ABSTRACT
Biofiltration is a method of biological treatment belonging to cleaner technologies because it does not produce secondary air pollutants, but helps to integrate natural processes in microorganisms for decomposing volatile air pollutants and solving odor problems. The birch wood biochar has been chosen as a principal material for biofilter bed medium. The experiments were conducted at the temperatures of 24, 28, and 32 °C, while the concentration of acetone, xylene, and ammonium reached 300 mg/m3 and the flow rate was 100 m3/hr. Before passing through the stage of the experimental research into the packing material inside biofilters, microorganisms were introduced. Four strains of microorganisms (including micromycetes Aspergillus versicolor BF-4 and Cladosporium herbarum 7KA, as well as yeast Exophiala sp. BF1 and bacterium Bacillus subtilis B20) were selected. At the inlet loading rate of 120 g/m3/hr, the highest elimination capacity of xylene in the biochar-based biofilter with the inoculated medium was 103 g/m3/hr, whereas that of ammonia was 102 g/m3/hr and that of acetone was 97 g/m3/hr, respectively. The maximum removal efficiency reached 86%, 85%, and 81%, respectively. The temperature condition (though characterized by some rapid changes) can hardly have a considerable influence on the biological effect (i.e., microbiological activity) of biofiltration; however, it can cause the changes in physical properties (e.g., solubility) of the investigated compounds.
Implications: The birch biochar can be successfully used in the biofiltration system for propagation of inoculated microorganisms, biodegrading acetone, xylene, and ammonia. At the inlet loading rate of 120 g/m3/hr, the highest elimination capacity of xylene was 103 g/m3/hr, that of ammonia was 102 g/m3/hr, and that of acetone was 97 g/m3/hr, respectively. The morphological structure of biochar can be affected by the aggressive air contaminants, causing the change in the medium specific surface area, which is one of the factors controlling the biofilter performance. Although biological effects in biofiltration are typically considered to be more important than physical effects, the former may be more important for compounds with high Henry’s Law coefficient values, and the biofilter design should thus provide conditions for better compound absorption.
Introduction
The European Commission reported that the air pollution caused premature deaths of 420,000 people in the European Union (EU) in 2010. Volatile compounds, including acetone, xylene, and ammonia are dangerous air pollutants, and, therefore, new measures and targets for protecting human health and the environment from them must be set for the period until 2030 (Pagans et al., Citation2006; Beck-Friis et al., 2001).
Promotion of cleaner technologies for industry is included in the EU strategic plan for the cleaner air (European Environment Agency [EEA], Citation2012). Biofiltration is a biological treatment method that belongs to cleaner technologies because it does not produce secondary air pollutants, but helps to integrate natural processes in microorganisms for decomposing volatile air pollutants and solving odor problems (Malhautier et al., Citation2005). It can also be used with simple design, allowing for easily available and local materials to be applied as the biofilter bed medium (Baltrėnas and Zagorskis, Citation2009, Citation2010; Gallastegui et al., Citation2011; Mitin et al., Citation2015). The biofiltration process is widely used in the chemical industry, at domestic and industrial wastewater treatment plants, and in composting equipment systems, timber production, and varnish and surface coating processes. Only in Europe, biofiltration is employed in more than 600 chemical companies (Mudliar et al., Citation2010). Biofiltration is not only an effective tool for decreasing pollutant concentrations, but it also assists in dealing with the problem of neutralizing the odors produced by the spread of pollutants (Sakuma et al., Citation2008).
In a biofiltration system, various processes, including the diffusion in the gas phase, solubility and reactions in the liquid phase, as well as diffusion to biofilter bed medium pores, adsorption on the biofilter bed medium, and biomass degradation, take place. The efficiency of biofiltration highly depends on the cultures of microorganisms proliferating in the biofilter bed medium. Therefore, a proper selection of the biofilter bed medium for a biofiltration system ensures the effective operation of a biofilter from the environmental point of view, as well as providing its economic feasibility. Biochar seems to be a promising medium for a biofiltration system based on the following practical considerations: (i) a relatively low cost due to local availability and the ease of preparation (e.g., avoiding activation of the adsorbent); (ii) a high potential of regeneration because of the prevalence of physical adsorption; (iii) low probability that the adsorbent will cause chemical reactions (Komkienė and Baltrėnaitė, 2015); (iv) the convenience of filling large-volume biofiltration systems; (v) thermal and chemical stability; (vi) durability (5–8 yr) (Schmidt, Citation2012); and (vii) a possibility of producing an adsorbent from biodegradable (wood) waste (Zheng et al., Citation2015). This helps to implement the requirements for the end-of-waste status following the Directive 2008/98/EC, as well as promoting innovative environmental protection technologies and anticipating an opportunity for utilizing biochar as soil amendment (Schmidt, Citation2012; Baltrėnaitė et al., Citation2016b). The paper is aimed at investigating the efficiency of a biofilter in removing volatile compounds (acetone, xylene, and ammonia) from the air, when a biofiltration system contains the biochar-based bed medium inoculated with microorganisms. Various temperatures and pollutants with dissimilar properties were applied to evaluate the sustainability of a biofiltration process, as well as the viability of the introduced microorganisms over the period of 3 months of the biofilter operation.
Methodology
The setup of the biofilter
The schematic view of the biofilter is given in . The polluted air is supplied through an inlet duct with an inner diameter of 42 mm. The duct has an installed blower that is used to introduce and control the airflow rate and pressure. The air passed through the blower is heated with the help of an air-heating element. The polluted airflow passes through the valve and gets into the aqueous medium (with nutrient supplement) of the biofilter. Then, through the tubes filled with the biofilter bed medium and facing the ongoing process of treating, polluted airflow moves towards the purified air duct. Water temperature could be observed on the screen of the gauge measuring the temperature of the aqueous medium. Under the pressure created by the blower, the treated air moves upward towards the purified air duct, 96 mm in diameter.
Figure 1. A scheme of the biofilter: (a) front view; (b) a view of the section B–B; (c) a view of the section A–A. 1 is the inlet of the polluted air; 2 is the gauge of the airflow rate, temperature, and humidity; 3 is the outlet of the purified air; 4 is the biofilter lid; 5 is the perforated sealing plate that holds tubes above the tank with aqueous media containing nutrients; 6 is the fastener of the sealing plate; 7 denotes the biofilter tubes filled with the biochar-based medium; 8 is the biofilter casing; 9 is the aqueous medium refilling tank; 10, 17 are the valves; 11 denotes the biofilter poles; 12 is the heating element of the aqueous medium; 13 is the air distribution system; 14 is the drain valve; 15 is the control unit; 16 denotes the blower and the air heating element; X1, X2, D1, D2, D3, D4, and D5 are the points of measuring biofilter parameters; and M1, M2, M3, M4, M5 are the points of sampling the biofilter bed medium.
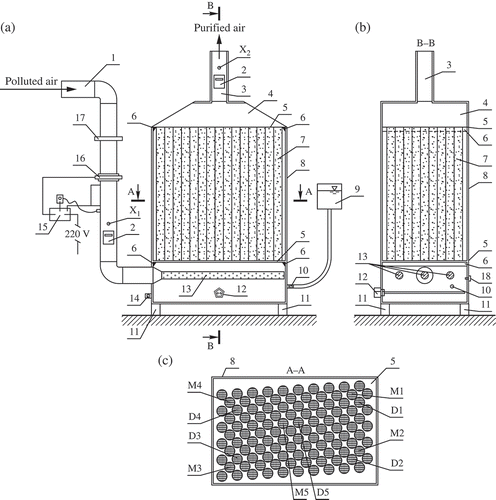
The selection of the biofilter bed medium
The birch wood biochar has been chosen as a principal material for the biofilter bed medium. Due to its hydrophobicity, biochar is suitable for adsorbing nonpolar and weakly polar pollutants better than water (in particular, with regard to the structure examined in this paper, when the packing material was additionally humidified because of the capillary effect). Biochar was mixed with birch fiber (10:1), whereas the fiber was chosen as a maintaining nutrition substrate for microorganisms. Biochar was prepared at a temperature of 750 °C. The biochar of this type has a large BET (Brunauer-Emmett-Teller method) surface area (300–400 m2/g) due to the developed microporous system, providing conditions for the adsorption of pollutants, as well as for the survival of microorganisms (Baltrėnas et al., Citation2015).
The operation conditions of the biofilter
The biofilter performance was tested for the period of 3 months at the airflow rate of 100 ± 5 m3/hr after the adaptation period (at the airflow rate of 30 ± 1.5 m3/hr). The biofilter was supplied with acetone vapor at the temperatures of 24, 28, and 32 °C. For the first 2 weeks, the temperature was maintained at 24 °C, 28 °C for the next 2 weeks, and 32 °C for the final 2 weeks. Following a similar principle, the investigation of two other pollutants, i.e., xylene and ammonia, was carried out.
The concentration of the pollutants was selected on the basis of the results of the experimental research performed using the laboratory models for biofilters. Thus, the pollutant (acetone, xylene, or ammonia) with the concentration of 300 ± 25 mg/m3 was injected daily, and the removal efficiency was monitored. The concentration of the pollutant was measured before (X1) and after (X2) it passed through treatment equipment. The measurement points are shown in .
Moreover, the moisture of the bed medium was checked every day of the experiment. The airflow rate and temperature were measured in the inlet and outlet air ducts. A composite sample of the packing material has been examined twice a week to determine the development of microorganisms. For the examination of microorganisms, the composite sample was taken from five places following the envelope principle (four samples were taken from tubes in the corners and the fifth one was taken from the tube at the midpoint of the cross-sectional area of the biofilter medium).
The efficiency of the removal of the gas pollutant, as well as the elimination capacity, was calculated using equations provided by Devinny et al. (Citation1999).
Morphological analysis of biochar
From the beginning to the end of the research, the morphological parameters of the packing material (diameter and volume of the pores and the surface area) have been tested four times. The first specimen was taken on the first day of the research, the second specimen on the first day following the research period of removing acetone, the third specimen on the first day following the research period of removing xylene, and the fourth specimen was taken on the last day of the research period of removing ammonia. The dimensions of the selected biochar specimens before starting the research were 10 × 6 × 3 mm.
The tests on the material’s pore structure were performed with a mercury intrusion porosimeter (Quantachrome Poremaster PM-33-12; Hartley Wintney, UK). The maximum pressure developed during the process of measurement was 231 MPa. The measurements involved the pore diameters from 1069 to 0.006469 μm. Prior to testing, the material was dried to constant mass at 105 °C. Mercury does not wet a biochar sample and can access the pores only under pressure. When the pressure is increased, mercury penetrates smaller pores and capillaries. The diameter of the pores was calculated according to the Washburn’s (1921) equation, pr = −2γ cos θ, where p is pressure, r is the pore radius, γ is the surface tension of mercury, and θ is the wetting angle of mercury and the material analyzed.
The data used during the experiments were as follows: the surface tension of mercury was 480 erg/cm2, and the wetting angle for mercury was 140°. Morphological tests on biochar were performed using scanning electron microscopy (SEM) (Giffin et al., Citation2013). The SEM analysis was performed using a field-emission scanning electron microscope (JEOL ISM-7600 F) The samples without coating were analyzed using low voltage (4 kV) (Baltrėnas et al., Citation2015).
The inoculation of the medium
Ten strains were selected for inoculating the biofilter. The set included fungi (Aspergillus versicolor BF-4, Cladosporium herbarum 7KA, Gliocladium virens BF-81, Stachybotrys sp. BF-90), yeast (Aureobasidium pullulans BTLAP1.2, Exophiala jeanselmei BTLBF1, Sporobolomyces roseus BTLBF23), and bacteria (Bacillus subtilis, Burkholderia cepacia [= Burkholderia convexa], Rhodococcus sp.), which were selected as microorganisms tolerant to the emissions of acetone, xylene, and ammonia (Mačaitis et al., Citation2014). The cultures grown in test tubes were washed with sterile saline and filtered through sterile cotton wool. All suspensions were mixed and diluted to make 1 L of solution so that the amount of conidia (cells) would be equal at least to 108 colony-forming units (CFU)/mL. The suspension was evenly sprayed with a hand sprayer over the biofilter medium at the beginning of the experiment.
The serial dilution technique was used to count the microorganisms from the biofilter. One gram wet weight of biofilter packing material was added to 99 mL of sterilized saline shaken at 200 rpm for 10 min, and a dilution series was prepared. One milliliter of the suitable suspension was plated in Petri dishes in three replicates and then filled with a particular medium. Thus, nutrient agar (Oxoid, Hampshire, UK) was used for bacteria, whereas Sabouraud Chloramphenicol (CAF) agar (Liofilchem, Italy) was used for yeast and malt agar with chloramphenicol (250 mg/L for bacterial growth inhibition) was employed for fungal isolation. The cultures were incubated at 26–30 °C temperature for 2–7 days. After incubation, the microbial colonies were counted and colony-forming units per gram of dry weight (CFU/g DW) of biofilter were calculated.
The aqueous medium with nutrients
The aqueous medium employed was used to maintain the optimal moisture in the biofilter medium and was prepared using the following components: 1 g K2HPO4; 0.5 g KCl; 0.5 g MgSO4∙7H2O; 0.1 g FeSO4∙7H2O; 0.90g NaNO3, and 1000 g of water (Baltrėnas and Zagorskis, Citation2009). Aqueous medium was added and replaced with a new one once the sediments appeared.
Results and discussion
The morphology of biochar
Two types of granules, such as a metallic-black glossy surface and a matt black surface, were monitored in the selected biochar specimens. Two types of pores, having large (40–45 μm) and small (5–8 μm) diameters ( and ), could be observed in the cross-section of the biochar specimens. The observed small amounts of wood fiber on the surface of the tested biochar are shown in . The membranes completely covering the cross-section of small-diameter pores could be observed in the cross-section ().
The analyzed data on the elemental composition of biochar surfaces were obtained using the spectrometer Inca Energy 350, measuring energy dispersion of the X-ray waves of an electronic microscope ().
Figure 3. Elemental analysis of (a) walls of biochar pores and (b) membranes of small biochar pores.
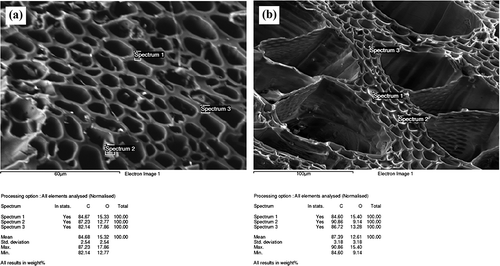
The developed method fails to identify H; therefore, it can be maintained that the employed biochar contains elemental carbon and a relatively significant amount of transitive organic compounds. The elements C and O were found in the walls of the pores of biochar. Biochar (without taking into account H) consisted, on average, of 84.68% of C and 15.32% of O. The membranes completely covering the small pores of biochar could be observed for the first time. In order to identify their nature, elemental analysis was performed. The obtained findings are shown in . These membranes must be attributed to the structure of wood. Their elemental composition only slightly differs from that of the walls of the pores; however, the content of C in them is higher (87.39%), and that of O is lower (12.61%). Although these elements are of extremely small thickness, the full transition of organic compounds of wood to C had not been reached.
The views of the surface structure of biochar specimens processed with acetone in the tubular biofilter are presented in and . Birch fiber could not be observed on the surface and the membranes covering the cross-section of the pores in these specimens. The diameter of the large pores observed in the specimen was about 50–60 μm, and that of the small ones was around 6–7 μm. The new structures with the elongated shape (0.5–1 μm) found on the lateral surfaces of the pores, which could not be observed in the specimen of biochar before processing, could be monitored (). The views of the porous structure of biochar processed with xylene and ammonia in the tubular biofilter are given in . The images showed the damage to the outer layer of biochar. The damaged tracheids, presenting the uneven surface and a number of small fragments 10–50 μm in length, could be observed.
Figure 4. Specimens of biochar processed with acetone: (a) magnification, ×100; (b) magnification, ×3000.
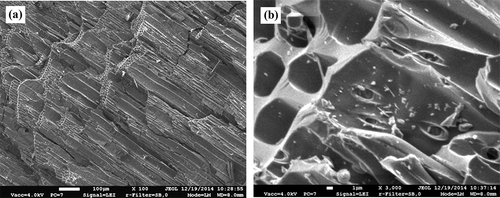
Figure 5. A treated specimen of birch biochar: (a) magnification, ×500 (xylene); (b) magnification, ×1000 (ammonia).
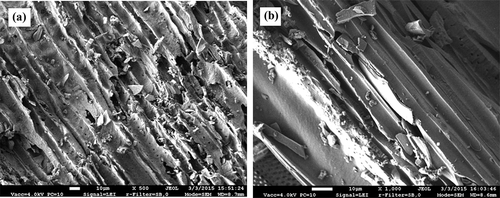
The data on the research into the porous structure of birch biochar speciments were obtained applying the mercury porosimetry technique and are given in . The obtained data show considerable differences in the porous structure of biochar. This may be due to difficulties in selecting the model specimen from the tested samples. As mentioned above, the biochar samples varied in color and, obviously, in structure. However, the porosity (62.89%) of the nontreated specimens and the surface area of the pores (6.15 m2/g) in particular were significantly lower than those of the treated specimens. The specified volume of the pores was also lower. The impact of xylene was strong, which dramatically increased the surface area of biochar pores. This can be explained by the impact of xylene on residual organic compounds. Each living cell (including wood cells) contains lipids as a storage form of food. Lipids and their different forms have low solubility in water, but are highly soluble in organic solvents. The lipid-dissolving properties of xylene make it easy to dissolve the lipid-containing cell membranes (Niaz et al., Citation2015) and thus to increase the surface area. It implies that during biochar production, some lipids are retained and react with pollutants during biofiltration.
Table 1. The changes of the porous structure of birch biochar.
When the BET method for determining the specific biochar surface area was used (see Baltrėnaitė et al., Citation2016a), the obtained larger value was even higher (about 291 m2/g), because this method can access the contribution of micropores, rather than macro- and mesopores in the specific surface area of the material. Although this surface area, similar to that of biochar obtained from the widely known Giant miscanthus (360 m2/g) (Lee et al., Citation2013), is several times smaller than that of activated carbon (although the price of wood biochar can be up to 4 times lower than that of activated carbon [personal communications]), it is considerably higher than that of biochar obtained from some popular bioproducts. For example, the surface area of green algae used for getting biochar may reach 1.15–4.26 m2/g (Bird et al., Citation2011), whereas that of bone biochar is 107 m2/g (Ip et al., Citation2010) and that of soybean 122 m2/g (Ahmad et al., Citation2012). The development of a larger specific surface area would cause the increase in adsorption, which is one of the most important processes in biofiltration system and also plays a significant role in the formation of a large surface area. In its turn, microporosity of biochar may reach 77% and micropores might occupy the volume of 119 mm3/g, thereby increasing gas adsorption (Pan and Van Staden, Citation1998). Micropores improve the retaining of gaseous pollutants because bigger molecules (0.4–0.6 nm) of the investigated gaseous pollutants are better retained in narrower pores (the average diameter of micropores according to the IUPAC [International Union of Pure and Applied Chemistry] is about 2 nm) (Ip et al., Citation2010). On the other hand, maintaining the structure of meso- and macropores creates the conditions for more efficient pollutant biodegradation due to the creation of favorable conditions for microorganisms, when the average size of microorganisms participating in biofiltration is 1–3 μm. Therefore, microorganisms can exist in the pores, which are larger than 1 μm.
Determining the amount and composition of microorganisms
All groups of microorganisms were found to be able to operate in the biofilter packed with the mixture of biochar and fiber under the conditions of temperature variation from +24 to +32 °C and the injection of contaminants, such as acetone, xylene, and ammonia. During the filtration of acetone under the considered temperatures of 24, 28, and 32 °C, the number of fungi remained almost unchanged and reached 108 CFU/g DW, whereas the largest number was found in the last week of investigation and reached 1.8 × 109 CFU/g DW (). The number of yeast microorganisms gradually increased with the temperature rising from 24 to 32 °C. The lowest amount of yeast microorganisms was found within the first week and the highest amount during the last week of research (1.1 × 107 and 4.1 × 109 CFU/g DW, respectively). The number of bacteria was highly dependent on temperature. It is interesting to note that the highest number of bacteria was recorded under the injection of acetone at a temperature of 24 °C (2.4 × 1010 CFU/g DW). The increase in the temperature up to 28 °C resulted in the reduction in bacteria to 109 and 108 CFU/g DW, whereas under the increase in temperature to 32 °C, the number of bacteria started gradually growing and reached 1.3 × 1010 CFU/g DW. Thus, it can be supposed that the growth of bacteria was not significantly affected by acetone.
Figure 6. The number of microorganisms found on the biofilter during filtration of acetone at different temperatures.
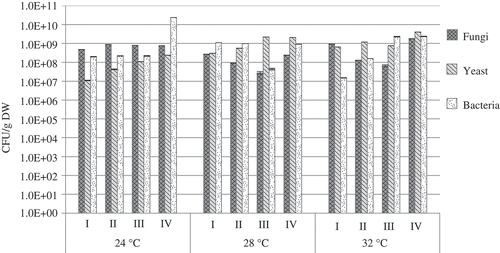
In the filtration of xylene at all the investigated temperatures, 24, 28, and 32 °C, the number of fungi reached 108–109 CFU/g DW (). The largest number of fungi, compared with yeast and bacteria, was found under the temperature of 24 °C. Thus, it can be concluded that this temperature is most favorable for the growth of fungi, whereas xylene has little impact on them. Meanwhile, under the temperatures of 28 and 32 °C, the number of the investigated yeast microorganisms was the highest during the whole experiment: it varied from 1.4 × 1010 to 2.9 × 1010 CFU/g DW (32 and 28 °C, respectively). The number of bacteria under the investigated temperatures did not exceed 109 CFU/g DW.
Figure 7. The number of microorganisms found on the biofilter during filtration of xylene at different temperatures.
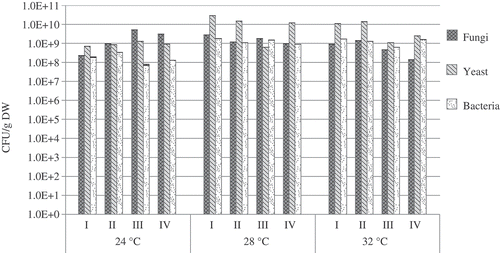
During the filtration of ammonia at the temperatures of 24 and 28 C, the number of bacteria gradually increased from 109 to 1010 CFU/g DW, whereas at a temperature of 32 °C, the number of these microorganisms decreased again to 109 CFU/g DW (). Meanwhile, the highest number of fungi and yeast microorganisms was established at a temperature of 24 °C (5.8 × 109 and 2.9 × 109 CFU/g DW, respectively). A further increase in temperature resulted in the reduced number of the above-mentioned microorganisms. Thus, it can be concluded that a higher temperature and vapor of ammonia inhibit the growth and development of microorganisms.
Figure 8. The number of microorganisms found on the biofilter during filtration of ammonia at different temperatures.
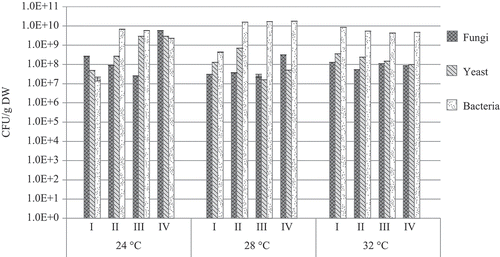
Thus, the findings of the conducted research revealed that bacteria, yeast, and fungi managed to function in the tubular biofilter filled with the packing material of biochar at the temperatures changing from 24 to 32 °C and when such contaminants such as acetone, xylene, and ammonia were injected. For the growth and development of fungi in the course of filtering volatile materials, including acetone, xylene, and ammonia, a temperature of 24–28 °C was found to be optimal, whereas that for yeast and bacteria was 28–32 °C. The inoculated microorganisms, such as yeast Exophiala jeanselmei, bacterium Bacillus subtilis, and fungus Cladosporium herbarum, as well as Aspergillus versicolor, were identified throughout the whole experiment of filtering the investigated volatile compounds (acetone, xylene, and ammonia) found in the air under the temperatures of 24–32 °C.
The penetration coefficient of the packing material
Assuming that the packing material of the tube makes a porous medium through which the polluted air gets through and with reference to Darcy’s law, the basic vectorial relation of laminar filtration was obtained as follows:
where v = (u, v, w) (m/sec) is a vector of the filtration rate, μ is the coefficient of the dynamic viscosity of the supplied air (Ns/m2), p is pressure (Pa), and k is the penetration coefficient of the porous medium (m2). Since movement is only in one direction, i.e., along the tube, supposedly, in the direction of the Ox axis, eq 1 is scalar:
Moreover, the rate must satisfy the equation of continuity:
where m is the porosity of the packing material defined as the volume of the pores of the packing material in a unit of its volume and ρ is density (kg/m3). When the packing material is not deformed, the volume of the pores remains constant, thus m = constant; consequently, we obtain from eq 3 that
The unknown pressure p is usually replaced with the function q(p) in the gas filtration theory:
Then,
and eq 4 becomes a parabolic equation:
for finding a solution, to which the formulation of the appropriate boundary and initial conditions is required.
In the case of the polytrophic process p1/n = RTρ, where n is a polytrophic index, R is gas constant (J/(kg K)), and T is temperature (K). Due to the fact that filtration takes place under constant temperature (isothermal process n = 1), we have
thus, eq 9 is obtained:
Under a stationary regime (observed in the course of conducting the experiments),
the function q becomes harmonic:
i.e., q(x) is a linear function q(x) = c1 + c2x, whereas the constants c1 and c2 are obtained based on the values of pressure at the initial x = 0, p(0) = p1 and final x = L, p(L) = p2 points of the tube. With reference to eq 8,
is obtained; thus, the constants c1 and c2 can be calculated taking into account that a change in pressure will take place in accordance with the parabolic law:
Moreover, with reference to eqs 2, 6, 12, and 13, the expressions of the filtration rate are as follows:
and the laws of rate and density variation (along the tube) are obtained:
Considering the specific structure, which includes a biofilter made of a number of tubes, the penetration coefficient k of the employed packing material was calculated. The performed tests show that the variations in the air pressure at the inlet (the tube is 42 mm in diameter, the length L1 = 0.2 m, the air rate v1 = 20 m/sec, Re = 84,000) and the outlet (the tube is 96 mm in diameter, length L2 = 0.2 m, the air rate v2 = 3.8 m/sec, Re = 36,480) of the biofilter between the measurement points reach approximately 450 Pa. To estimate the aerodynamic resistance of the packing material in the biofilter, the drops in pressure on the way from gauges to the packing material had to be rejected. This is a turbulent motion regime, and, therefore, the above equation was used:
where the parameter is found from the equation:
Consequently, λ1 = 0.019, λ2 = 0.022, and Δp1 = 23 Pa, Δp2 = 0.5 Pa. The substantial resistance is caused by a bend of the inlet pipe, which is equal to
Therefore, the aerodynamic resistance of the packing material of a single tube was equal to 375 Pa. Then, with reference to eq 15, when ρ = 1.29 kg/m3, u = 0.13 m/sec, μ = 2 × 105 N·sec/m2, R = 287 J/(kg·K), T = 300 K, L = 0.6 m, p1 = 105 Pa, p2 = p1 − 375 Pa, the penetration coefficient of the packing material k = 4.6 × 10−9 m2 was obtained.
The efficiency of the biofilter
The concentration of pollutants supplied to the biofilter remained constant (300 mg/m3). The air pressure reached on average 470 Pa in the air supply duct and 28 Pa in the air exhaust duct, whereas the tubes maintained the pressure of 8.2 Pa. The trends of the variation in the efficiency of filtering acetone, xylene, and ammonia vapor through the packing material of biochar and wood fiber are shown in –.
Figure 9. The dependence of air treatment efficiency in the biofilter on the supplied air polluted with acetone vapor and the maintained air temperature: (A, B) 24 °C; (C) 28 °C; (D) 32 °C.
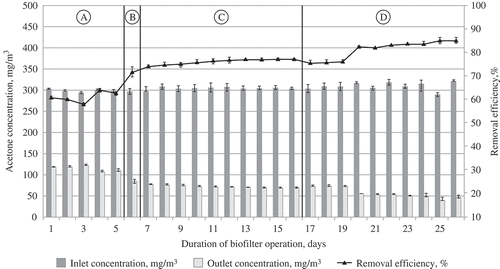
Figure 10. The dependence of air treatment efficiency in the biofilter on the supplied air polluted with xylene vapor and the maintained air temperature: (A) 24 °C; (B) 28 °C; (C) 32 °C.
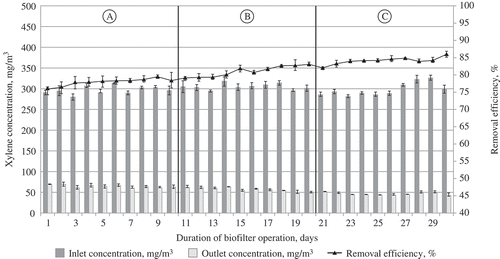
Figure 11. The dependence of air treatment efficiency in the biofilter on the supplied air polluted with ammonia vapor and the maintained air temperature: (A) 24 °C; (B) 28 °C; (C) 32 °C.
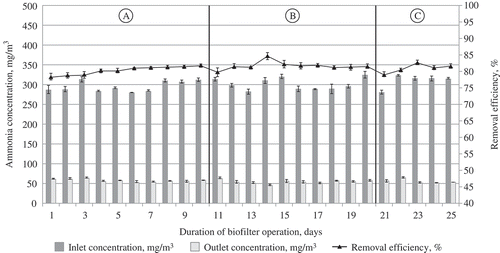
Thus, the use of tubular biofilters and removal of acetone and xylene vapor, irrespective of the air temperature (i.e., in the course of the whole research), allowed us to observe an increase in efficiency, up to 0.25–0.30% per day. The situation can be explained by the monitored ongoing increase in the population of microorganisms. Therefore, higher efficiency of a biofilter of a similar structure can be achieved after a longer period of time.
Completely different trends of efficiency variation at particular temperature-controlled stages were determined, when the results of research on the air polluted with the inorganic compounds of ammonia were examined. Although a general trend towards an increase in efficiency remained, it was much slower and reached only 0.05–0.10% per day on average. Thus, a maximal value was obtained at the initial stage, i.e., 10 days, whereas later the efficiency of the biofilter remained uniform and reaching ≈80%. This could be determined by the above-mentioned steady number of microorganisms in the ammonia medium.
It should be mentioned that no major changes could be observed in comparing the work of the models with the laboratory biofilters filled with inoculated packaging material, which allowed us to more accurately interpret the research results obtained in the performed tests.
presents the data on the dependence of the efficiency of removing acetone, xylene, and ammonia from the airflow for three temperature ranges. It is clearly shown that this dependence differs for various pollutants. In the case of acetone and xylene, higher efficiency of their removal could be observed at a higher airflow temperature. This proves the statement that a warmer reactor treats the contaminants faster, and microbial metabolic activity increases proportionally through the temperature range, where the coordination of the reactions can be maintained. However, the statement that the activity of microorganisms (i.e., a biological factor) is the dominant factor in a biofiltration system is true only ifthe Henry law coefficients of the examined contaminants are lower, which means that they can be faster dissolved in water or other solution used for moisturizing biofilter media. In the considered case, higher Henry law coefficient values (kHϴ = ca/pg, where ca is the contaminant concentration in the water phase, pg is a partial contaminant pressure in the gas phase, and ϴ is mean the standard conditions [Tϴ = 298.15 K]) are characteristic of ammonia (up to 76 M/atm) rather than of other contaminants, such as acetone and xylene (22–35 M/atm and 0.19–0.25 M/atm) (Sander, Citation1999). Thus, in the cases of less soluble contaminants, whose Henry law coefficients are larger, physical factors manifest themselves more clearly in a biofiltration system. A decrease in gas solubility is probably the most important trend under the increasing temperature. The contaminants with higher Henry law coefficients (ammonia, in this case) will have even higher values of these coefficients, implying that a smaller amount of the compound will be dissolved in water. The sorption of the compound will probably be reduced. Another effect is that transfer of the contaminant from the air to microorganisms is less effective. According to Budavari (Citation1996), the variation of ammonia solubility depends on temperature as follows: at the temperature of 20 °C, its solubility is 33–34%, whereas at 25 °C it is 31–34%, at 30 °C it is 25%, and at 50 °C it is 18%. A similar trend can be also observed in the case of a biofilter at the temperature of ammonia removal at 28 °C. The removal effectiveness was higher than that obtained at 24 °C; however, at the temperature of 32 °C, it was lower and similar to the value observed at the temperature of 24 °C. This idea was supported by McNevin and Barford (Citation2000), who explained that the biofilter efficiency of ammonia removal in the biofilter was based on the fact that ammonia was absorbed due to the biofilter humidity and adsorption on the medium rather than on the reduction of its amount due to biodegradation. Bird et al. (Citation2011) reported a similar trend in the pattern of ammonia removal of gases from composting facilities during biofiltration.
Conclusions
The examined model of a biofilter, characteristic of the aerodynamic biofilter with the resistance of 4050 Pa and the penetration coefficient of the bed medium being 4.6 × 10−9 m2, was suitable for removing acetone, xylene, and ammonia from the polluted air. At the inlet loading rate of 120 g/m3/hr, the highest elimination capacity of xylene in the biochar-based biofilter with the inoculated medium was 103 g/m3/hr, whereas that of ammonia was 102 g/m3/hr and that of acetone was 97 g/m3/hr, respectively. The maximum removal efficiency reached 86%, 85%, and 81%, respectively.
Inoculated bacteria, fungi, and yeast were found to be able to function on biochar-based tubular-type biofilter for removal acetone, xylene, and ammonia from the air. The biochar employed in the biofilter was appropriate for developing microorganisms, because the population reached 107–109 CFU/g for fungi, 107–1010 CFU/g for yeast, and 108–1010 CFU/g for bacteria, respectively.
Physical investigation of the packing materials of the biofilter has revealed that the damage to the surface structure of biochar increases along with the increase in the length of the treatment period. After processing with xylene and ammonia, the damage to the outer layer of biochar, in the form of an uneven surface and a number of small fragments 10–50 μm in length on the surface, could be observed. The porosity of unprocessed biochar reached 62.9%, whereas the surface area of the pores (6.15 m2/g), in particular, was extremely smaller than that of the processed specimens. The impact of xylene should be regarded as rather strong, remarkably increasing the surface area of biochar pores up to 20.39 m2/g. This can be explained by the impact of xylene on residual organic compounds, which melts them, and this results in the development of an additional structure of micropores.
The temperature regime, although characterized by some rapid changes, can hardly considerably influence the biological effect (i.e., microbiological activity) of biofiltration, but can lead to the changes in physical properties (e.g., solubility) of the investigated compounds. These changes can occur when the aqueous medium is designed to have a controlling role in the biofiltration system and the investigated compounds (e.g., ammonia) have high Henry law coefficient values.
Funding
The research was carried out within the framework of project “Applied research and technological development of plate type air treatment biofilter with a capillary humidification system for packing material ‘BIOFILTER’” (project no. VP1-3.1-ŠMM-10-V-02-015) under the Operational Programme for the Development of Human Resources 2007–2013 priority axis 3 “Strengthening researchers abilities,” measure VP1-3.1-ŠMM-10-V “Promotion of high level international research.” The project was supported and co-funded by the European Union and the Republic of Lithuania
Additional information
Funding
Notes on contributors
Pranas Baltrėnas
Pranas Baltrėnas is Professor and Dr. Habil in the Institute of Environmental Protection, Institute of Vilnius Gediminas Technical University, Vilnius, Lithuania.
Edita Baltrėnaitė
Edita Baltrėnaitė is an associated professor in the Department of Environmental Protection, Vilnius Gediminas Technical University, Vilnius, Lithuania.
Jonas Kleiza
Jonas Kleiza is a professor at the Department of Mathematical Modelling in Vilnius Gediminas Technical University, Vilnius, Lithuania.
Jurgita Švedienė
Jurgita Švedienė is a researcher at the Laboratory of Biodeterioration Research, Nature Research Centre, Vilnius, Lithuania.
References
- Ahmad, M., S.S. Lee, X. Dou, D. Mohan, J.-K. Sung, J.E. Yang, and Y.S. Ok. 2012. Effects of pyrolysis temperature on soybean stover- and peanut shell-derived biochar properties and TCE adsorption in water. Bioresour. Technol. 188:536–544. doi:10.1016/j.biortech.2012.05.042
- Baltrėnaitė, E., Baltrėnas, P., and Lietuvninkas, A. 2016a. The Sustainable Role of the Tree in Environmental Protection Technologies. Monograph. Germany: Springer.
- Baltrėnas, P., Baltrėnaitė, E., and Spudulis, E. 2015. Biochar from pine and birch morphology and pore structure change by treatment in biofilter. Water Air Soil Pollut. 226:1–14. doi:10.1007/s11270-015-2295-8
- Baltrėnaitė, E., Lietuvninkas, A., and Baltrėnas, P. 2016b. Modeling the balance of metals in the amended soil for the case of ‘atmosphere-plant-soil’ system. Environ. Model. and Assess. In press. doi:10.1007/s10666-016-9505-7
- Baltrėnas, P., and Zagorskis, A. 2009. Investigation of cleaning efficiency of a biofilter with aeration chamber. J. Environ. Eng. Landscape Manage. 17:12–19.
- Baltrėnas, P., and Zagorskis, A. 2010. Investigation into the air treatment efficiency of biofilters of different structures. J. Environ. Eng. Landscape Manage. 18:23–31. doi:10.3846/jeelm.2010.03
- Beck-Friis, B., Smars, S., Jonsson, H., Eklind, Y., and Kirchmann, H. 2003. Composting of source-separated household organics at different oxygen levels: Gaining an understanding of the emission dynamics. Compost Sci. Util. 11:41–50. doi:10.1080/1065657X.2003.10702108
- Bird, M. I., Wurster, C. M., de Paula Silva, P. H., Bass, A. M., and de Nys, R. 2011. Algal biochar—Production and properties. Bioresour. Technol. 102:1886–1891. doi:10.1016/j.biortech.2010.07.106
- Budavari, S. (ed.). 1996. The Merck Index: An Encyclopedia of Chemicals, Drugs and Biologicals, 12th ed. Whitehouse Station, NJ: Merck Research Laboratories.
- Devinny, J. S., Deshusses, M. A., and Webster, T. S. 1999. Biofiltration for Air Pollution Control. Boca Raton, FL: CRC Lewis Publishers.
- European Environment Agency. 2012. Air Quality in Europe—2012 Report. Report No. 4/2012, http://www.eea.europa.eu/publications/air-quality-in-europe-2012 ( accessed January 28, 2016).
- Gallastegui, G., Ramirez, A.A., Elias, A., Jones, J.P., and Heitz, M. 2011. Performance and macrokinetic analysis of biofiltration of toluene and p-xylene mixtures in a conventional biofilter packed with inert material. Bioresour. Technol. 102:7657–7665. doi:10.1016/j.biortech.2011.05.054
- Giffin, S., Littke, R., Klaver, J., and Urai, J. L. 2013. Application of BIB-SEM technology to characterize macropore morphology in coal. Int. J. Coal Geol. 114:85–95. doi:10.1016/j.coal.2013.02.009
- Ip, A. W. M., Barford, J. P., and McKay, G. 2010. Biodegradation of reactive Black 5 and bioregeneration in upflow fixed bed bioreactors packed with different adsorbents. J. Chem. Technol. Biotechnol. 85:658–667. doi:10.1002/jctb.v85:5
- Komkienė, J., and Baltrėnaitė, E. 2016. Biochar as adsorbent for removal of heavy metal ions (cadmium(II), copper(II), lead(II), zinc(II)) from aqueous phase. Int. J. Environ. Sci. Technol. 13:471–482. doi:10.1007/s13762-015-0873-3
- Lee, Y., Eum, P. R. B., Ryu, C., Park, Y.-K, Jung, J.-H., and Hyun, S. 2013. Characteristics of biochar produced from slow pyrolysis of Geodae-Uksae 1. Bioresour. Technol. 130: 345–350. doi:10.1016/j.biortech.2012.12.012
- Mačaitis, K., Misevičius, A., Paškevičius, A., Raudonienė, V., and Repečkienė, J. 2014. Effectiveness research on a wavy lamellar plate-type biofilter with a capillary system for the humidification of the packing material applying intro induced microorganisms. J. Environ. Eng. Landscape Manage. 22: 254–263. doi:10.3846/16486897.2014.972409
- Malhautier, L., Khammar, N., Bayle, S., and Faulo, J.L. 2005. Biofiltration of volatile organic compounds. Appl. Microbiol. Biotechnol. 68:16–22. doi:10.1007/s00253-005-1960-z
- McNevin, D., and Barford, J. 2000. Biofiltration as an odour abatement strategy. Biochem. Eng. J. 5:231–242. doi:10.1016/S1369-703X(00)00064-4
- Mitin, A., Nikolajkina, N., and Pushnov, A. 2015. Aerodynamic resistance of a biofilter with a packing of pine cone. J. Environ. Eng. Landscape Manage. 23:138–146. doi:10.3846/16486897.2015.1009912
- Mudliar, S., Giri, B., Padoley, K., Satpue, D., Dixit, R., Bhatt, P., Pandey, R., Juwarkar, A., and Vaidya, A. 2010. Bioreactors for treatment of VOCs and odours. A review. J. Environ. Manage. 91:1039–1054. doi:10.1016/j.jenvman.2010.01.006
- Niaz, K., Bahadar, H., Maqbool, F., and Abdollahi, M. 2015. A review of environmental and occupational exposure to xylene and its health concerns. EXCLI J. 14:1167–1186.
- Pagans, E., Barrena, R., Font, X., and Sanchez, A. 2006. Ammonia emissions from the composting of different organic wastes. Dependency on process temperature. Chemosphere 62:1534–1542. doi:10.1016/j.chemosphere.2005.06.044
- Pan, M. J., and van Staden, J. 1998. The use of charcoal in in vitro culture—A review. Plant Growth Regul. 26:155–163. doi:10.1023/A:1006119015972
- Sander, R. 1999. Compilation of Henry‘s Law constants for inorganic and organic species of potential importance in environmental chemistry, version 3. Available at: http://www.mpch-mainz.mpg.de/~sander/res/henry.html ( accessed January 28, 2016).
- Sakuma, T., Jinsiriwanit, S., Hattori, T., and Deshusses, M.A. 2008. Removal of ammonia from contaminated air in a biotrickling filter—Denitrifying bioreactor combination system. Water Res. 42:4507–4513. doi:10.1016/j.watres.2008.07.036
- Schmidt, H.P. 2012. 55 uses of biochar. Ithaka J. 2012:286–289. www.ithaka-journal.net ( accessed January 28, 2016).
- Zheng, R., Chen, Z., Cai, C., Tie, B., Liu, X., Reid, B.J., Huang, Q., Lei, M., Sun, G., and Baltrėnaitė, E. 2015. Mitigating heavy metal accumulation into rice (Oryza sativa L.) using biochar amendment—A field experiment in Hunan, China. Environ. Sci. Pollut. Res. 22(14):11097–11108 doi:10.1007/s11356-015-4268-2