ABSTRACT
Carbonaceous components (organic carbon [OC] and elemental carbon [EC]) and optical properties (light absorption and scattering) of fine particulate matter (aerodynamic diameter <2.5 μm; PM2.5) were simultaneously measured at an urban site in Gwangju, Korea, during the winter of 2011. OC was further classified into OC1, OC2, OC3, and OC4, based on a temperature protocol using a Sunset OC/EC analyzer. The average OC and EC concentrations were 5.0 ± 2.5 and 1.7 ± 0.9 μg C m−3, respectively. The average single-scattering albedo (SSA) at a wavelength of 550 nm was 0.58 ± 0.11, suggesting that the aerosols observed in the winter of 2011 had a local warming effect in this area. During the whole sampling period, “stagnant PM” and “long-range transport PM” events were identified. The light absorption coefficient (babs) was higher during the stagnant PM event than during the long-range transport PM event due to the existence of abundant light-absorbing OC during the stagnant PM event. In particular, the OC2 and OC3 concentrations were higher during the stagnant PM event than those during the long-range transport event, suggesting that OC2 and OC3 might be more related to the light-absorbing OC. The light scattering coefficient (bscat) was similar between the events. On average, the mass absorption efficiency attributed to EC (σEC) was 9.6 m2 g−1, whereas the efficiency attributed to OC (σOC) was 1.8 m2 g−1 at λ = 550 nm. Furthermore, the σEC is comparable among the PM event days, but the σOC for the stagnant PM event was significantly higher than that for the long-range transport PM event (1.7 vs. 0.5).
Implications: Optical and thermal properties of carbonaceous aerosol were measured at Gwangju, and carbonaceous aerosol concentration and optical property varied between “stagnant PM” and “long-range transport PM” events. More abundant light absorbing OC was observed during the stagnant PM event.
Introduction
Atmospheric aerosols play an important role in climate change by scattering and absorbing solar radiation (radiation balance), in addition to acting as cloud condensation nuclei (Intergovernmental Panel on Climate Change [IPCC], Citation2007). Aerosols can also enter into the human respiratory system and cause negative health effects (Dockery et al., Citation1993). Such aerosol effects depend on the chemical and physical properties of the particles. Carbonaceous aerosols, which are one of the major chemical components in atmospheric aerosols, can be classified as organic carbon (OC) and elemental carbon (EC) (Malm et al., Citation1994; Ramanathan et al., Citation2001a). EC is nonvolatile, thermally, and chemically stable species under atmospheric conditions (Petzold et al., Citation2013). Various thermal-optical methods have been used to determine the concentration of atmospheric EC (Chow et al., Citation2004). In the thermal-optical method, the EC content are directly measured through the thermal evolution/combustion, and laser reflectance or transmittance is used to determine OC/EC split. An optical method was used to determine equivalent black carbon (BC) concentration through the mass absorption efficiency from the measured light absorption/scattering properties. Atmospheric OC is highly chemically complex and includes hundreds or thousands of compounds with a wide range of molecular forms, volatility, and water solubility (Rogge et al., Citation1991; Jacobson et al., Citation2000; Sullivan et al., Citation2004, Citation2006; Favez et al., Citation2008; Bae and Schauer, Citation2009; Batmunkh et al., Citation2011). The atmospheric OC can be produced directly and indirectly from a wide range of sources, including fossil fuel combustion, industrial emissions, plant matter, biomass burning, and biogenic emissions (e.g., condensation of low-volatility oxidation products of volatile organic carbon) (Kanakidou et al., Citation2005; Seinfeld and Pandis, Citation2012). It has been reported that the atmospheric OC constitutes 20~90% of the total fine particulate mass with significant spatial and temporal variations (Kanakidou et al., Citation2005; Zhang et al., Citation2007; Batmunkh et al., Citation2013).
Thermal-optical methods have been used to measure carbon fractions (OC and EC) in suspended particles collected on filters (Chow et al., Citation2004). The thermally derived OC fractions (e.g., OC1, OC2, OC3, or OC4) at different evolution temperatures have been useful for the estimation of OC types and their sources (Chow et al., Citation2005; Park et al., Citation2008; Miyazaki et al., Citation2007; Jung and Kawamura, Citation2011). Miyazaki et al. (Citation2007) reported that the OC1 was low refractory and more volatile organic compounds, which was thermally evolved from the beginning of analysis until 300 °C, whereas the OC4, which was evolved at 600–870 °C, was highly refractory compounds. Jung and Kawamura (Citation2011) found that the OC2 (450–600 °C) was the most dominant among OC fractions at background site (Gosan, Korea).
It is well known that EC strongly absorbs light from over the entire solar spectrum (i.e., 300–2500 nm) (Horvath Citation1993; Ramanathan et al., Citation2001b; Bergstrom et al., Citation2002; Arnott et al., Citation2005; Bond and Bergstrom, Citation2006; Ramanathan and Carmichael, Citation2008; Chow et al., Citation2009). The mass absorption efficiency (σ) or mass-specific absorption cross-section of fresh soot at a light wavelength (λ) of 550 nm is reported to be 7.5 ± 1.2 m2 g−1 (Bond and Bergstrom, Citation2006). The mass absorption efficiency depends on chemical composition (Ram et al., Citation2010), measurement protocol (Chow et al., Citation2009), meteorological condition (Lack et al., Citation2009), and mixing state or aging of aerosols (Bond et al., Citation2007). Weingartner et al. (Citation2003), Schnaiter et al. (Citation2003), and Kirchstetter et al. (Citation2004) demonstrated that the light absorption of particles produced from high-temperature combustion processes (e.g., diesel soot) has low spectral dependence (approximately λ−1), whereas particles emitted from low-temperature combustion processes (e.g., biomass burning) has strong spectral dependence with a low light absorption coefficient.
On the other hand, it has been reported that OC contributes predominantly to the scattering of incident solar radiation (Gelencsér, Citation2004), and that a complex refractive index for OC was assumed to be a value close to that of sulfate aerosols (scattering aerosols) (Liousse et al., Citation1996). However, its absorbing components were recently identified. Although BC absorbs light strongly over the 300–2500 nm spectrum, some OC (e.g., yellowish/brownish carbon) absorbs light efficiently in the ultraviolet (UV) and blue regions (Jacobson and Mark, 1999; Bond, Citation2001; Andreae and Gelencsér, Citation2006; Roden et al., Citation2006; Bergstrom et al., Citation2007; Sun et al., Citation2007). It has been reported that OC displaying light-absorbing behavior can be produced by solid-fuel burning (e.g., coal combustion) (Bond, Citation2001) and biomass burning (Kirchstetter et al., Citation2004; Roden et al., Citation2006). Sun et al. (Citation2007) demonstrated that light-absorbing OC includes oxygenated and multifunctional groups with high molecular weights. Thus, the simultaneous measurement of OC, EC, and the optical properties (light scattering and light absorption) of the particles is essential for better understanding of the effects of carbonaceous aerosols on climate change. Those data with the classification of OC subgroups are lacking, especially over downwind regions of the East Asian continent.
In this study, an intensive measurement of carbonaceous aerosols and their optical properties was conducted in Gwangju, Korea (downwind region of the East Asian continent), in the winter of 2011. OC and EC concentrations, including OC fractions (OC1, OC2, OC3, and OC4), were measured using a Sunset OC/EC analyzer (model 3F; Sunset Laboratory Inc., Tigard, OR, USA) with a thermal-optical transmittance (TOT) protocol (Birch and Cary, Citation1996). Simultaneously, the light absorption and light scattering of aerosols were measured with an aethalometer (AE-31; Magee Scientific, Berkeley, CA, USA) and nephelometer (NGN-3A; Optec, Lowell, MI, USA), respectively, to examine the relationship between the concentrations of EC and OC and optical characteristics. The single-scattering albedo (SSA) and absorption Ångström exponent (AAE), which are important optical parameters for determining the effect of aerosols on radiation balance, were also determined. In addition, specific particulate matter (PM) events (e.g., long-range transport event) were defined based on the mass concentration of particulate matter less than 2.5 µm (PM2.5) and air mass backward-trajectory data.
Methods
An intensive measurement was conducted from November 22 to December 20, 2011, at an urban site in Gwangju, Korea. The sampling site (35.13°N, 126.50°E) is affected by a residential/commercial area (~0.6 km away from the site), a highway (~1.5 km away from the site), a small industrial area (~1.5 km away from the site), and an agricultural area (~0.8 km away from the site). Gwangju is the sixth largest city in Korea and has a population of ~1,500,000. A major industrial area (the largest petrochemical industrial complex and steel works in Korea) is located ~90 km southeast from the site. In addition, the site can be affected by long-range-transported air masses originating from China and passing over the major industrial area (northwest from the site) (Park et al., Citation2012).
OC and EC concentrations in PM2.5 were measured using a semicontinuous Sunset OC/EC analyzer with a TOT method (Birch and Cary, Citation1996). The ambient air was drawn at a flow rate of 8 liters per minute (lpm) through a 2.5-μm cut-point cyclone inlet and passed through a multichannel parallel-plate denuder with a carbon-impregnated filter to remove semivolatile organic vapors that could be potentially adsorbed onto the quartz-filter media (Turpin et al., Citation2000). OC and EC were analyzed under prescribed temperature protocols in an inert and oxidizing atmosphere. The sampling time was 44 min, and the analysis time was 12 min for one measurement run. The sample-loaded filter was heated to evaporate OC and was subsequently cooled down to 550 °C for 5 sec in a nonoxidizing atmosphere (i.e., He atmosphere). OC concentrations detected at analysis temperatures of 395 °C (90 sec), 650 °C (55 sec), 775 °C (60 sec), and 800 °C (55 sec) were assigned to OC1, OC2, OC3, and OC4, respectively. Then, the carrier gas was switched to 10% O2 in He, and the temperature was stepped up to 840 °C to combust EC as well as pyrolyzed OC. EC concentration was determined at temperatures of 650 °C (45 sec) and 840 °C (120 sec). The analyzer was automatically calibrated at the end of every analysis cycle by injecting an internal standard CH4 mixture (5% CH4 in He) via a fixed injection-volume carbon loop to compensate for the variability in the instruments’ nondispersive infrared (NDIR) sensor responses.
The accumulation of refractory metal oxides on the filter can cause a substantial decrease in the laser signal (Park et al., Citation2005). The metal oxides can act as oxidants, causing the EC to evolve in the inert atmosphere. This leads to underestimation of EC and overestimation of OC (Chow et al., Citation2001). Polidori et al. (Citation2006) suggested that the highest OC temperature in inert mode should be reduced to 700°C, which could mitigate such effect caused by the refractory metal oxides. To minimize the effect of refractory metal oxides, the filter was changed when laser correction was lower than 0.91, and the highest temperature in the inert atmosphere was selected as 800 °C for this study. The laser correction factor is used as an indicator for the aging of a filter media and accumulation of refractory materials (e.g., iron oxide) on the filter (laser transmittance of light from a diode laser is used to optically correct pyrolysis/charring carbon formed from organic compounds). Detection limits of OC1, OC2, OC3, OC4, and EC were found to be 0.29, 0.18, 0.11, 0.09, and 0.20 μg C m–3, respectively, which were calculated as 3 times the standard deviation of a dynamic blank. The dynamic blank was obtained by sampling and analyzing particle-free ambient air.
An aethalometer was used to determine the light absorption at seven wavelengths (370, 470, 520, 590, 660, 880, and 950 nm) (Hansen et al., Citation1984). The flow rate was maintained at 3.9 lpm through a 2.5-μm cut-point cyclone. The aerosol was dried to relative humidity (RH) <40% by a diffusion dryer prior to particle detection because water uptake by aerosols can increase the light absorption up to 1.35 times at RH values ranging from 30% to 95% (Redemann et al., Citation2001). The detection limit of the BC concentration obtained from the light absorption was 0.22–0.31 μg C m−3 in the wavelength range of 370–950 nm, which was calculated as 3 times the standard deviation of the dynamic blank measurement.
The aerosol light absorption coefficient (babs) was determined by using previously described methods (Weingartner et al., Citation2003; Arnott et al., Citation2005; Schmid et al., Citation2006), accounting for multiple scattering and shadowing effects (Posfai et al., Citation1999; Naoe and Okada, Citation2001; Weingartner et al., Citation2003; Arnott et al., Citation2005; Schmid et al., Citation2006). The light attenuation coefficient (σATN) measured with the aethalometer can be converted to the light absorption coefficient (babs) by correcting effects caused by multiple scattering and filter loading with eq 1.
where the C is a multiple scattering factor that corrects the filter media itself (the value for the C suggested by Schmid et al. [Citation2006] was used here), and the RATN is a shadowing factor which accounts for the “shadowing” effect due to filter loading. The RATN is defined as in eq 2 (Weingartner et al., Citation2003).
where the ATN is the amount of light attenuation and the f is a loading factor calculated by eq 3.
where the BC is black carbon concentration and the i refers to the number of measurements.
The absorption Ångström exponent (AAE) was determined by using the change in light absorption as a function of wavelength (i.e., 370, 470, 520, 590, 660, 880, and 950 nm). The light scattering coefficient (bscat at 550 nm) of particles was measured with a nephelometer. A zero-air calibration was performed every 12 hr during the intensive measurement period. With a time resolution of 1 min, the detection limit of bscat was determined to be 4.4 Mm−1, which was calculated as 3 times the standard deviation of the dynamic blank measurement. The single-scattering albedo (SSA) was determined using the ratio of bscat to the aerosol extinction coefficient (bext), which was calculated as the sum of bscat and babs. Because the wavelengths of the measured bscat and babs were not the same, a correction was made (i.e., babs ~ (λ)−AAE). The mass absorption efficiency (σabs) and mass scattering efficiency (σscat) for the PM2.5 were determined using the ratio of the absorption and scattering coefficients to the PM2.5 mass concentration, respectively.
The PM2.5 mass concentration was measured with a time resolution of 10 min by an aerosol spectrometer (model 265; Grimm Aerosol Technik GmbH, Ainring, BY, Germany), which determines the concentration of aerosols by quantifying the scattering of particles passing through a light beam (a laser diode). The aerosol spectrometer system consists of an aerosol spectrometer and a dry air dilution system combined with a heated sampling line. The concentrations of CO were measured continuously (5-min interval) using an NDIR instrument (48C; Thermo, Waltham, MA, USA) at flow rate of 1.5 lpm. A zero-air calibration was performed twice a day. The initial and periodic multipoint calibrations of the CO trace gas analyses were performed during the intensive measurement period. One-hour average data for light absorption, light scattering, PM2.5, and CO were used to be compared with OC and EC data.
A 4-day back-trajectory analysis was conducted using the Hybrid Single-Particle Lagrangian Integrated Trajectory (HYSPLIT) model (Draxler and Rolph, Citation2013) (http://ready.arl.noaa.gov/HYSPLIT.php) to find the origin and transport of air masses arriving at 50 m above ground level (AGL) of the sampling site. The aerosol optical depth (AOD) was retrieved from Moderate Resolution Imaging Spectroradiometer (MODIS) satellite data using the modified Bremen AErosol Retrieval (MBAER) algorithm (Lee et al., Citation2007) to identify air pollutant plumes.
Results and discussion
OC and EC concentrations and OC/EC ratios
The average OC and EC concentrations at the urban site in Gwangju, Korea, during the sampling period and their comparison with other sampling sites in East Asia are summarized in . The average OC concentration is 5.0 ± 2.5 μg C m−3, which is comparable to the value in Tokyo (5.0 μg C m−3), higher than concentrations measured at background site (0.8 μg C m−3 in Rishiri), and lower than those measured in mega cities (Seoul and Beijing) and city Ulaanbaatar (see ). The average EC concentration is 1.7 ± 0.9 μg C m−3, with an OC/EC ratio of 3. The concentration of EC is more closely related to the population size of cities (r = 0.89) than the OC concentration (not shown here). The fraction of OC in PM2.5 is 17%, whereas that of EC is 4%.
Table 1. Concentrations of OC and EC and the ratio of OC to EC at sampling sites in East Asia.
The OC/EC ratio is useful to infer primary and secondary OC. Turpin and Huntzicker (Citation1991) reported that when the OC/EC value is lower than 2.2, the OC can come from primary combustion sources, which also emit the EC. The OC/EC value (~3) at Gwangju suggests that a significant amount of OC was produced by secondary process (Huang et al., Citation2012). Also, the biomass burning aerosols can also contribute to the high OC/EC ratio (Zeng and Wang, Citation2011). The high OC concentration (26.5 μg C m−3) and OC/EC ratio (17.7) at Ulaanbaatar, Mongolia, might be caused by strong coal combustion and biomass burning for heating in the winter season.
Light absorption and scattering coefficients, MAE, SSA, and AAE
shows the time series of hourly and daily PM2.5, hourly OC (OC1, OC2, and OC3), EC, CO, babs, and bscat, and meteorological data, including wind speed, wind direction, and precipitation. Missing data on December 1, 2012, were due to continuous rain. During days with precipitation and/or strong wind, the PM2.5 concentration was generally low. Based on the PM2.5 mass concentration, a PM event is defined as when the daily PM2.5 mass concentration exceeded the 1-month average value by 1 standard deviation (i.e., >53 µg m−3). Three PM events (November 29, December 11, and December 12 of 2011) during the whole sampling period were identified. The average concentrations of PM2.5, OC (OC1, OC2, and OC3), and EC and babs and bscat in the whole sampling period and on PM event days are summarized in . The OC2 was found to be most dominant in the total OC (52% of the total OC), with a mean of 2.6 ± 1.5 μg C m−3, whereas the OC1 contributed 40% to the total OC. However, the OC3 concentration was significantly lower (0.6 ± 0.3 μg C m−3) relative to OC1 and OC2 and contributed 12% to the total OC. The OC4 concentration was close to the detection limit of the OC/EC analyzer (~0.1 ± 0.1 μg C m−3) and was not included in our analysis.
Figure 1. Time series of hourly and daily PM2.5 and hourly OC1, OC2, OC3, EC, CO, bscat, babs, and meteorological parameters such as wind speed, direction, and precipitation (rainfall rate [RR] indicates the amount of rain water in mm per hour precipitation) in the winter of 2011.
![Figure 1. Time series of hourly and daily PM2.5 and hourly OC1, OC2, OC3, EC, CO, bscat, babs, and meteorological parameters such as wind speed, direction, and precipitation (rainfall rate [RR] indicates the amount of rain water in mm per hour precipitation) in the winter of 2011.](/cms/asset/c5d3fe17-8153-4645-bb4c-e8844c67d43e/uawm_a_1101031_f0001_b.gif)
Table 2. Concentrations of PM2.5, OC1, OC2, OC3, and EC and optical properties (babs and bscat) during PM events and of different air masses.
Diurnal variations in CO, PM2.5, OC, and EC, optical properties (bscat, babs, and SSA), planetary boundary layer (PBL) depth, and meteorological parameters, including wind speed and temperature, are shown in , which were averaged during the whole sampling period. A distinct diurnal pattern was observed for OC1, OC2, EC, and CO (high in the morning and evening and low in the afternoon). This pattern occurred because local combustion sources, such as traffic emissions and residential heating, were intense in the morning and evening and because the weakening of the combustion source strength and the enhanced mixing layer (i.e., the higher PBL) in the afternoon caused their concentrations to decrease (i.e., more dilution). The babs varied between 12.2 and 30.8 Mm−1, with an elevated value in the morning and evening, and the bscat varied between 18.9 and 39.1 Mm−1 and had a similar diurnal pattern to the babs. The average SSA (the ratio of bscat to the aerosol extinction coefficient [bext]) at 550 nm, which is a useful parameter for the determination of aerosol effects on the radiation balance of the Earth, was 0.58 ± 0.11 for the whole sampling period. Hansen et al. (Citation1997) suggested that the threshold value for discrimination between cooling and warming effects of aerosols should be 0.85. The SSA value of this study was much lower than the threshold value, suggesting that the aerosols observed in the winter of 2011 had a local warming effect in this area. The SSA was found to be lowest (~0.45) in the morning (08:00), which corresponded to the highest babs. The SSA increased up to 0.57 at 3:00 p.m. due to decreasing light-absorbing aerosol source strength, increasing mixing height, and increasing secondary aerosol formation (i.e., scattering aerosols) in the afternoon. The evening SSA peak was maintained until ~5:00 a.m. on the following day because of the stable and shallow boundary layer.
Figure 2. Diurnal variations of CO, PM2.5, carbonaceous aerosols (OC1, C2, OC3, and EC), aerosol optical properties (bscat, babs, and SSA), planetary boundary layer (PBL) depth, and meteorological parameters, including temperature and wind speed, calculated during the whole observation period in the winter of 2011.
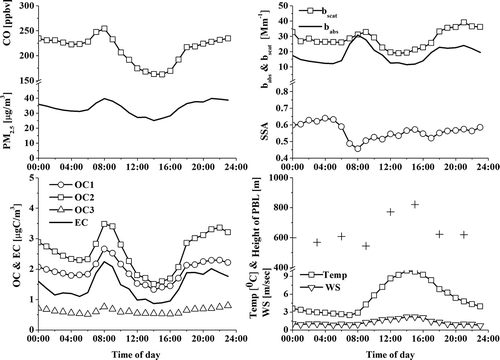
In order to investigate the contribution of EC and OC to the total light absorption, a multiple linear regression analysis was conducted. The multiple linear regression (see Supplemental Material) attempts to model the relationship between two or more explanatory variables and a response variable by fitting a linear equation to observed data. It was reported that OC can also increase the total light absorption by approximately 10–50% (Kirchsteter et al., 2004; Andreae et al., Citation2008). As the occurrence of Asian dust was not observed during the sampling period, the light absorption caused by mineral dust was not considered in this study. As shown in , the average mass absorption efficiency attributed to EC (σEC) was 9.6 m2 g−1 at 550 nm, which is higher than that attributed to fresh soot particles (7.5 m2 g−1) (Bond and Bergstrom, Citation2006). This result reveals that EC particles can be likely more aged than fresh soot particles. The σEC is comparable to that found in Seoul, Korea, in the winter (10.3 m2 g−1 at λ = 550 nm) (Jung et al., Citation2010); in Xianghe, China, in the spring (10.0 m2 g−1 at λ = 520 nm) (Yang et al., Citation2009); in Kanpur, India, from October 2008 to April 2009 (9.6 m2 g−1 at λ = 670 nm) (Ram et al., Citation2012); and in Fresno, California, USA, from August to September 2005 (9.3 m2 g−1 at λ = 532 nm) (Chow et al., Citation2009). The average mass absorption efficiency attributed to OC (σOC) was 1.8 m2 g−1 at 550 nm, which is higher than that found in Xianghe, China, in spring (0.63 m2 g−1 at λ = 520 nm) (Yang et al., Citation2009) and in Guangzhou, China, in fall (0.76 m2 g−1 at λ = 540 nm) (Andreae et al., Citation2008).
Table 3. Mass absorption efficiency (m2 g−1) attributed to EC (σEC), OC (σOC), and PM2.5 (σabs) at λ = 550 nm.
Characteristics of aerosols according to PM events and air masses
As discussed earlier, three PM events during the whole sampling period were identified. According to the air mass backward-trajectory and AOD data, the PM events were classified as (a) “stagnant PM event” and (b) “long-range transport PM event.” In the stagnant PM event on November 29, 2011, the air mass was stagnant around the Korean peninsula (within 800 km from the sampling site, as shown in ) where the average wind speed was as low as 0.72 msec−1, which may cause the accumulation of local and regional air pollutants and enhance PM2.5 mass concentration (defined here as the “stagnant PM event”). On the other hand, in the long-range transport PM event on December 12, 2011, the air mass originated from northern China and Mongolia and passed over northeastern China (strong anthropogenic sources exist), as shown in . The observation of high AOD in northeastern China as shown in supports the occurrence of strong aerosol emissions in the industrial region. A similar observation was also found on December 11, 2011. On these days, the long-range-transported aerosols could influence the enhancement of the PM2.5 mass concentration in addition to locally produced aerosols in Korea (defined here as the “long-range transport PM event”). To evaluate the long-range transport PM event days, we also obtained PM10 concentrations from the 20 network sites that are operated by the Ministry of Environment in Korea on December 9, 2011, December 12, 2011, and December 16, 2011, and these data are shown in Figure S1 (Supplemental Material). Data clearly indicate that the PM10 concentrations simultaneously increased during the PM event day (December 12, 2011) over the southwest part of Korea. The PM10 concentrations on that day were more than 2 times greater than the concentrations found on other days. Figure S1 also includes a three-dimensional back-trajectory matrix of the air mass. These results also support that the southwest region of Korea was affected by a long-range-transported air mass from the western area of China.
Figure 3. MODIS AOD image and 4-day air mass backward trajectories on (a) November 29, 2011 (“stagnant PM event”) and (b) December 12, 2011 (“long-range transport PM event”).
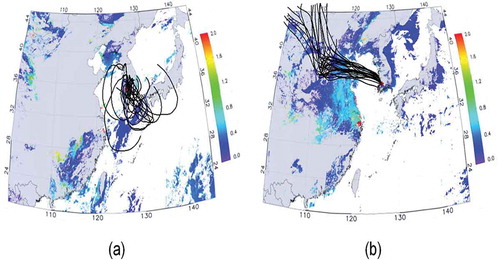
For the PM event days, the average mass concentrations of PM2.5 and carbonaceous aerosols, babs, and bscat were higher than the overall average values, as shown in . In particular, the babs for the stagnant PM event increased by 62–78% compared with the overall average value. The higher babs for the PM events can be attributed to the light-absorbing OC rather than EC (the most light-absorbing materials). Note that EC concentration was comparable among days. The babs for the stagnant PM event was much higher (31–52%) than that for the long-range transport PM event. The higher babs for the stagnant PM event rather than the long-range transport PM event can also be attributed to the light-absorbing OC. The concentrations of EC in the stagnant and long-range transport PM events were 1.9 and 1.7 μg C m−3, respectively, and OC concentration in the stagnant PM event was higher than that in the long-range transport PM event. In particular, the OC2 and OC3 values were higher during the stagnant PM event than those for the long-range transport event, suggesting that OC2 and OC3 might be more related to the light-absorbing OC. The dependence of babs on wavelengths on different PM event days and nonevent days will be discussed in the later section. The bscat was not much different between the stagnant and long-range transport PM events (bscat for stagnant and long-range transport PM events is 49.4 and 48.4 Mm−1 [inverse megameters], respectively).
The σEC or σOC at 550 nm was also compared between different PM event days and nonevent days, as shown in . The σEC is comparable among the PM event days; however, the σOC during the long-range PM event was much lower than those in nonevent and the stagnant PM event, suggesting that OC observed during the long-range PM event contributed significantly less to the light absorption than the OC observed during the stagnant PM event or nonevent.
The spectral dependence of babs on different PM event days and nonevent days was examined, as shown in . The AAE provides the change in light absorption as a function of wavelength, which is calculated using a power-law relationship from the spectral dependence of babs. The AAE of graphite carbon or EC from fossil fuel combustion is close to 1, whereas biomass-burning OC and dust can lead to the higher AAE (>1) (Kirchstetter et al., Citation2004). Also, the mixture of OC and EC (e.g., EC core coated by nonabsorbing species) can also lead to the higher AAE (>1) (Lack and Cappa, Citation2010). Thus, the AAE is useful to infer chemical composition. The scattering Ångström exponent (SAE), which can be calculated using a power-law relationship from the spectral dependence of bscat (this is not available in this study), can provide useful information on particle size (e.g., the higher SAE, the smaller particle size) (Cazorla et al., Citation2013). The AAE of 1.42 was found during the stagnant PM event, suggesting that the mixture of OC and EC contributed to the light absorption. The dust contribution to the light absorption would be small because no dust events happened during the current sampling period and the dust fraction in PM2.5 would not be so large. Somewhat higher AAE (1.58) was found during the long-range PM event than that (1.42) in the stagnant event.
Figure 4. Comparison of spectral dependence of babs among PM events: Line, dot, and dash represent the stagnant PM event, long-range transport PM event, and nonevent, respectively.
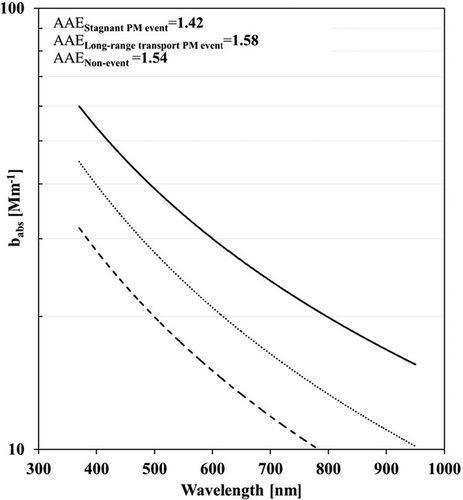
The effects of air mass on the PM2.5, OC, EC, and babs and bscat were also investigated. The air mass was classified as a northeastern air mass or northwestern air mass during the whole sampling period (see ). The northwestern air mass was often observed, for 22 days out of 26 days, and the northeast air mass was observed for 4 days. As shown in , the average mass concentration of PM2.5 for the northwestern air mass was higher than that observed with the northeastern air mass (34.2 ± 17.5 vs. 17.1 ± 7.2). Northwestern air masses are typically encountered from industrial areas in China. The concentrations of carbonaceous aerosols as well as babs and bscat with the northwestern air mass were 1.2–2.0 times higher than those measured with the northeast air mass, suggesting that the air mass also played an important role in the enhanced concentrations of PM2.5 and carbonaceous species at the sampling site.
Conclusions
In this study, the mass concentrations of OC and EC and the optical properties of PM2.5 were determined by conducting time-resolved intensive measurements at an urban site in Gwangju, Korea, from November 22 to December 20, 2011. Both locally produced aerosols as well as long-range-transported aerosols influenced the enhanced PM concentration at the sampling site. OC, including OC1, OC2, OC3, and OC4; EC concentrations; and optical properties varied with the different PM events (stagnant PM event and long-rang transport PM event) and air masses. The babs was higher during the stagnant PM event than during the long-range transport PM event because of the abundant concentration of light-absorbing OC in the stagnant PM event. The OC2 and OC3 were much higher than during the stagnant PM event than during the long-range transport event, suggesting that OC2 and OC3 might be more closely connected to the light-absorbing OC. The bscat was similar between the events. On average, the σEC at 550 nm was approximately 5 times higher than the σOC. Furthermore, the σEC values were comparable among the PM event days, whereas the σOC in the stagnant PM event was significantly higher than in the long-range transport PM event, suggesting that OC observed during the stagnant PM event contributed significantly more to the light absorption than OC observed for the long-range PM event. The 4-day backward air trajectory analysis indicated that the northwestern air mass was observed more frequently than the northeastern air mass during the sampling period. The average PM2.5 with the northwestern air mass (typically encountered from industrial areas in China) was approximately 2 times higher than that observed with the northeastern air mass. Similarly, the concentrations of carbonaceous aerosols, as well as babs and bscat, with the northwestern air mass were 1.2–2.0 times higher than those measured with the northeastern air mass. These results indicate that the air mass greatly influenced the enhancement of the concentration of PM2.5 and carbonaceous aerosols at the sampling site. Our data suggest that different types of OC in terms of their thermal (varying OC1, OC2, and OC3 fractions) and optical properties (varying light absorption) were produced, depending on the types of PM events (stagnant PM event versus long-range transport PM event) and air masses.
Acknowledgment
Mr. Kwan Chul Kim is acknowledged for the retrieval of aerosol optical depth (AOD) images.
Funding
The research was supported by the National Leading Research Laboratory program (NRF-2011-0015548), and the PM2.5 Research Center (NRF-2014 M3C8A5028593) was supported by the Ministry of Science, ICT, and Future Planning (MSIP) and the National Research Foundation (NRF) of Korea.
Supplemental Material
Supplemental data for this article can be accessed on the publisher’s website.
Supplemental Material
Download Zip (886.8 KB)Additional information
Funding
Notes on contributors
Tsatsral Batmunkh
Tsatsral Batmunkh is a postdoctoral fellow at the School of Environmental Science and Engineering in Gwangju Institute of Science and Technology (GIST), Gwangju, Republic of Korea and senior researcher at the Research Institute of Meteorology, Hydrology and Environment, Ulaanbaatar, Mongolia.
KwangYul Lee
KwangYul Lee is a Ph.D. candidate at the Gwangju Institute of Science and Technology (GIST), Gwangju, Republic of Korea.
Young J. Kim
Young J. Kim is a professor at the School of Environmental Science and Engineering in Gwangju Institute of Science and Technology (GIST), Gwangju, Republic of Korea.
Min-Suk Bae
Min-Suk Bae is a professor at the Department of Environmental Engineering, Mokpo National University, Muan-gun, Republic of Korea.
Shila Maskey
Shila Maskey is a postdoctoral fellow at the School of Environmental Science and Engineering in Gwangju Institute of Science and Technology (GIST), Gwangju, Republic of Korea.
Kihong Park
Kihong Park is a professor at the School of Environmental Science and Engineering and director of PM2.5 research center in Gwangju Institute of Science and Technology (GIST), Gwangju, Republic of Korea.
References
- Andreae, M., and A. Gelencsér. 2006. Black carbon or brown carbon? The nature of light-absorbing carbonaceous aerosols. Atmos. Chem. Phys. 6:3131–3148. doi:10.5194/acp-6-3131-2006
- Andreae, M.O., O. Schmid, H. Yang, D. Chand, J.Z. Yu, L.-M. Zeng, and Y.H. Zhang. 2008. Optical properties and chemical composition of the atmospheric aerosol in urban Guangzhou, China. Atmos. Environ. 42:6335–6350. doi:10.1016/j.atmosenv.2008.01.030
- Arnott, W.P., K. Hamasha, H. Moosmüller, P.J. Sheridan, and J.A. Ogren. 2005. Towards aerosol light-absorption measurements with a 7-wavelength aethalometer: Evaluation with a photoacoustic instrument and 3-wavelength nephelometer. Aerosol Sci. Technol. 39:17–29. doi:10.1080/027868290901972
- Bae, M.-S., and J.J. Schauer. 2009. Analysis of organic molecular markers in atmospheric fine particulate matter: Understanding the impact of “unknown” point sources on chemical mass balance models. J. Korean Soc. Atmos. Environ. 25:219–236. doi:10.5572/KOSAE.2009.25.3.219
- Batmunkh, T., Y. Kim, K. Lee, M. Cayetano, J. Jung, S. Kim, K. Kim, S. Lee, J. Kim, and L. Chang. 2011. Time-resolved measurements of PM2.5 carbonaceous aerosols at Gosan, Korea. J. Air Waste Manage. Assoc. 61:1174–1182. doi:10.1080/10473289.2011.609761
- Batmunkh, T., Y.J. Kim, J.S. Jung, K. Park, and B. Tumendemberel. 2013. Chemical characteristics of fine particulate matters measured during severe winter haze events in Ulaanbaatar, Mongolia. J. Air Waste Manage. Assoc. 63:659–670. doi:10.1080/10962247.2013.776997
- Bergstrom, R.W., P. Pilewskie, P. Russell, J. Redemann, T. Bond, P. Quinn, and B. Sierau. 2007. Spectral absorption properties of atmospheric aerosols. Atmos. Chem. Phys. 7:5937–5943. doi:10.5194/acp-7-5937-2007
- Bergstrom, R.W., P.B. Russell, and P. Hignett. 2002. Wavelength dependence of the absorption of black carbon particles: Predictions and results from the TARFOX experiment and implications for the aerosol single scattering albedo. J. Atmos. Sci. 59:567–577. doi:10.1175/1520-0469(2002)059<0567: WDOTAO>2.0.CO;2
- Birch, M., and R. Cary. 1996. Elemental carbon-based method for monitoring occupational exposures to particulate diesel exhaust. Aerosol Sci. Technol. 25:221–241. doi:10.1080/02786829608965393
- Bond, T.C. 2001. Spectral dependence of visible light absorption by carbonaceous particles emitted from coal combustion. Geophys. Res. Lett. 28:4075–4078. doi:10.1029/2001GL013652
- Bond, T.C., and R.W. Bergstrom. 2006. Light absorption by carbonaceous particles: An investigative review. Aerosol Sci. Technol. 40:27–67. doi:10.1080/02786820500421521
- Bond, T.C., E. Bhardwaj, R. Dong, R. Jogani, S. Jung, C. Roden, D.G. Streets, and N.M. Trautmann. 2007. Historical emissions of black and organic carbon aerosol from energy-related combustion, 1850–2000. Glob. Biogeochem. Cycles 21:GB2018.
- Cazorla, A., R. Bahadur, K. Suski, J.F. Cahill, D. Chand, B. Schmid, V. Ramanathan, and K. Prather. 2013. Relating aerosol absorption due to soot, organic carbon, and dust to emission sources determined from in-situ chemical measurements. Atmos. Chem. Phys. 13:9337–9350. doi:10.5194/acp-13-9337-2013
- Cheng, Y., K. He, F. Duan, M. Zheng, Y. Ma, and J. Tan. 2009. Positive sampling artifact of carbonaceous aerosols and its influence on the thermal-optical split of OC/EC. Atmos. Chem. Phys. 9:7243–7256. doi:10.5194/acp-9-7243-2009
- Cheng, Z., J. Jiang, C. Chen, J. Gao, S. Wang, J. Watson G.; Wang, H.; Deng, J.; Wang, B.; and Zhou, M.. 2014. Estimation of aerosol mass scattering efficiencies under high mass loading: Case study for the megacity of Shanghai, China. Environ. Sci. Technol. 49:831–838. doi:10.1021/es504567q
- Chow, J., J. Watson, L.-W. Chen, G. Paredes-Miranda, M.-C. Chang, D. Trimble, K. Fung, H. Zhang, and Zhen Yu, J.. 2005. Refining temperature measures in thermal/optical carbon analysis. Atmos. Chem. Phys. 5:2961–2972. doi:10.5194/acp-5-2961-2005
- Chow, J.C., J.G. Watson, L.-W. A. Chen, W.P. Arnott, H. Moosmüller, and K. Fung. 2004. Equivalence of elemental carbon by thermal/optical reflectance and transmittance with different temperature protocols. Environ. Sci. Technol. 38:4414–4422. doi:10.1021/es034936u
- Chow, J.C., J.G. Watson, D. Crow, D.H. Lowenthal, and T. Merrifield. 2001. Comparison of IMPROVE and NIOSH carbon measurements. Aerosol Sci. Technol. 34:23–34. doi:10.1080/02786820119073
- Chow, J.C., J.G. Watson, P. Doraiswamy, L.-W. A. Chen, D.A. Sodeman, D.H. Lowenthal, K. Park, W.P. Arnott, and N. Motallebi. 2009. Aerosol light absorption, black carbon, and elemental carbon at the Fresno Supersite, California. Atmos. Res. 93:874–887. doi:10.1016/j.atmosres.2009.04.010
- Dockery, D.W., C.A. Pope, X. Xu, J.D. Spengler, J.H. Ware, M.E. Fay, B.G. Ferris Jr., and F.E. Speizer. 1993. An association between air pollution and mortality in six US cities. N. Engl. J. Med. 329:1753–1759. doi:10.1056/NEJM199312093292401
- Draxler, R.R., and G.D. Rolph. 2013. HYSPLIT (HYbrid Single-Particle Lagrangian Integrated Trajectory)., Silver Spring, MD: NOAA Air Resources Laboratory. Model access via NOAA ARL READY Web site. http://ready.arl.noaa.gov/HYSPLIT.php ( accessed in August 30, 2013).
- Favez, O., J. Sciare, H. Cachier, S.C. Alfaro, and M.M. Abdelwahab. 2008. Significant formation of water-insoluble secondary organic aerosols in semi-arid urban environment. Geophys. Res. Lett. 35:L15801. doi:10.1029/2008GL034446
- Gelencsér, A. 2004. Carbonaceous Aerosol. Atmospheric and Oceanographic Sciences Library, 30. Dordrecht, The Netherlands: Springer, 350.
- Han, S., Y. Kondo, N. Oshima, N. Takegawa, Y. Miyazaki, M. Hu, P. Lin, Z. Deng, Y. Zhao, and N. Sugimoto. 2004. Temporal variations of elemental carbon in Beijing. J. Geophys. Res. Atmos. 114(D23):D23202. doi:10.1029/2009JD012027
- Hansen, A., H. Rosen, and T. Novakov. 1984. The aethalometer—An instrument for the real-time measurement of optical absorption by aerosol particles. Sci. Total Environ. 36:191–196. doi:10.1016/0048-9697(84)90265-1
- Hansen, J., M. Sato, and R. Ruedy. 1997. Radiative forcing and climate response. J. Geophys. Res. 102:6831–6864. doi:10.1029/96JD03436
- Horvath, H. 1993. Atmospheric light absorption—A review. Atmos. Environ. A 27:293–317. doi:10.1016/0960-1686(93)90104-7
- Huang, H., K. Ho, S. Lee, P. Tsang, S. Ho S.H.; Zou, C.; Zou, S.; Cao, J., and H. Xu. 2012. Characteristics of carbonaceous aerosol in PM2.5: Pearl Delta River region, China. Atmos. Res. 104:227–236. doi:10.1016/j.atmosres.2011.10.016
- Intergovernmental Panel on Climate Change. 2007. Synthesis Report. Contribution of Working Groups I, II and III to the Fourth Assessment Report of the Intergovernmental Panel on Climate Change. Geneva, Switzerland: Intergovernmental Panel on Climate Change.
- Jacobson, M., H. Hansson, K. Noone, and R. Charlson. 2000. Organic atmospheric aerosols: Review and state of the science. Rev. Geophys. 38:267–294. doi:10.1029/1998RG000045
- Jacobson, M.Z. 1999. Isolating nitrated and aromatic aerosols and nitrated aromatic gases as sources of ultraviolet light absorption. J. Geophys. Res. 104(D3):3527–3542. doi:10.1029/1998JD100054
- Jung, J., and K. Kawamura. 2011. Springtime carbon emission episodes at the Gosan background site revealed by total carbon, stable carbon isotopic composition, and thermal characteristics of carbonaceous particles. Atmos. Chem. Phys. 11:10911–10928. doi:10.5194/acp-11-10911-2011
- Jung, J., Y. Kim, K. Lee, M. Cayetano, T. Batmunkh, J.-H. Koo, and J. Kim. 2010. Spectral optical properties of long-range transport Asian dust and pollution aerosols over Northeast Asia in 2007 and 2008. Atmos. Chem. Phys. 10:5391–5408. doi:10.5194/acp-10-5391-2010
- Jung, J., H. Lee, Y. J. Kim X. Liu, Y. Zhang, M. Hu, and N. Sugimoto. 2009. Optical properties of atmospheric aerosols obtained by in situ and remote measurements during 2006 Campaign of Air Quality Research in Beijing (CAREBeijing—2006). J. Geophys. Res. Atmos. 114(D2):D00G02. doi:10.1029/2008JD010337
- Kanakidou, M., J. Seinfeld, S. Pandis, I. Barnes, F. Dentener, M. Facchini, R. Dingenen V.; Ervens, B., A. Nenes, and C. Nielsen. 2005. Organic aerosol and global climate modelling: A review. Atmos. Chem. Phys. 5:1053–1123. doi:10.5194/acp-5-1053-2005
- Kim, H.-S., J.-B. Huh, P.K. Hopke, T.M. Holsen, and S.-M. Yi. 2007. Characteristics of the major chemical constituents of PM2.5 and smog events in Seoul, Korea in 2003 and 2004. Atmos. Environ. 41:6762–6770. doi:10.1016/j.atmosenv.2007.04.060
- Kirchstetter, T.W., T. Novakov, and P.V. Hobbs. 2004. Evidence that the spectral dependence of light absorption by aerosols is affected by organic carbon. J. Geophys. Res. Atmos. 109(D21):D21208. doi:10.1029/2004JD004999
- Lack, D., and C. Cappa. 2010. Impact of brown and clear carbon on light absorption enhancement, single scatter albedo and absorption wavelength dependence of black carbon. Atmos. Chem. Phys. 10:4207–4220. doi:10.5194/acp-10-4207-2010
- Lack, D. A., P.K. Quinn, P. Massoli, T.S. Bates, D. Coffman, D.S. Covert ; Sierau, B., S. Tucker, T. Baynard, and E. Lovejoy. 2009. Relative humidity dependence of light absorption by mineral dust after long-range atmospheric transport from the Sahara. Geophys. Res. Lett. 36:L24805. doi:10.1029/2009GL041002
- Lee, K. H., Y.J. Kim, W. von Hoyningen-Huene, and J.P. Burrow. 2007. Spatio-temporal variability of satellite-derived aerosol optical thickness over Northeast Asia in 2004. Atmos. Environ. 41:3959–3973. doi:10.1016/j.atmosenv.2007.01.048
- Lin, P., M. Hu, Z. Deng, J. Slanina, S. Han, Y. Kondo, N. Takegawa, Y. Miyazaki, Y. Zhao, and N. Sugimoto. 2009. Seasonal and diurnal variations of organic carbon in PM2.5 in Beijing and the estimation of secondary organic carbon. J. Geophys. Res. Atmos. 114(D2):D00G11. doi:10.1029/2008JD010902
- Liousse, C., H. Cachier, and S. Jennings. 1993. Optical and thermal measurements of black carbon aerosol content in different environments: Variation of the specific attenuation cross-section, sigma (σ). Atmos. Environ. A 27:1203–1211. doi:10.1016/0960-1686(93)90246-U
- Liousse, C., J. Penner, C. Chuang, J. Walton, H. Eddleman, and H. Cachier. 1996. A global three-dimensional model study of carbonaceous aerosols. J. Geophys. Res. Atmos. 101(D14):19411–19432. doi:10.1029/95JD03426
- Magi, B. 2009. Chemical apportionment of southern African aerosol mass and optical depth. Atmos. Chem. Phys. 9:7643–7655. doi:10.5194/acp-9-7643-2009
- Malm, W. C., J.F. Sisler, D. Huffman, R.A. Eldred, and T.A. Cahill. 1994. Spatial and seasonal trends in particle concentration and optical extinction in the United States. J. Geophys. Res. 99:1347–1347. doi:10.1029/93JD02916
- Matsumoto, K., Y. Uyama, T. Hayano, H. Tanimoto, I. Uno, and M. Uematsu. 2003. Chemical properties and outflow patterns of anthropogenic and dust particles on Rishiri Island during the Asian Pacific Regional Aerosol Characterization Experiment (ACE-Asia). J. Geophys. Res. Atmos. 108(D23):8666. doi:10.1029/2003JD003426
- Miyazaki, Y., Y. Kondo, S. Han, M. Koike, D. Kodama, Y. Komazaki, H. Tanimoto, and H. Matsueda. 2007. Chemical characteristics of water-soluble organic carbon in the Asian outflow. J. Geophys. Res. Atmos. 112(D22):D22S30. doi:10.1029/2006JD007125
- Miyazaki, Y., Y. Kondo, N. Takegawa, Y. Komazaki, M. Fukuda, K. Kawamura, M. Mochida, K. Okuzawa, and R. Weber. 2006. Time-resolved measurements of water-soluble organic carbon in Tokyo. J. Geophys. Res. Atmos. 111(D23):D23206. doi:10.1029/2006JD007125
- Naoe, H., and K. Okada. 2001. Mixing properties of submicrometer aerosol particles in the urban atmosphere—With regard to soot particles. Atmos. Environ. 35:5765–5772. doi:10.1016/S1352-2310(01)00367-3
- Park, K., J. Park, S. Lee, H.-j. Cho, and M. Kang. 2012. Real time measurement of chemical composition of submicrometer aerosols at urban Gwangju in Korea by aerosol mass spectrometer. Atmos. Environ. 62:281–290. doi:10.1016/j.atmosenv.2012.08.022
- Park, S. S., M.S. Bae, J.J. Schauer, S.Y. Ryu, Y.J. Kim S.Y.; Cho, and S.J. Kim. 2005. Evaluation of the TMO and TOT methods for OC and EC measurements and their characteristics in PM2.5 at an urban site of Korea during ACE-Asia. Atmos. Environ. 39:5101–5112. doi:10.1016/j.atmosenv.2005.05.016
- Park, S. S., K.-H. Lee, Y.J. Kim, T.Y. Kim, S.Y. Cho, and S.J. Kim. 2008. High time-resolution measurements of carbonaceous species in PM2.5 at an urban site of Korea. Atmos. Res. 89:48–61. doi:10.1016/j.atmosres.2007.12.002
- Petzold, A., J. Ogren, M. Fiebig, P. Laj, S.-M. Li, U. Baltensperger, T. Holzer-Popp, S. Kinne, G. Pappalardo, and N. Sugimoto. 2013. Recommendations for reporting “black carbon” measurements. Atmos. Chem. Phys. 13:8365–8379. doi:10.5194/acp-13-8365-2013
- Polidori, A., B.J. Turpin, H.-J. Lim, J.C. Cabada, R. Subramanian, S.N. Pandis, and A.L. Robinson. 2006. Local and regional secondary organic aerosol: Insights from a year of semi-continuous carbon measurements at Pittsburgh. Aerosol Sci. Technol. 40:861–872. doi:10.1080/02786820600754649
- Pósfai, M., J.R. Anderson, P.R. Buseck, and H. Sievering. 1999. Soot and sulfate aerosol particles in the remote marine troposphere. J. Geophys. Res. 104(D17):21685–21693. doi:10.1029/1999JD900208
- Ram, K., M. Sarin, and P. Hegde. 2010. Long-term record of aerosol optical properties and chemical composition from a high-altitude site (Manora Peak) in Central Himalaya. Atmos. Chem. Phys. 10:11791–11803. doi:10.5194/acp-10-11791-2010
- Ram, K., M. Sarin, and S. Tripathi. 2012. Temporal trends in atmospheric PM2. 5, PM10, elemental carbon, organic carbon, water-soluble organic carbon, and optical properties: Impact of biomass burning emissions in the Indo-Gangetic Plain. Environ. Sci. Technol. 46:686–695. doi:10.1021/es202857w
- Ramanathan, V., and G. Carmichael. 2008. Global and regional climate changes due to black carbon. Nat. Geosci. 1:221–227. doi:10.1038/ngeo156
- Ramanathan, V., P. Crutzen, J. Kiehl, and D. Rosenfeld. 2001. Aerosols, climate, and the hydrological cycle. Science 294:2119–2124. doi:10.1126/science.1064034
- Ramanathan, V., P.J. Crutzen, J. Lelieveld, A. Mitra, D. Althausen, J. Anderson, M. Andreae, W. Cantrell, G. Cass, and C. Chung. 2001. Indian Ocean Experiment: An integrated analysis of the climate forcing and effects of the great Indo-Asian haze. J. Geophys. Res. Atmos. 106(D22):28371–28398. doi:10.1029/2001JD900133
- Redemann, J., P.B. Russell, and P. Hamill. 2001. Dependence of aerosol light absorption and single-scattering albedo on ambient relative humidity for sulfate aerosols with black carbon cores. J. Geophys. Res. Atmos. 106(D21):27485–27495. doi:10.1029/2001JD900231
- Roden, C. A., T.C. Bond, S. Conway, and A. B. O. Pinel. 2006. Emission factors and real-time optical properties of particles emitted from traditional wood burning cookstoves. Environ. Sci. Technol. 40:6750–6757. doi:10.1021/es052080i
- Rogge, W. F., L.M. Hildemann, M.A. Mazurek, G.R. Cass, and B.R. Simoneit. 1991. Sources of fine organic aerosol. 1. Charbroilers and meat cooking operations. Environ. Sci. Technol. 25:1112–1125. doi:10.1021/es00018a015
- Schmid, O., P. Artaxo, W. Arnott, D. Chand, L. Gatti, G. Frank, A. Hoffer, M. Schnaiter, and M. Andreae. 2006. Spectral light absorption by ambient aerosols influenced by biomass burning in the Amazon Basin. I: Comparison and field calibration of absorption measurement techniques. Atmos. Chem. Phys. 6:3443–3462. doi:10.5194/acp-6-3443-2006
- Schnaiter, M., H. Horvath, O. Möhler, K.-H. Naumann, H. Saathoff, and O. Schöck. 2003. UV-VIS-NIR spectral optical properties of soot and soot-containing aerosols. J. Aerosol Sci. 34:1421–1444. doi:10.1016/S0021-8502(03)00361-6
- Seinfeld, J. H., and S.N. Pandis. 2012. Atmospheric Chemistry and Physics: From Air Pollution to Climate Change. New York: John Wiley & Sons.
- Sullivan, A., R.E. Peltier, C. Brock, J. De Gouw, J. Holloway, C. Warneke, A. Wollny, and R. Weber. 2006. Airborne measurements of carbonaceous aerosol soluble in water over northeastern United States: Method development and an investigation into water-soluble organic carbon sources. J. Geophys. Res. Atmos. 111(D23):D23S46. doi:10.1029/2006JD007072
- Sullivan, A., R. Weber, A. Clements, J. Turner, M. Bae, and J. Schauer. 2004. A method for on-line measurement of water-soluble organic carbon in ambient aerosol particles: Results from an urban site. Geophys. Res. Lett. 31:L13105. doi:10.1029/2004GL019681
- Sun, H., L. Biedermann, and T.C. Bond. 2007. Color of brown carbon: A model for ultraviolet and visible light absorption by organic carbon aerosol. Geophys. Res. Lett. 34:L17813. doi:10.1029/2007GL029797
- Tao, J., L. Zhang, K. Ho, R. Zhang, Z. Lin, Z. Zhang, M. Lin, J. Cao, S. Liu, and G. Wang. 2014. Impact of PM2.5 chemical compositions on aerosol light scattering in Guangzhou—The largest megacity in South China. Atmos. Res. 135:48–58.
- Turpin, B. J., and J.J. Huntzicker. 1991. Secondary formation of organic aerosol in the Los Angeles Basin: A descriptive analysis of organic and elemental carbon concentrations. Atmos. Environ. A 25:207–215. doi:10.1016/0960-1686(91)90291-E
- Turpin, B. J., P. Saxena, and E. Andrews. 2000. Measuring and simulating particulate organics in the atmosphere: Problems and prospects. Atmos. Environ. 34:2983–3013. doi:10.1016/S1352-2310(99)00501-4
- Wang, Y., S. Liu, P. Shi, Y. Li, C. Mu, and K. Du. 2013. Temporal variation of mass absorption efficiency of black carbon at urban and suburban locations. Aerosol Air Qual. Res. 13:275–286. doi:10.4209/aaqr.2012.05.0125
- Weingartner, E., H. Saathoff, M. Schnaiter, N. Streit, B. Bitnar, and U. Baltensperger. 2003. Absorption of light by soot particles: Determination of the absorption coefficient by means of aethalometers. J. Aerosol Sci. 34:1445–1463. doi:10.5194/acp-9-2035-2009
- Yang, M., S. Howell, J. Zhuang, and B. Huebert. 2009. Attribution of aerosol light absorption to black carbon, brown carbon, and dust in China–interpretations of atmospheric measurements during EAST-AIRE. Atmos. Chem. Phys. 9:2035–2050. doi:10.5194/acp-9-2035-2009
- Zeng, T., and Y. Wang. 2011. Nationwide summer peaks of OC/EC ratios in the contiguous United States. Atmos. Environ. 45:578–586. doi:10.5194/acp-5-2961-2005
- Zhang, Q., J. Jimenez, M. Canagaratna, J. Allan, H. Coe, I. Ulbrich, M. Alfarra, A. Takami, A. Middlebrook, and Y. Sun. 2007. Ubiquity and dominance of oxygenated species in organic aerosols in anthropogenically-influenced Northern Hemisphere midlatitudes. Geophys. Res. Lett. 34:L1380. doi:10.1029/2007GL029979