation2002). The adsorption energy of MEK is approximately 56.2 kJ/mol (780 J/g) (Pre et al., Citation2002). Long-term heat accumulation due to MEK adsorption may cause the overheating of AC beds, severely influencing the efficiency and safety of the AC beds (fire hazard) (Zerbonia and Brockmann, 2001). BDO is produced during the process of MEK recovery. Converting MEK into BDO is thermodynamically favorable (C4H8O[MEK] + O2 → C4H6O2 [BDO] + H2O). Under oxygen-containing and high-temperature conditions (typically ≥100ºC), part of the MEK is converted into BDO. However, studies on MEK oxidation to BDO are limited. A previous study indicates that the BDO concentration can be reduced from 700 ppm to 300 ppm if the desorption temperature and pressure of an AC bed are decreased from 100°C to 80°C and from 1 atm to 0.47 atm, respectively (Gen and Ikuo, Citation2002). Typically, MEK concentration, oxygen content, or desorption temperature must be decreased to reduce BDO generation.
The ignition point of most ACs is within the range of 450°C to 500°C, and some are less than 450°C. The ignition point mainly depends on the ash content of the AC. Ash is primarily composed of alkali metals, alkaline earth metals, and alkali salts. In addition, a considerable amount of oxygen-containing functional groups (OCFGs) exists on the surface of ACs. A previous study indicated that the amount of OCFGs of the AC increased from 197 ± 4 μeq/g to 240 ± 4 μeq/g when ozone was applied for modifying the AC (Chiang et al., Citation2002). The purpose of the modification was to reduce the oxidation reactions of the AC, and the most direct approach was to reduce the OCFGs. A previous study indicated that the amount of OCFGs of AC decreased after the AC was treated in a nitrogen atmosphere at 350°C (Gen and Ikuo, Citation2002). The other route was to reduce the acidity of the AC, such as by adding a basic compound. In addition, sulfides and nitrides can be applied for modifying AC to reduce the activity of metal impurities and the interaction between organic solvents and oxygen. Sulfur-or nitrogen-containing modifiers generate strong bonds with metals even at room temperature, reducing the activity of metal surfaces to prevent the susceptibility to oxidation and effectively increasing the ignition point of AC (Gen and Ikuo, Citation2002).
This study focuses on the solvent recovery process for designing and constructing a continuous fixed-bed activated carbon adsorption–desorption apparatus as the main test system. The main goals of this research are to reduce the BDO concentration of the recovered organic solvent containing MEK and to increase the ignition point of AC in order to reduce the associated fire hazard. Relatively favorable adsorption–desorption efficiencies of recovering MEK were achieved by using commercial GACs. However, BDO concentration was relatively high in the MEK solvent recovered using these commercial ACs. Thus, different agents exhibiting varying loadings were applied for modifying AC-1, and the effects were experimentally evaluated. Moreover, the effects of desorption temperature, desorption medium, and OCFGs on adsorption and desorption processes were experimentally evaluated. The objective is to develop a method that could be applied for effectively reducing the BDO concentration in recovered solvents and enhancing the ignition point of AC. The molecular diameter of VOCs was between approximately 3 Å and 8.5 Å (Chang, 2002). For surface adsorption, the ratio of pore diameter to the diameter of adsorbate is a key parameter, and the proportion of micropores (<20 Å) of AC is critical to the effective adsorption of VOCs (Wu and Yuo, Citation2007; Mauguet et al., Citation2005; Chiang et al., Citation2001; Yamamoto et al., Citation2004).
Experimental
Four types of commercial GAC (φ = 4 mm) were used as the main adsorbents for the tests. AC-1 was composed of coconut shell (TAKETA-G2X, Japan), whereas AC-2, AC-3, and AC-4 either were derived from coal or were anthracite coal-based. AC-2 was CTC-100 (China), AC-3 was WS-490 (China), and AC-4 was HP-106 (United States). shows the characteristics of the ACs. In addition, one ACF was applied for comparative performance tests. It was provided by Symbio, Inc. (phenolic resin series), and made in China. Another commercial modified AC (KOH) was used for an ignition test and comparison. MEK and toluene were used as adsorbates. shows the characteristics of MEK and toluene. The specific surface area and pore diameter of the adsorbents were measured using the Micromeritic ASAP2000 analyzer. Total ash was measured with an x-ray fluorescent analyzer (XRF: Philip, PW 2400; ASTM-D2866-83), the sample weight was 10 g ± 0.1 mg, and the heating temperature was 650 ± 25ºC (forced circulation). Ignition point was measured by thermal gravimetric analysis (METTLER TGA/SDTA 851), the airflow rate was 80 mL/min, heating rate was 10ºC/min, and the operating temperature range was 25 to 800ºC. Continual cyclic adsorption–desorption tests were performed for comparing the MEK adsorption capacities, amount of BDO generated, and ignition points of modified and original ACs.
Continual Adsorption–Desorption Cycle Test
Adsorption
The experimental setup for the continual AC adsorption–desorption test is shown in . The diameter of the fixed bed reactor is 2.72 cm and the length of the fixed bed reactor is 30 cm. Adsorption condition was MEK = 4000 ppm in air, gas velocity = 0.3 m/sec, adsorption temperature of 30°C, relative humidity (RH) < 10%. After the AC was baked in an oven (140–150°C, 4 hr), it was removed and placed in a drying pan for cooling. The required AC was weighed (W1) and placed in a fixed bed reactor for the adsorption test. Compressed air at a constant flow rate was injected into bubble vials (maintained at 20°C) containing organic solvent (MEK or toluene). The concentration of single (4000 ppm MEK or 4000 ppm toluene) or binary (2000 ppm MEK + 2000 ppm toluene) vapor was controlled by regulating the rotameters. The gas was then introduced into the reactor for an experimental test (maintained at 30°C). The experimental test continued until the adsorbent achieved saturation, which is defined as time at which the outlet VOC concentration attained 95% of the inlet concentration. The vapor concentration in the gas stream was measured using a VOC analyzer (Rosemount Analytical, Inc., model 400A, hydrocarbon analyzer). The AC was then removed from the reactor and weighed (W2) for calculating the saturated MEK adsorption capacity.
Desorption
The AC was reinstalled into the reactor after being weighed. The valves were then switched to the desorption process. The temperature of the cooler was set at 5°C, and the heating tapes were activated until the required temperature (100°C or 130°C) of the desorption medium was achieved. The desorption time was 10 min. When the center of the reactor achieved approximately 35°C, a desorption medium was introduced into the reactor. Desorbed solvent was then collected. When the set desorption time was fulfilled, the heating and desorption medium were terminated and the temperature began to decline. Thereafter, purged compressed air was injected (nitrogen has been used in certain experimental tests) into the reactor until the central temperature decreased to room temperature. Desorbed solvent was collected and weighed (W3) for calculating the working capacity of the AC. The working capacity was defined as the difference between the saturated VOC adsorption capacity and the residual VOCs on carbon after the purge. The composition of the solvent was then analyzed using a gas chromatograph (HP-5890 Series II). A BDO calibration curve was established as a basis for calculating BDO concentration in the desorbed solvent:
wehre θ (wt%) is the saturated MEK adsorption capacity of AC, θ 1 (wt%) is the MEK working capacity of AC, W1 is the mass of AC before adsorption, W2 is the mass of AC after saturation was achieved, and W3 is the mass of solvent desorbed from AC.
The adsorption zone length is calculated as follows:
where Z is the carbon bed height (m), Za the adsorption zone length, Te the time at C = C0 × 0.95 (min), Tb the time at C = C0 × 0.05 (min), and F the fraction of the nonadsorbed zone, which is generally 0.4 to 0.5.
Analysis of Functional Groups
OCFGs are the major functional groups existing on carbon surfaces. These functional groups may include carboxyl, anhydride, lactone, phenolic, carbonyl, ether, and pyrone (Li et al., Citation2011). According to the literature (Gen and Ikuo, Citation2002; Thrower, Citation1989), the contents of carboxyl, lactone, phenolic, and carbonyl groups can be analyzed using a titration method. Sodium bicarbonate (NaHCO3) can react with only a carboxyl group, whereas sodium carbonate (Na2CO3) can react with carboxyl and lactone groups. Moreover, sodium hydroxide (NaOH) can react with carboxyl, lactone, and phenolic groups, whereas sodium ethoxide (C2H5ONa) can react with carboxyl, lactone, phenol, and carbonyl groups. OCFGs were analyzed with an acid–base neutralization reaction by using different alkaline solutions (Gen and Ikuo, Citation2002). Hydrochloric acid (HCl) was then used for performing back-titration on the remaining alkali solutions. The values of back-titration of four alkali solutions were subtracted from each other to obtain the relative proportions of the carboxyl, lactone, phenolic, and carbonyl groups. The detailed procedure is presented as follows. First, 0.05-N solutions of NaHCO3, Na2CO3, NaOH, and C2H5ONa were prepared. Subsequently, 0.2 g carbon was placed into Erlenmeyer flasks and 25 g of the aforementioned alkali solutions were added individually. The flasks were then placed in an oscillator for 24 hr (40°C, 100 rpm), enabling complete reactions. The solutions were filtered and back-titrations were performed on the filtered solutions by using 0.05 N HCl. Thereafter, the titration endpoint and amount of HCl used were recorded for calculating the proportions of various functional groups.
Modification of AC
Commonly used commercial modifiers are applied in this study. The main function of the modifier is to form the bonding with metal components of ash in activated carbon and to reduce the reactivity of activated carbon with ketone. Modifiers used included ethanolamine, ethylenediamine, MgO, and KNO3, for possibly reducing BDO generation and enhancing the ignition point of the AC. Before modification, the AC was baked and dried at 150°C for 4 hr. shows the amount of original carbon, modifier, and impregnation solvent used for the modification.
Results and Discussion
Surface characteristics of ACs and adsorption–desorption tests
presents the results of the surface functional group analysis for AC-1, AC-2, AC-3, and AC-4. The carbonyl group did not appear on all four types of AC surface, and the phenolic group was the primary functional group, followed by the lactone and carboxyl groups as secondary and tertiary, respectively. The ash content in the AC, ranked from the highest to lowest, was AC-3 > AC-2 > AC-4 > AC-1, as shown in . The results of ignition-point analysis indicated that AC-3 > AC-4 > AC-2 > AC-1 (AC-4 ≒ AC-2). The results showed that a high ash content in the AC caused a high ignition point. AC-3 was a coal-based AC that exhibited a high ash content. The ash of AC-3 contained a high proportion of Fe (42%) (high metal content). AC-1 was a coconut-based AC that exhibited a low ash content. shows the results of ash composition analysis and indicates that Si is the major element (81%) in the ash content of AC-1.
Table 1. Characteristics of the ACs evaluated.
Five consecutive MEK adsorption–desorption tests were conducted with AC-1 to AC-4 and ACF under the same condition. After desorption, compressed air was used to cool and dry the ACs. The average operating capacities, length of adsorption zone, and BDO concentrations in the desorbed solvents were calculated, as shown in and . The BDO concentration of the original MEK solvent was 0.023%, and this value was compared with that obtained in the follow-up AC tests. The average BDO concentration in the desorbed solvents of AC-1 to AC-4 yielded an order of AC-1 > AC-2 > AC-4 > AC-3, and the value varied from 0.104 wt% to 0.123 wt%. However, the BDO concentrations of the recovered MEK with all original ACs were higher than those of the original MEK solvent (0.023%). In particular, the BDO concentration increased gradually as the adsorption–desorption cycle increased.
Table 2. Characteristics of two adsorbates tested.
Table 3. Original carbon, modifier, and impregnation solvent used.
Table 4. Adsorption capacity and average BDO concentration in the desorbed solvent with various ACs evaluated.
Regarding the saturated desorption capacities and working capacities, the order was AC-1 > AC-4 > AC-2 > AC-3. The length of adsorption zone in descending order was AC-2 > AC-3 > AC-4 > AC-1. Regarding pore properties, the size of specific surface areas in descending order was AC-2 > AC-1 > AC-4 > AC-3, and the average pore diameter in descending order was AC-2 > AC-3 > AC-4 > AC-1, as shown in . The experimental results indicated that the average pore diameter of AC-1 was the smallest among the four ACs tested. MEK is a polar organic molecule exhibiting a low boiling point and low molecular weight. The molecular diameter was approximately 5.25 Å. Thus, the specific surface areas, pore volume, average pore diameter, and length of adsorption zone are often used to determine the activated carbon adsorption capacity. The adsorption and working capacities of AC-1 to MEK were the highest, and its length of adsorption zone was the shortest among the four ACs tested. The short length of the adsorption zone prolonged the operation time of the AC. Thus, we determined that AC-1 was most suitable for the recovery of the MEK solvent among the four ACs tested. However, AC-1 exhibited the highest BDO concentration and lowest ignition point among the four commercial ACs tested. Thus, AC-1 was used as the primary AC to be modified for subsequent experiments. ACF exhibited a higher MEK adsorption capacity compared with AC-1 to AC-4. The BDO concentration of ACF ranged from 0.04% to 0.07%, yielding an average value of 0.06%, which was substantially lower than that of GAC-1 to GAC-4. In addition, the ACF maintained a stable BDO concentration without a rapid increase. The ACF exhibited a relatively higher specific surface area, adsorptivity, and desorptivity and a lower pressure drop compared with granular carbon (Huang et al., Citation2003; Fuertes, et al., Citation2003). Moreover, ACF can reduce fire hazards caused by heat accumulation on AC beds. Thus, ACF is suitable for recovering MEK solvents. However, the high cost has limited its applications in VOC recovery.
Autoignition phenomenon of AC
Furthermore, this study involved testing a commercial modified AC (KOH/AC) that has been treated with KOH to remove sulfides. A large amount of KOH (10–13 wt%) was loaded onto the AC surface and the adsorbing capacity of the MEK was substantially reduced to 8 wt%, which is significantly lower than that achieved with the four original ACs (20.8–29.3 wt%). Moreover, the BDO concentration in the desorbed solvent was relatively high (0.174 wt%) if compared with that of the four original ACs, AC-1 to AC-4 (range: 0.104–0.123 wt%). In addition, the modified AC (KOH/AC) exhibited a relatively low ignition point (281°C) (thermogravimetric analyzer, METTLER TGA/SDTA 851). When compressed air (room temperature) was injected to cool the AC bed, autoignition occurred on the AC (KOH/AC). White smoke was observed at the bottom outlet of the AC bed. Based on the experimental results, we inferred that the AC modified with high-loading KOH was unsuitable for recovering MEK. The low ignition point considerably induced the autoignition phenomenon in the AC. A previous study indicated that when the gas stream containing cyclohexanone (2000 ppm) was introduced into the four types of AC beds at a temperature of 85°C, all of the tests revealed a rapid temperature increase (Gen and Ikuo, Citation2002). Thus, ketone compounds easily lead to a rapid temperature increase and even AC bed fires during the adsorption–desorption process.
Influence of desorption temperature on BDO concentration
shows the effects of desorption temperature (100°C or 130°C) on the BDO concentration of the recovered solvent when AC-1 was applied as an adsorbent. In the first cycle, the BDO concentration of the recovered solvent desorbed at a temperature of 130°C was higher than that at 100°C. However, in the second to fifth cycles, the BDO concentration of the solvent desorbed at 100°C increased from 0.217 wt% to 0.25 wt%, whereas the BDO concentration in the solvent desorbed at 130°C increased only slightly from 0.104 wt% to 0.15 wt%. Moreover, when the desorption temperature was controlled at 100°C, the BDO concentration of the desorbed solvent after the second cycle was significantly higher than that desorbed at 130°C. A previous study indicated that the BDO concentration was reduced from 700 ppm to 300 ppm when the desorption temperature and pressure of AC were decreased from 100°C to 80°C and from 1 atm to 0.47 atm, respectively (Gen and Ikuo, Citation2002). Decreasing desorption temperature results in increased residual MEK on AC and increases the BDO concentration of the recovered solvent (from 0.123 wt% to 0.199 wt %). Moreover, the saturated adsorption capacity was reduced from 29.3 wt% to 26.8 wt%, and the average working capacity was reduced from 22.5 wt% to 16.5 wt %. Effective solvent recovery is critical to the continual adsorption–desorption operation of ACs, and complete desorption of solvents is preferred to maintain good adsorption performance and to reduce the BDO formation. This study indicates that reducing only the desorption temperature is not an effective way to reduce BDO concentration. Reducing desorption pressure should also be considered for reducing the residual MEK and BDO formation.
Effects of a modifier on ignition point
After modifying with ethanolamine (EA), ethylene diamine (EDA), KNO3, and MgO (designated as Im-1, Im-2, Im-3, and Im-4, respectively), the ignition points of the modified ACs were analyzed (thermogravimetric analyzer, METTLER TGA/SDTA 851), and the results are shown in . Among the four (EA, EDA, KNO3, and MgO) modifiers tested, MgO increased the ignition point by 12°C. EA and EDA increased the ignition point by 3°C and 4°C, respectively. However, applying KNO3 as the modifier decreased the ignition point of the AC by 28°C, possibly because of the oxidative property of KNO3 that prompted the autoignition of the AC. Thus, using an appropriate modifier is critical for increasing the ignition points.
Table 5. Effect of various commercial modifiers on ignition point.
Effects of modifier loadings
We further compared the performance of AC-1 modified with EA by using different loadings (Im-1: 3.1%; Im-5: 6.2%). shows that modifier loading influences the adsorption capacity of the AC. When the loading was less than 3.1% (Im-1), the saturated adsorption capacity of Im-1 was decreased from 29.3 wt% (AC-1) to 27.6 wt%. However, a substantial influence was observed when the loading was increased to 6.2% (Im-5). The saturated adsorption capacity of Im-5 was decreased from 29.3 wt% (AC-1) to 20.7 wt%, and the working capacity decreased from 22.5 wt% (AC-1) to approximately 9.6 wt%. In addition, the adsorption zone increased from 90 mm to 164 mm. shows that the average BDO concentration of the recovered solvent with two types of modified carbon (Im-01, Im-05) tested was substantially lower than that of AC-1. The BDO concentration of Im-1 was slightly lower than that of AC-1 during all of the test cycles. The average BOD concentration of Im-5 was 0.075%, which was significantly lower than that of AC-1 (0.123 wt%) and Im-1 (0.111 wt%), indicating the inhibiting effect of the modifier. Based on the aforementioned test results, a high modifier loading may result in the blockage of the AC pore. Thus, AC performance might be adversely affected. However, the results of increasing the loading of modifier agent effectively inhibited the BDO concentration. Therefore, determining how to achieve a balance between the reduction of BDO concentration and AC adsorption performance is worthwhile.
Table 6. Comparison of AC desorption performance and BDO concentrations with different modifier loadings.
Effects of OCFGs on BDO concentrations
Im-6 represents the AC-1 being processed in a nitrogen atmosphere at 350°C for four hours to remove partial OCFGs, whereas Im-7 refers to the AC-1 being treated with ozone for 24 h at a concentration of 200 ppm and flow rate of 6 L/min). shows the adsorption and desorption characteristics of MEK and the BDO concentration of Im-6 and Im-7 based on an identical adsorption–desorption procedure during three cycles. The results indicated that the BDO concentration in the desorbed solvent of Im-6 during the first cycle was 0.023 wt%, and 0.089 wt% and 0.106 wt% during the second and third cycles, respectively (the average was 0.073 wt%), which were slightly lower than that of AC-1 (0.123 wt%). The BDO concentration in the desorbed solvent of Im-7 during the first cycle was approximately 0.116 wt%, which was slightly lower than that of AC-1 (0.123 wt%). However, during the second and third cycles, the BDO concentrations sharply increased to 0.576 wt% and 0.723 wt%, respectively (the average was 0.472 wt%), exhibiting a fourfold increase compared with that of AC-1 (0.123 wt%).
Figure 7. Comparison of BDO concentrations in the desorbed solvents achieved with modified ACs (Im-6, Im-7).
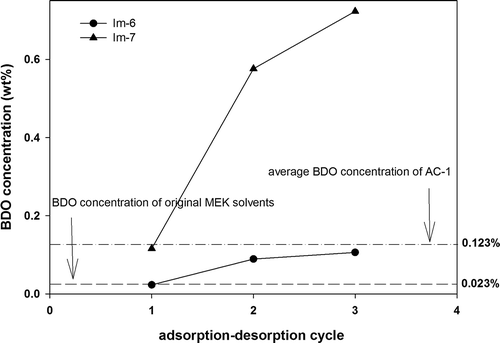
When we compared the OCFGs content of AC-1 with that of Im-6 (), the phenolic group decreased from 0.493 mmol/g (AC-1) to 0.361 mmol/g, the lactone group decreased from 0.14 mmol/g (AC-1) to 0.042 mmol/g, and the carboxyl group decreased from 0.08 mmol/g (AC-1) to 0.028 mmol/g. The results revealed a similar trend to that of a previous study (Gen and Ikuo, Citation2002). When we compared the OCFG contents in AC-1 and Im-7, the phenolic group increased from 0.493 mmol/g (AC-1) to 0.614 mmol/g, the lactone group increased from 0.140 mmol/g (AC-1) to 0.145 mmol/g, and the carboxyl group increased from 0.08 mmol/g (AC-1) to 0.225 mmol/g. The results showed a trend similar to that of previous findings. Based on the aforementioned test results, the AC treated with nitrogen (Im-6) showed a low OCFG content (from 0.713 mmol/g to 0.431 mmol/g) and reduced BDO production (from 0.123 wt% to 0.073 wt%). By contrast, the AC treated with ozone (Im-7) revealed a high OCFG content (from 0.713 mmol/g to 0.984 mmol/g) and increased BDO concentration from 0.123 wt% (AC-1) to 0.472 wt%.
Effects of feeding toluene on BDO concentration
In this study, an alternate feeding method involving MEK (4000 ppm) and toluene (4000 ppm) was applied for the experiment. First, a modified AC (Im-2) was fed with MEK only for conducting an adsorption–desorption test. The average BDO concentration in the desorbed solvent of Im-2 was 0.099 wt%, as shown in . The BDO concentration in the desorbed solvent of the first cycle was 0.032 wt%; however, the BDO concentration increased to 0.098 wt% in the second cycle. Subsequently, toluene was fed during the third to fifth cycles, and the BDO concentration in the desorbed solvents decreased substantially. When MEK was fed during the sixth and seventh cycles, the BDO concentration increased again. After repetitive operations (toluene during the eighth and ninth cycles and MEK during the 10th and 11th cycles), the results showed that feeding MEK into Im-2 for adsorption clearly increased the BDO concentration, whereas feeding toluene clearly decreased the BDO concentration of the recovered solvent. These results indicated that the BDO concentration in the recovered solvent gradually increased as the number of adsorption–desorption cycles increased when the MEK was adsorbed with AC. However, the BDO concentration could be reduced if toluene is alternately applied as the adsorbate.
Effects of using N2 as the desorption medium on BDO concentration
The adsorption–desorption procedure of AC can be divided into adsorption, desorption, and purging steps. Adsorption is consistently performed in an oxygen-containing atmosphere. However, the conditions for performing desorption and purging may be changed. Experimental tests were conducted using modified carbon Im-2 as the adsorbent and replacing steam as the desorption medium with N2. The desorption temperature was controlled at 200°C (Max.). Im-2 could be regenerated by high-temperature N2. After desorption, N2 was used to cool the carbon to room temperature, yielding an average working capacity of 19.6 wt%, which was higher than the average working capacity (13.8%) of Im-2 when it was desorbed by steam and purged by dry air (average working capacity of AC-1 was 22.5%). Regarding the amount of BDO produced, the results showed () that the desorption and purging procedures performed in N2 atmospheres effectively inhibited BDO production. MEK (4000 ppm) feeding produced an approximately 0.032 wt% to 0.043 wt% BDO concentration in the desorbed solvents, which were all lower than that of AC-1 (0.123 wt%). When the gas stream containing a low concentration of MEK (2000 ppm) was evaluated, the BDO concentration in the desorbed solvent was measured as 0.01 wt% to 0.023 wt%. This was possibly due to the low amount of MEK adsorbed on Im-2 when the feeding concentration of MEK was decreased. In addition, changing steam desorption into nitrogen desorption effectively inhibited BDO production.
shows the results of the desorption tests of AC-1 and modified carbon (Im-2) in mixed feeding (MEK 2000 ppm + toluene 2000 ppm) and nitrogen desorption conditions. During the first and second cycles, the BDO concentration of AC-1 in the desorbed solvents increased from 0.05% to 0.06%. During the third to fifth cycles, the BDO concentration was maintained at approximately 0.03%, which was slightly higher than that of the original MEK solvent (0.0115%). During the first to fourth cycles, the BDO concentration in the desorbed solvents with Im-2 was adsorbent when maintained between 0.01% and 0.025%, exhibiting a declining trend. The effectiveness of BDO inhibition was remarkable. The fifth cycle was operated for 3 days to evaluate the effects of operating time on BDO production. The results showed that the BDO concentration increased slightly to approximately 0.027%. Because MEK was adsorbed to the pores of the AC, a part of the MEK was oxidized. Although modifiers could postpone the oxidation process, they cannot completely prevent BDO generation. In particular, the long-term accumulation of oxide products may continue.
Conclusion and Recommendations
This study evaluated several methods used for increasing the ignition point of AC and reducing the BDO concentration of recovered MEK solvent. The results indicate that using an appropriate modifier and loading, decreasing OCFG content, using N2 as desorption and purging gases, and decreasing MEK concentration can effectively inhibit MEK oxidation to produce BDO. In addition, different approaches, such as applying modified carbon, using N2 as desorption and purging gases, and decreasing the feeding concentration of MEK, can be applied simultaneously to achieve high inhibition effects. Although these methods exhibited satisfactory effectiveness in inhibiting BDO concentration, carbon adsorption performance may be negatively affected. We expect that the results of this study can broaden the application of GAC (fixed bed) for recovering or processing ketone-based VOCs.
Funding
The authors express their gratitude for the funding provided by the Ministry of Economic Affairs (Small Business Innovation Research Program), Taiwan, Republic of China, 2009.
Additional information
Funding
Notes on contributors
Kai Chun Nien
Kai Chun Nien is a Ph.D. student with the Graduate Institute of Environmental Engineering, National Central University, Taoyuan, Taiwan.
Feng Tang Chang
Feng Tang Chang is the chairman of JG Environmental Technology Co., Ltd, Taiwan.
Moo Been Chang
Moo Been Chang is a Distinguished Professor with the Graduate Institute of Environmental Engineering, National Central University, Taoyuan, Taiwan.
References
- American Society Testing and Materials (ASTM) D2866-83 method: Total Ash Content of Activated Carbon.
- Bansal, R.C., and M. Goyal. 2005. Activated Carbon Adsorption. Boca Raton, FL: CRC Press.
- Chiang, H.L., P.C. Chiaing, Y.C. Chiang, and E.E. Chang. 1999. Diffusivity of microporous carbon for benzene and methyl-ethyl ketone adsorption. Chemosphere 38:2733–46. doi:10.1016/S0045-6535(98)00475-5
- Chiang, Y.C., P.C. Chiang, and C.P. Huang. 2001. Effects of pore structure and temperature on VOC adsorption on activated carbon. Carbon 39:523–34. doi:10.1016/S0008-6223(00)00161-5
- Chiang, H.L., P.C. Chiang, and C.P. Huang. 2002. Ozonation of activated carbon and its effects on the adsorption of VOCs exemplified by methylethylketone and benzene. Chemosphere 47:267–75. doi:10.1016/S0045-6535(01)00216-8
- Chiang, H.L., C.P. Huang, and P.C. Chiang. 2002. The adsorption of benzene and methylethylketone onto activated carbon: thermodynamic aspects. Chemosphere 46:143–56. doi:10.1016/S0045-6535(01)00126-6
- Chang, F.T. 2005. Characteristics of next generation panel plant volatile organic gas purification equipment. Ph.D. thesis, National Tsing Hua University, Hsinchu, Taiwan.
- Duan, X.H., C. Srinivasakannan, and J.S. Liang. 2014. Process optimization of thermal regeneration of spent coal based activated carbon using steam and application to methylene blue dye adsorption. J. Taiwan Inst. Chem. Eng. 45: 1618–27.
- Fuertes, A.B., G. Marban, and D.M. Nevskaia. 2003. Adsorption of volatile organic compounds by means of activated carbon fibre-based monoliths. Carbon 41:87–96. doi:10.1016/S0008-6223(02)00274-9
- Gnesdilov, N.N., K.V. Dobrego, and I.M. Kozlov. 2007. Parametric study of recuperative VOC oxidation reactor with porous media. Int. J. Heat Mass Transfer. 50:2787–94. doi:10.1016/j.ijheatmasstransfer.2006.11.004
- Gen, L., and A. Ikuo. 2002. Application of activated carbon technology, Japan.
- Huang, Z.H., F. Kang, K.M. Liang, and J. Hao. 2003. Breakthrough of methyethylketone and benzene vapors in activated carbon fiber beds. J. Hazard. Mater. 98:107–15. doi:10.1016/S0304-3894(02)00284-4
- Khan, F.I., and A.K. Ghoshal. 2000. Removal of volatile organic compounds from polluted air. J. Loss Prevent. Proc. 13:527–45. doi:10.1016/S0950-4230(00)00007-3
- Kim, S.J., S.Y. Cho, and T.Y. Kim. 2002. Adsorption of chlorinated volatile organic compounds in a fixed bed of activated carbon. Korean J. Chem. Eng. 19:61–67. doi:10.1007/BF02706875
- Lordgooei, M., M.J. Rood, and R.A. Massoud. 1998. Sorption of toxic chemical vapors in fixed bed adsorbers containing activated carbon fiber cloth and modeling of diffusivity and mass transfer. Proceedings of the Air & Waste Management Association’s 91st Annual Meeting & Exhibition, San Diego, California, June 14–18, 121–42.
- Lo, S.Y., D. Ramirez, M.J. Rood, K.J. Hay. 2002. Characterization of the physical, thermal and adsorption properties of a series of activated carbon fiber cloths. Proceedings of the Air & Waste Management Association’s 95th Annual Conference and Exhibition, Baltimore, MD.
- Luo, L., D.M. Ramirez, M.J. Rood, G. Grevillot, K.J. Hay, D.L. Thurston. 2006. Adsorption and electrothermal desorption of organic vapors using activated carbon adsorbents with novel morphologies. Carbon 44:2715–23. doi:10.1016/j.carbon.2006.04.007
- Lee, M.G., S.W. Lee, and S.H. Lee. 2006. Comparison of vapor adsorption characteristics of acetone and toluene based on polarity in activated carbon fixed-bed reactor. Korean J. Chem. Eng. 23:773–78. doi:10.1007/BF02705926
- Lee, S.W., S.K. Kam, and M.G. Lee. 2007. Comparison of breakthrough characteristics for binary vapors composed of acetone and toluene based on adsorption intensity in activated carbon fixed-bed reactor. J. Ind. Eng. Chem. 13:911–16.
- Lee, S.W., J.K. Cheon, H.J. Park, and M.G. Lee. 2008. Adsorption characteristics of binary vapors among acetone, MEK, benzene, and toluene. Korean J. Chem. Eng. 25:1154–59. doi:10.1007/s11814-008-0190-3
- Li, N., X. Ma, Q. Zha, K. Kim, Y. Chen, and C. Song. 2011. Maximizing the number of oxygen-containing functional groups on activated carbon by using ammonium persulfate and improving the temperature-programmed desorption characterization of carbon surface chemistry. Carbon 49:5002–13. doi:10.1016/j.carbon.2011.07.015
- Li, L., Z. Sun, H. Li, and T.C. Keener. 2012. Effects of activated carbon surface properties on the adsorption of volatile organic compounds. J. Air Waste Manage. Assoc. 62:1196–202. doi:10.1080/10962247.2012.700633
- Liu, Z.H., J.R. Qiu, H. Liu, Z.Q. Tan, Z.Q. Yan, M.L. Zhang, H.C. Zhang, and H. Yang. 2012. Effects of SO2 and NO on removal of VOCs from simulated flue gas by using activated carbon fibers at low temperatures. Fuel Process. Technol. 40:93–99. doi:10.1016/S1872-5813(12)60008-5
- Mauguet, M.C., A. Montillet, and J. Comiti. 2005. Macrostructural characterization of granular activated carbon beds. J. Mater. Sci. 40:747–55. doi:10.1007/s10853-005-6316-7
- Popescu, M., J.P. Joly, J. Carre, and C. Danatoiu. 2001. Dynamical adsorption and temperature-programmed desorption of VOCs (toluene, butyl acetate and butanol) on activated carbons. Carbon 41:739–48. doi:10.1016/S0008-6223(02)00391-3
- Pre, P., F. Delage, C. Faur-Brasquet, and P. Le Cloirec. 2002. Quantitative structure–activity relationships for the prediction of VOCs adsorption and desorption energies onto activated carbon. Fuel Process. Technol. 77–78: 345–51.
- Ruthven, D.M. 1984. Principles of Adsorption and Adsorption Process. New York, NY: John Wiley.
- Ramalingam, S.G., P. Pré, S. Giraudet, L. Le Coq, P. Le Cloirec, O. Baudouin, and S. Déchelotte. 2012. Recovery comparisons-hot nitrogen vs steam regeneration of toxic dichloromethane from activated carbon beds in oil sands process. J. Hazard. Mater. 205–6:222–28. doi:10.1016/j.jhazmat.2011.12.062
- Sotelo, J.L., M.A. Uguin, J.A. Delgado, and L.I. Celemin. 2004. Adsorption of methyl ethyl ketone and trichloroethene from aqueous solutions onto activated carbon fixed-bed adsorbers. Sep. Purif. Technol. 37:149–160. doi:10.1016/j.seppur.2003.09.006
- Shah, I.K., P. Pre, and B.J. Alappat. 2014. Effect of thermal regeneration of spent activated carbon on volatile organic compound adsorption performances. J. Taiwan Inst. Chem. Eng. 45:1733–38. doi:10.1016/j.jtice.2014.01.006
- Tai, H.M., and C.L. Lee. 2007. Desorption of methy-ethyl-ketone from granular activated carbon with microwave radiation. Environ. Prog. Sunstain. Environ. 26(3):299–303. doi:10.1002/ep.10224
- Thrower, P.D., ed. 1989. Chemistry and Physics of Carbon, vol. 21. New York, NY: Marcel Dekker.
- Uguina, M.A., J.L. Sotelo, J.A. Delgado, J.M. Gómez, and L.I. Celemin. 2005. Adsorption of methyl ethyl ketone and trichloroethene from aqueous solutions onto silicalite fixed-bed adsorbers. Sep. Purif. Technol. 42:91–99. doi:10.1016/j.seppur.2004.06.007
- Wu, H.M., and J.H. You. 2007. Destruction of methyl ethyl ketone vapor by ozone on activated carbon. J. Chin. Inst. Chem. Eng. 38:117–124. doi:10.1016/j.jcice.2006.10.002
- Yamamoto, T., A. Endo, T. Ohmori, and M. Nakaiwa. 2004. Porous properties of carbon gel microspheres as adsorbents for gas separation. Carbon 42:1671–76. doi:10.1016/j.carbon.2004.02.021
- Zerbonia, R.A., C.M. Brockmann, and P.R. Peterson. 2001. Carbon bed fires and the use of carbon canisters for air emissions control on fixed-roof tanks. J. Air Waste Manag. Assoc. 51:1617–27. doi:10.1080/10473289.2001.10464393