ABSTRACT
To investigate the impacts of major factors on carbon loss via gaseous emissions, carbon dioxide (CO2) and methane (CH4) emissions from the ground of open dairy lots were tested by a scale model experiment at various air temperatures (15, 25, and 35 °C), surface velocities (0.4, 0.7, 1.0, and 1.2 m sec−1), and floor types (unpaved soil floor and brick-paved floor) in controlled laboratory conditions using the wind tunnel method. Generally, CO2 and CH4 emissions were significantly enhanced with the increase of air temperature and velocity (P < 0.05). Floor type had different effects on the CO2 and CH4 emissions, which were also affected by air temperature and soil characteristics of the floor. Although different patterns were observed on CH4 emission from the soil and brick floors at different air temperature-velocity combinations, statistical analysis showed no significant difference in CH4 emissions from different floors (P > 0.05). For CO2, similar emissions were found from the soil and brick floors at 15 and 25 °C, whereas higher rates were detected from the brick floor at 35 °C (P < 0.05). Results showed that CH4 emission from the scale model was exponentially related to CO2 flux, which might be helpful in CH4 emission estimation from manure management.
Implications: Gaseous emissions from the open lots are largely dependent on outdoor climate, floor systems, and management practices, which are quite different from those indoors. This study assessed the effects of floor types and air velocities on CO2 and CH4 emissions from the open dairy lots at various temperatures by a wind tunnel. It provided some valuable information for decision-making and further studies on gaseous emissions from open lots.
Introduction
Open lots are typical feeding operations for beef or dairy cattle in many countries (Eghball et al., Citation1994; Bjorneberg et al., Citation2009; Ding et al., Citation2015a). In such a system, a large amount of solid and liquid manure from the animals is usually produced on the floor surface, and cleaned from days to months, depending on the climate and floor systems. Reports showed that the open lot operations may cause some environmental issues such as nutrient loss through emissions (Eghball et al., Citation1994; Eigenberg et al., Citation1998; Kissinger et al., Citation2007). Carbon dioxide (CO2) is a major form of carbon loss via gaseous emissions (Hao et al., Citation2004). Although it may not be the biggest share of carbon loss via gaseous emission, methane (CH4) from livestock manure has proven to be an important source of greenhouse gas (GHG), which contributes to 15% of the total GHG emissions in agriculture (U.S. Environmental Protection Agency [EPA], Citation2012).
Gaseous emissions from animal manure on the open lots are reported to have increasingly been causing concerns in major livestock producing countries (Chianese et al., Citation2009; Mukhtar et al., Citation2008; Borhan et al., Citation2011a, Citation2011b; Ding et al., Citation2015b), and studies have been carried out to quantify the emissions. In such a dairy farming system, studies show that 58–93% of ground-level CO2 emissions and 1.7–45% of ground-level CH4 emissions are from the lots (Borhan et al., Citation2011a, Citation2011b).
Gaseous emissions from animal manure are affected by several important factors, including air temperature, wind, floor type, manure characteristics and management, etc. (Gonzalez-Avalos et al., Citation2001; Saha et al., Citation2010; Pereira et al., Citation2011, Citation2012; Owen and Silver, 2014). As for open lots, these factors might be quite different from those inside the barns, which may lead to the differences in gaseous emissions characteristics and quantities between the two sources. Aside from the different thermal environments such as air temperature, humidity, and velocity at outdoor conditions, the characteristics of the manure on open lots are also different. Moisture content of collected solid manure from the open lots ranges from 30% to 78%, whereas it is about 87% to 92% for well-mixed slurry or liquid manure collected in the barn or under stalls (Kissinger et al., Citation2007).
Compared with open operations, gaseous emissions from well-mixed slurry or liquid manure having a much higher water content in the barns were studied mostly. The typical air velocities over the slurry/manure surface normally ranged from 0.1 to 0.5 m sec−1 (Arogo et al., Citation1999; Ye et al., Citation2008; Saha et al., Citation2010), which are much lower than those of outdoor open lots. According to a field survey of open lots by the authors (Ding et al., Citation2016), the probability of wind speed higher than 0.5 m sec−1 reached 39–69% in different seasons. Unlike the barns with solid or slatted concrete floors, open lots are usually equipped with unpaved soil or brick-paved floors. The influence of floor type, especially the brick floor, on CO2 and CH4 emissions from open lots is rarely assessed. All of the differences mentioned may result in a big variation on gaseous emissions from the indoor operations.
Several field measurements had been done to quantify the gaseous emissions from open lots (Borhan et al., Citation2011a, Citation2011b; Ding et al., Citation2016), but these measured emission rates resulted from multiple factors under uncontrolled conditions. The emission pattern on a scale model with easily measured or controlled parameters would be very helpful in characterizing the gaseous emissions from open lots. Moreover, there are some emission models available to estimate gaseous emissions from the open lots (Bonifacio et al., Citation2015), the laboratory-scale studies could also be useful to support or contradict the relationships in the existing or upcoming models to estimate gaseous emissions from open lots. Thus, the objectives of this study were to evaluate the effects of relatively high surface velocity (≥0.4 m sec−1) on CO2 and CH4 emissions at ground level of open dairy lots at different air temperatures by a scale model experiment, and to characterize the effect of typical floor types on the emissions.
Materials and methods
Experimental setup
The experimental setup is shown in . It consists of a scale model of open lots with soil or brick floors, a wind tunnel with a preheating system to warm the incoming air to a designed temperature and to provide expected air velocity over the emitting surface, and a measurement system to monitor thermal environment and gas concentrations.
Scale model of open dairy lots
A scale model was built to simulate the open lots with either unpaved soil or brick-paved floors, which are typical types for dairy cattle farms, especially in China. In this study, a wooden box () with a size of 2.2 m long, 0.72 m wide, and 0.15 m deep, filled with compacted soil, was used to simulate the open lots with unpaved soil on a real farm. The soil inside the box was 0.12 m deep and collected from croplands in Denmark. Its bulk density, pH, moisture, total solid (TS), volatile solid (VS), total nitrogen (TN), ammonium nitrogen (NH4+-N), nitrite nitrogen (NO3−-N), and mineral nitrogen (mineral-N) were 2.1 g cm−3, 5.79, 16.7%, 83.3%, 3.7% of TS, 1.05 g kg−1, 13.31 mg kg−1, 3.76 mg kg−1, and 184.4 mg m−2, respectively. For the scale model with a brick-paved floor, commercial red bricks for building construction were paved on the top of the compacted soil (the same height as the unpaved soil floor) inside the box.
Wind tunnel
The wind tunnel was 2 m long, 0.5 m wide, and 0.45 m high, with a working area of 0.7 m2 (1.4 × 0.5 m; ). It is a standardized device of gaseous emission for field measurements in Denmark, which is also commercially available. The wind tunnel is made by stainless steel and wrapped with timber on the outside. The base was 0.03 m in height and integrated with the wind tunnel at the working area. After manure deposition, the wind tunnel was inserted into the emitting layer and sealed by the manure to monitor emissions. To achieve expected surface velocities at the working area and to get a better gas concentration difference between inlet and outlet, a ceiling was built inside the wind tunnel to leave a 0.03 m high headspace above the working area (). A mesh of cotton fiber (1 cm thick) was installed at the inlet of the wind tunnel to get relatively uniform air velocities over the working area. Airflow going through the wind tunnel was driven by a suction fan with a maximum airflow rate of 160 m3 hr−1 (RS 160; Ruck Ventilatoren, Boxberg, Germany), and its frequency was adjusted by a controller to achieve the desired air velocity above the floor surface. The outlet of the suction fan was connected to the air exhaust chamber of the laboratory, where the experiment was conducted, to avoid exhausted air returning to contaminate the incoming air of the wind tunnel. A preheating system, which was composed of electrical heating rods and a mixing fan, was set at the inlet of the wind tunnel to warm up the incoming air to the desired air temperatures. The power of the electrical heating rods was adjusted from 0 to 10 kW.
Experimental treatment
Impacts of different surface air velocities and floor types on CO2 and CH4 emissions from the scale model of the open lots were examined at different air temperatures. The examined air temperatures (15, 25, and 35 °C), surface velocities (0.4, 0.7, 1.0, and 1.2 m sec−1), and floor types (soil or brick floors) are summarized in . Ambient air temperatures of the laboratory were controlled at 15–16 °C during all experiments.
Table 1. Examined floor types, air temperatures (Tair), and surface air velocities in the study.
The surveyed ranges of the environmental factors were chosen based on the air temperatures and probabilities of wind speeds obtained in the field measurement on the open dairy lots from spring to autumn by the authors (Ding et al., Citation2016). About 61–86% of the observed wind speeds were lower than 1.2 m sec−1 and over 50% were in the range of 0.4–1.2 m sec−1 during the field measurements (Ding et al., Citation2016).
A total of twelve 24-hr runs () were performed, and each test was replicated three times during the experiment. Each experimental run was conducted at one air temperature and two surface velocities. For the first 12 hr of a 24-hr run, during which the surface air velocity was controlled at the speed to be tested, the system and emission were assumed in a stabilizing stage; thus, the data on the gaseous emission were abandoned. In the second 12 hr, gaseous concentrations were monitored at one temperature and two surface air velocities, and the data were applied to quantify gaseous emissions from the scale model. Taking the run at 15 °C, for example, the system was operated for 6 hr at the air velocity of 0.4 m sec−1, connected by another 6 hr under the velocity of 0.7 m sec−1. The sequence of tested air velocities was alternated in three replicates.
Urine and feces for scale model experiment
Prior to the experiments, the surface of the soil or brick floors was fouled with dairy slurry for 2 months at room temperature (15 °C) to promote the development of urease or microbes activities, which was to create a similar condition as a practical lot with manure. The old slurry on the surface was removed and replaced with new once a week. Slurry applied on the floor surface was collected from the research farm of Aarhus University in Foulum, Denmark (AU Foulum), where 110 lactating cows were housed and fed with normal ration (68.39% silage, 18.86% rolled barley, 2.57% rape seed, 8.57% soybean meal, 0.93% minerals, 0.47% urea, and 0.21% supplement).
In each run, one dose of feces (15 kg) and urine (1.5 L) was successively applied to the floor to form a gaseous emitting layer, which was about 3 cm in thickness, and then the emission was continuously monitored for 24 hr. The old layer on the surface was removed and replaced with new manure once a day to simulate the practical management of scraping the manure from the lots. Fresh urine and feces for the runs were collected separately per week from a subgroup of 28 lactating cows on the same farm. To avoid unnecessary chemical and biological changes of the manure, newly collected urine and feces was kept in refrigerators at 4 °C separately (Wang et al., Citation2006) in small doses of 15 kg feces and 1.5 L urine. Before being applied to the floor surface, urine and feces used for the experiments were conditioned at the room temperature (15 °C) for 8 hr to help with the recovery of temperature. Characteristics of the urine and feces used in the experiments were tested and given in .
Table 2. Characteristics of feces and urine collected from lactating cows (N = 6) at the research farm of Aarhus University.
Measurements
Gaseous concentrations and emissions
As shown in , gas concentrations (CO2 and CH4) of the incoming and exhausted air were continuously monitored at the inlet and outlet of the tunnel, respectively, by a photoacoustic multigas monitor (INNOVA 1412i; LumaSense Technologies A/S, Santa Clara, CA, USA) and a multiplexer (type 1309; LumaSense Technologies). The detection limit and accuracy of the analyzer was 1.50 and 1.70 ppm for CO2, and 0.40 and 0.15 ppm for CH4, respectively. Gas concentrations were tested by the analyzer at an interval of 1 min. Every sampling lasted for 40 sec, which was followed by a 20-sec air flushing of the testing chamber. The multiplexer switched the measurements between the incoming and outgoing air every 5 min. The results of the last 3-min measurements were averaged to calculate gas concentration differences between the inlet and outlet air. Gaseous emission rates were estimated by using the airflow rates and the measured concentration differences.
Surface air velocity and airflow rate
Airflow rate through the wind tunnel was monitored by a flow meter using the orifice-pressure deferring principle (DIRU 160; Lindab, Haderslev, Denmark). Maximum airflow rate and accuracy of the detection were 60 L sec−1 and 5%. Pressure differences between the upstream and downstream sides of the orifice were measured by a TSI velocity meter, which was integrated with a differential pressure transmitter, a hot wire anemometer, as well as an air temperature and relative humidity sensor (model 9565 with a sensor probe 964; TSI, Shoreview, MN, USA). Measurement ranges of differential pressure, velocity, temperature, and humidity were 0–300 Pa, 0–50 m sec−1, −10–60 °C, and 5–95% relative humidity (RH), respectively; and the accuracies were ±0.7%, ±0.015 m sec−1, ±0.3 °C, and ±3% RH, respectively. Airflow rate of the wind tunnel was calculated using the equation given by the manufacturer:
where q is the airflow rate (L sec−1) and Δp is the pressure difference between upstream and downstream of the orifice (Pa).
Actual surface air velocities were logged with the same TSI velocity meter (model 9565 with a sensor probe 964), and the probe was set at a height of 2 cm above the surface in the headspace close to the tunnel outlet side (). Surface velocity and differential pressure data were automatically recorded every 30 sec.
Temperature and humidity
Temperature (Tair) and relative humidity (RH) of outgoing air was monitored at the same surface velocity measurement position by using the same integrated instrument (model 9565 with a sensor probe 964; TSI) at an interval of 1 min. Air temperatures of the working area was measured by T-type thermocouples located 3 cm above the surface at an interval of 5 min at five different positions ().
Manure temperature
Manure temperature (Tmanure) was detected and logged every 5 min by a T-type thermocouple, embedded about 2 cm deep inside the manure ().
Statistical analyses
SPSS (version 20.0; IBM, Armonk, NY, USA) was used for statistical analyses. According to the normality test, CO2 and CH4 emissions from the scale model were not normally distributed at varied air temperature and velocity, and thus log10 transformation was applied. Repeated-measures of analysis of variance (ANOVA) was carried out to analyze the impacts of air temperature, velocity, and floor type on gaseous emissions. Statistical significance was based on P < 0.05. As mentioned, only the gaseous emission data in the second 12 hr of each run were analyzed.
Results were expressed as mean ± SD. Gaseous emission rates were expressed as mg CO2 or CH4 per kg fresh manure per hour (mg kg−1 hr−1) due to the fact that emissions were related to the amount of manure organic matters, except for the section of “Impacts of floor type on CO2 and CH4 emissions.” In that section, comparisons of gaseous emissions were made before and after the manure was scraped. So the emission rates were expressed as g per m2 per hour (g m−2 hr−1) when the fresh manure weight was 15 kg.
Results and discussion
Air and manure temperatures during experiments
Air temperature (Tair) and relative humidity (RH) of different experimental runs are shown in .As it flowed through the scale model, incoming air was cooled down mainly by the heat loss through conduction/convection with the cooler manure and wind tunnel enclosure.
Table 3. Temperatures (Tout) and relative humidity (RH) of the air leaving the tunnel and manure temperatures (Tman) of the runs under designed incoming air temperatures (Tin) on the soil and brick floors.
Manure temperature (Tman) is documented as an important factor on gas production from manure (Hashimoto et al., Citation1981, Citation1982; Husted et al., Citation1994; Pereira et al., Citation2011, Citation2012). Tman of the experiments are given in . The logged Tman at the working area inside the scale model increased during the first 2–3 hr after the manure application on the floor surface due to heat transfer from the air ()and then was kept relatively stable when a general dynamic balance was established. gives an example of the Tman on the brick floor at various Tair during experiments.
Figure 2. Manure temperature (Tman) during experiments. (a) An example of Tman changes under different incoming air temperatures on brick floor. (b) Linear regression of Tman and Tair data collected on the brick floor experiment.
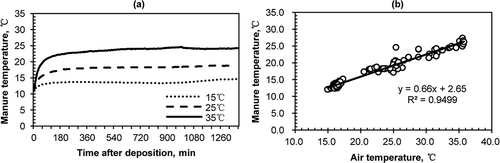
Temperature of the manure on open lots generally follows that of the ambient air, and it is also affected by solar radiation (Wilhoit et al., Citation1991; Armitage, Citation2007; Ding et al., Citation2016). In our study, Tman on the scale model was lower than Tair when the laboratory temperature was controlled at 15–16 °C, and it was found to be linearly related to temperature of the air leaving the wind tunnel (P < 0.05; ).
Impacts of temperatures on CO2 and CH4 emissions
Air temperature is a key factor on driving gas production and emission from animal manure. Generally, the generation process of gases is highly dependent on manure temperature, whereas its transfer and emitting from manure to air would be greatly affected by air temperature above the surface (Arogoet al., Citation1999; Ni, Citation1999). Thus, measured gaseous emissions to the air are normally an integrated effect of both air and manure temperatures. Results showed that CO2 and CH4 emissions significantly increased as air temperature increased (P < 0.05; ). This is in agreement with other reports from experiments on concrete or slatted floors (Pereira et al., Citation2011, Citation2012). Kelly et al. (Citation1981) and Husted (Citation1994) used Q10, which was defined as the ratio of CH4 emissions measured at manure temperatures of (x + 10) °C and x °C, to evaluate the temperature change effect. In our case, Q10 was also calculated at manure temperatures of 25/15 and 35/25 °C. CH4 emissions at manure temperatures of 15, 25, and 35 °C were calculated by plotting the Arrhenius relationship between manure temperatures and gaseous emissions measured at various air temperatures (Castro González, Citation2002).
Figure 3. CO2 and CH4 emissions at different air temperatures (15–35 °C) and surface air velocities (0.4–1.2 m sec−1) from soil and brick floors (N = 3 for each type of floor).
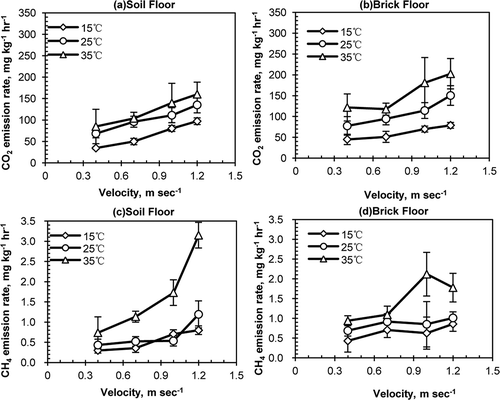
Husted (Citation1994) calculated the Q10 of CH4 emissions from cattle manure storage under different manure temperatures in both laboratory incubations and field measurements. The averaged Q10 was 1.8 for slurry and 2.1 for solid manure in laboratory incubations when Tman ranged from 5 to 35 °C, but 3.4 for slurry and 2.7–4.9 for solid manure from field measurements at the same Tman range. When the incoming air temperature rose from 15 to 35 °C, manure temperatures increased from 11 to 24 °C for the soil floor and from 13 to 25 °C for the brick floor. The calculated Q10 based on manure temperatures at 25/15 °C was 1.9 for the brick floor and 2.0 for the soil floor, respectively, which was close to the reported data in laboratory incubations given by Husted (Citation1994). According to field measurements of an open dairy lot by the authors (Ding et al., Citation2015b, Citation2016), Q10 was 3.2 when manure temperature increased from 15 to 35 °C, which was also comparable to the documented results from the field measurement by Husted (Citation1994). These results showed that Q10 from laboratory-scale tests was much lower than that from field measurements, which may suggest that CH4 emissions from manure on practical open lots might be affected by more factors besides the temperature changes.
Impacts of air velocity on CO2 and CH4 emissions
Surface air velocity was found to have a significant impact on CO2 and CH4 emissions from the scale model of open dairy lots (P < 0.05), and the interactions of air temperature and surface velocity on CH4 emissions from the brick floor was also observed (P < 0.05). Generally, CO2 and CH4 emissions were enhanced with the increasing surface air velocity (). This could be explained by the theory of gases emitting from solutions, in which mass transfer coefficient increases with surface air velocity and consequently enhances the emissions (Arogo et al., Citation1999; Ni et al., Citation1999; Ye et al., Citation2008; Saha et al., Citation2010).
According to Arogo et al. (Citation1999), NH3 emissions were more sensitive to the changes at lower air velocity. Unlike the findings on NH3, CO2 emissions in this study were more sensitive to a relatively higher range of surface velocity from 0.7 to 1.0 m sec−1. The data showed that CO2 emissions from soil floor at 15 °C increased by 45%, 61%, and 21% when surface velocity was raised from 0.4 to 0.7 m sec−1, from 0.7 to 1.0 m sec−1, and from 1.0 to 1.2 m sec−1, respectively, whereas the brick floor increases were 14%, 37%, and 13%, respectively.
With varied surface velocity, CH4 emissions were characterized differently for the soil and brick floors. As surface velocity increased, CH4 emission from the brick floor increased under the temperatures of 15 and 25 °C, and then the flux slightly dropped before the velocity reached 1.0 m sec−1, and afterwards it increased again when surface velocity kept increasing (). On the other hand, at incoming air temperature of 35 °C, CH4 emission decreased after reaching the peak at surface velocity of 1.0 m sec−1. No similar trend was found on CH4 emission from the soil floor (), which kept increasing from the lower to the higher surface air velocities. Moreover, CH4 emission from the soil floor was more sensitive to the velocity changes at higher levels (1.0–1.2 m sec−1), whereas the brick floor trend occurred at lower velocities (0.4–0.7 m sec−1).
Impacts of floor type on CO2 and CH4 emissions
CO2 emissions
Average CO2 emissions from the scale model of an open dairy lot ranged from 34.4 to 160.1 mg kg−1 hr−1 for an unpaved soil floor, and the rates were within 44.9 to 202.6 mg kg−1 hr−1 for a brick-paved floor at various air temperature and velocity. The highest emission occurred at the highest air temperature (35 °C) and the highest air velocity (1.2 m sec−1), whereas the lowest emission was at the lowest air temperature (15 °C) and air velocity (0.4 m sec−1), for both the soil and brick floors. Besides the joint effects of air temperature and surface air velocity, CO2 emissions from the manure on the model were also affected by floor types. CO2 emissions from soil and brick floors at various air velocities were analyzed by paired t test at 15, 25, and 35 °C, respectively. No significant differences in CO2 emissions from the soil and brick floors were found at 15 and 25 °C (P > 0.05), whereas the emissions from the brick floor were higher at 35 °C (; P < 0.05).
Figure 4. Comparisons of CO2 emissions from different floor types under different air temperatures and surface air velocity (N = 3).
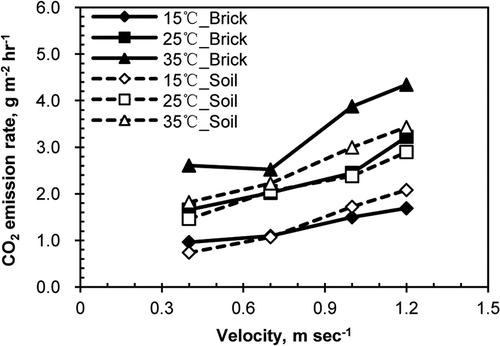
CO2 is generated in the process of urea hydrolysis and organic matter degradation of animal manure (Ni et al., Citation1999b; Møller et al., Citation2004). Compared with the brick-paved floor, it was observed that more liquid leached into the soil floor, which retained more soluble nutrient inside () and may retard the total CO2 production and emitting, particularly under higher air temperatures. It might partially explain why the CO2 emission from the brick floor was significantly higher at 35 °C.
Table 4. Characteristics of the soil packed inside the wooden box of the scale model in the beginning and end of the experiments (top 5 cm soil).
Besides the production from the waste, the floor itself can also be an important CO2 emission source, especially the soil floor. After the removal of manure from the scale model, CO2 emissions from the soil and brick floors were still observed at the surface air velocity of 0.4 m sec−1 (). For a practical lot, the “soil” is typically a mixture of urine, feces, and clay, making it an important source of CO2 emission even though the top manure was cleaned (Kissinger et al., 2006). Compared with the brick floor, CO2 emissions from the soil floor after manure removal were much higher (P < 0.05; ), which may be due to the fact that more urine and feces remained on the “soil.” This suggests a big contribution of the “soil” on CO2 emission under the floor surface after manure removal.
Figure 5. An example of CO2 emissions from the soil and brick floors after manure was scraped when air velocity was at 0.4 m sec−1.
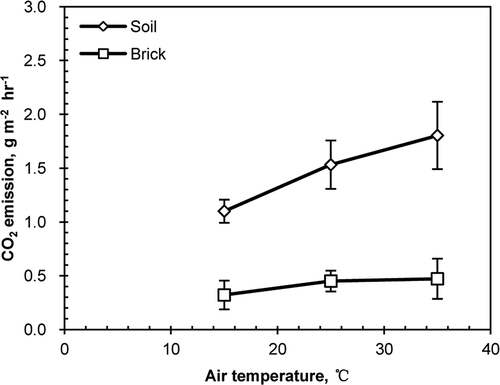
Characteristics of the soil packed inside the wooden box of the scale model in the beginning and ending of the experiments are shown in . At the end of the experiments, moisture content and total nitrogen in the top 5 cm soil increased by 9.9% and 89.3%, respectively, suggesting that water and some nutrients were retained in the soil after the liquid fraction of the manure infiltrated in. The infiltration of soluble nutrients may lead to a delayed CO2 emission from the soil after the manure was scraped and resulted in lower CO2 emissions measured from the manure as indicated above.
CH4 emissions
The CH4 emission sources on the soil and brick floors were somewhat different. Even after the manure was scraped away from the floor surface, some mixture of urine, feces, and clay still remained, especially for the soil floor. The soil underneath could be either an emission source or a sink of CH4 (Topp and Pattey, Citation1997; Le Mer and Roger, Citation2001). This makes the CH4 emission from the open lot with a soil floor to be much more complex.
Generally, methane uptake negatively associates with soil moisture (Luo et al., Citation2013). Nearly no CH4 is consumed by soil with a moisture content higher than 33%, and then the soil becomes an emission source if the moisture keeps increasing (Yonemura et al., Citation2000). Moisture content of the soil floor (top 5 cm) for the scale model ranged from 12.7% to 22.6% during the experiments. Thus, it was determined that the soil was not a CH4 emission source in this case, judged by the finding of Yonemura et al. (Citation2000). If the open lots are flooded, especially during rainy days, the soil floor may contribute more CH4 emission.
Average CH4 emissions measured from the scale model ranged from 0.3 to 3.2 mg kg−1 hr−1 for the unpaved soil floor and were from 0.4 to 2.1 mg kg−1 hr−1 for the brick-paved floor, at various air temperatures and surface air velocities. The lowest emission was measured at the lowest air temperature (15 °C) and air velocity (0.4 m sec−1) as well, for both the soil and brick floors, whereas the highest were at 35 °C and 1.2 m sec−1 for the soil floor and 35 °C and 1.0 m sec−1 for the brick floor.
Although the brick and soil floors showed different patterns on CH4 emission at various air temperatures or velocities (), statistical analysis did not show a significant difference in CH4 emissions between the soil and brick floors (P > 0.05). This is similar to the results observed from solid floor and slatted floors by Pereira et al. (Citation2011). However, the soil floor may have more CH4 emission when it is flooded, i.e., after a long-term use or with precipitation.
Other effects of floor types
Besides the gaseous emissions, the floor system in dairy production operations may also affect cow performance and manure management (Braam et al., Citation1997; Boyle et al., 2006). Compared with the soil or other floors with soft materials, studies showed that the lameness of dairy cows increased and lying behavior was altered as well when the open lots were equipped with brick floors (Cong et al., Citation2012; Hu et al., Citation2014). However, in terms of management practices, manure on a brick floor is much easier to remove. According to field surveys in China by the authors, manure on the farms with brick floors is usually collected from days to weeks. However, manure is typically kept on the soil floors from weeks to months for a much longer period, which may potentially cause more gaseous emissions and nutrient loss. Studies are needed to assess the long-term effects of different floor types and management on gaseous emissions and other environmental issues.
Relationship between CO2 and CH4 emissions
Hydrolysis, acidogenesis, acetogenesis/dehydrogenation, and methanation are the four major phases in the biogenic methane formation process of animal manure. In the acidogenesis and acetogenesis/dehydrogenation processes, acetic acid, H2, as well as CO2 are produced through microbiological or enzymatic conversion, which provide substrates for the two groups of methanogenic bacteria to produce CH4 by either the decomposition of acetate acid or the composition through CO2 and H2 (Whiticar et al., Citation1986; Weiland, Citation2010; Yu et al., Citation2013). Acetate acid decomposition is regarded as the major source of CH4 production in manure storage, whereas the composition of CO2 and H2 is the primary methane source in bovine rumen (Whiticar et al., Citation1986). CO2 and CH4 concentrations from animals are found to be linearly related in naturally ventilated dairy barns. The application of the ratio CO2/CH4 is suggested to be a potential way to quantify methane production if CO2 generation is easier to acquire (Madsen et al., Citation2010; Bjerg et al., Citation2012; Rong et al., Citation2014). If there is a similar relationship between CO2 and CH4 emissions from manure, the ratio would also be useful to estimate CH4 emission in manure management. However, it has not been positively verified for the emissions from manure on the open lots.
From the scale model experiment in this study, the CH4 emission was found to be a function of CO2 flux (P < 0.05; ). Different from the linearly related concentrations from animals, an exponential relationship was found between CO2 and CH4 emissions from the scale model of the open lots, especially for the soil floor experiment ().
Figure 6. Exponential relationships between CO2 and CH4 emissions from soil floor (a) and brick floor (b) at varied air temperatures and velocities.
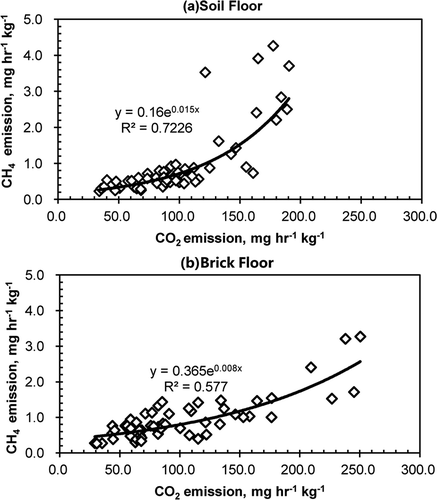
In the biogenic pathway of CH4, studies on carbon isotope exchange equilibria between CH4 and CO2 showed that the ratio was temperature dependent (Whiticar et al., Citation1986) and the relationship could be described as
where T is temperature in Kelvin, and ac is the equal carbon isotopic fractionation (δ13C) between CH4 and CO2. Equations 2 and 3 suggest that the relationship between CH4 and CO2 in a methane biogenic pathway is linear at a stable temperature, whereas it should be exponential at various temperatures.
Gaseous emissions were measured at various temperatures and velocities, and the exponential relationship of CO2 and CH4 emissions observed in this study was in agreement with the theoretical analysis from eqs 2 and 3. Different emission sources might result in the difference. In previous investigations (Madsen et al., Citation2010; Bjerg et al., Citation2012; Rong et al., Citation2014), the measurements covered all the CO2 and CH4 sources, including animals, feed storage, and manure management, etc. Especially for the emission from cows, it is usually considered an important contributor. However, in this study, the emission is primarily from the manure, even though the soil may have some contribution.
As analyzed, CO2 emissions in this scale model experiment come from urea hydrolysis, a microbial reaction in the manure and the floor. No statistical difference was found in CH4 emissions from different floor types, whereas higher CO2 emissions were observed from the brick floor at 35 °C due to manure infiltration. Gaseous emissions from the soil floor showed a stronger exponential regression and a higher coefficient ().
Summary
Influences of air temperature, surface velocity, and floor type on CO2 and CH4 emissions from the manure of open dairy lots were examined by a scale model experiment in this study. Higher air temperature and/or surface air velocity significantly enhanced both the CO2 and CH4 emissions from the manure on both the soil and brick floors (P < 0.05). CO2 emissions from the brick floor were higher than the soil floor at 35 °C, whereas statistical analyses didn’t show a significant difference in CH4 emission from different types of floors. CH4 emissions from the ground level of open lots were found to be exponentially dependent on the CO2 flux. The findings are expected to provide some fundamental information for decision-making as well as further studies on gaseous emissions from open lots.
Acknowledgment
The authors thank especially all the help from the Air Physics Lab and Biogas Lab in Aarhus University, Foulum, Denmark.
Funding
This study was funded by National High Technology Research and Development Program (2013AA10230602), National Nature Science Foundation of China (31172244), and China Agricultural Research System (CARS-37).
Additional information
Funding
Notes on contributors
Luyu Ding
Luyu Ding is a Ph.D. student in the Department of Agricultural Structure and Bioenvironmental Engineering, College of Water Resources and Civil Engineering, China Agricultural University, Beijing, People’s Republic of China.
Wei Cao
Wei Cao, Zhengxiang Shi, and Baoming Li are professors in the Department of Agricultural Structure and Bioenvironmental Engineering, College of Water Resources and Civil Engineering, China Agricultural University, Beijing, People’s Republic of China.
Zhengxiang Shi
Wei Cao, Zhengxiang Shi, and Baoming Li are professors in the Department of Agricultural Structure and Bioenvironmental Engineering, College of Water Resources and Civil Engineering, China Agricultural University, Beijing, People’s Republic of China.
Baoming Li
Wei Cao, Zhengxiang Shi, and Baoming Li are professors in the Department of Agricultural Structure and Bioenvironmental Engineering, College of Water Resources and Civil Engineering, China Agricultural University, Beijing, People’s Republic of China.
Chaoyuan Wang
Chaoyuan Wang is an associate professor in the Department of Agricultural Structure and Bioenvironmental Engineering, College of Water Resources and Civil Engineering, China Agricultural University, Beijing, People’s Republic of China.
Guoqiang Zhang
Guoqiang Zhang is a senior scientist in the Department of Engineering, Aarhus University, Tjele, Denmark.
Simon Kristensen
Simon Kristensen is a technician in the Department of Engineering, Aarhus University, Tjele, Denmark.
References
- Arogo, J., R.H. Zhang, G.L. Riskowski, L.L. Chiristianson, and D.L. Day. 1999. Mass transfer coefficient of ammonia in liquid swine manure and aqueous solutions. J. Agric. Eng. Res. 73:77–86. doi:10.1006/jaer.1998.0390
- Armitage, D.M. 2007. Environment of deep-pit poultry houses: Survey of air and manure temperatures in British houses. Br. Poultry Sci. 26:275–280. doi:10.1080/00071668508416813
- Bjerg, B., G. Zhang, J. Madsen, and H.B. Rom. 2012. Methane emission from naturally ventilated livestock buildings can be determined from gas concentration measurements. Environ. Monit. Assess. 184:5989–6000. doi:10.1007/s10661-011-2397-8
- Bjorneberg, D.L., A.B. Leytem, D.T. Westermann, P.R. Griffith, L. Shao, and M.G. Pollard. 2009. Measurement of atmospheric ammonia, methane, and nitrous oxide at a concentrated dairy production facility in Southern Idaho using open-path FTIR spectrometry. Trans. ASABE 52:1749–1756. doi:10.13031/2013.29137
- Bonifacio, H.F., C.A. Rotz, A.B. Leytem, H.M. Waldrip, and R.W. Todd. 2015. Process-based modeling of ammonia and nitrous oxide emissions from open-lot beef and dairy facilities. Trans. ASABE 58:827–846.
- Borhan, M.S., C.S. Capareda, S. Mukhtar, W.B. Faulkner, R. McGee, and C.B. Parnell. 2011a. Determining seasonal greenhouse gas emissions from ground-level area sources in a dairy operation in Central Texas. J. Air Waste Manage. Assoc. 61:786–795. doi:10.3155/1047-3289.61.7.786
- Borhan, M.S., C.S. Capareda, S. Mukhtar, W.B. Faulkner, R. McGee, and C.B. Parnell. 2011b. Greenhouse gas emissions from ground level area sources in dairy and cattle feed yard operations. Atmosphere 2:303–329. doi:10.3390/atmos2030303
- Braam, C. R., J.J.M.H. Ketelaars, and M.C.J. Smits. 1997. Effects of floor design and floor cleaning on ammonia emission from cubicle houses for dairy cows. Neth. J. Agric. Sci. 45:49–64. doi:10.1006/jaer.1997.0215
- Boyle, L.A., J.F. Mee, and P.J. Kiernan. 2007. The effect of rubber versus concrete passageways in cubicle housing on claw health and reproduction of pluriparous dairy cows. Appl. Anim. Behav. Sci. 106:1–12. doi:10.1016/j.applanim.2006.07.011
- Castro González, A. 2002. Arrhenius equation constants and thermodynamic analysis of CH4 and H2S production for the vinasses anaerobic treatment. In Congreso Interamericano de Ingeniería Sanitaria y Ambiental 28, 1–8. Cancun, Mexico, October 27–31, 2002: FEMISCA.
- Chianese, D.S., C.A. Rotz, and T.L. Richard. 2009. Simulation of methane emissions from dairy farms to assess greenhouse gas reduction strategies. Trans. ASABE 52:1313–1323. doi:10.13031/2013.27781
- Cong, H., L. Sha, S. Wang, F. Liu, and C. Wu. 2012. Comparison on the performance of brick and dried cow-dung as floor materials for open dairy lots [in Chinese]. China Dairy Cattle 2012(15):41–43. doi:10.3969/j.issn.1004-4264.2012.15.017
- Ding, L., Q. Lu, C. Wang, Z. Shi, W. Cao, and B. Li. 2015a. Effect of configuration and headspace mixing on the accuracy of closed chambers for dairy farm gas emission measurement. Appl. Eng. Agric. 31:153–162. doi:10.13031/aea.31.10853
- Ding, L., Q. Lu, L. Xie, J. Liu, W. Cao, Z. Shi, B. Li, S. Ren, C. Wang, and G. Zhang. 2015b. Field measurement of carbon dioxide, methane and nitrous oxide emissions from manure stockpile and open exercise yard of a typical dairy farm in China by chamber technique. Presented at 2015 ASABE Annual International Meeting: New Orleans, LA, July 26–29, 2015. Paper number: 152171852.
- Ding, L., Q. Lu, L. Xie, J. Liu, W. Cao, Z. Shi, B. Li, C. Wang, G. Zhang, and S. Ren. 2016. Greenhouse gas emissions from dairy open lot and manure stockpile in northern China: A case study. J. Air Waste Manage. Assoc. 55:267–279. doi:10.1080/10962247.2015.1124058
- Eghball, B., and J.F. Power. 1994. Beef cattle feedlot manure management. J. Soil Water Conserv. 49:113–122.
- Eigenberg, R.A., R.L. Korthals, J.A. Nienaber, and G.L. Hahn. 1998. Implementation of a mass balance approach to predicting nutrient fate of manure from beef cattle feedlots. Appl. Eng. Agric. 14:475–484.
- Gonzalez-Avalos, E., and L.G. Ruiz-Suarez. 2001. Methane emission factors from cattle manure in Mexico. Bioresour. Technol. 80:63–71. doi:10.1016/S0960-8524(01)00052-9
- Hao, X., C. Chang, and F.J. Larney, 2004. Carbon, nitrogen balances and greenhouse gas emission during cattle feedlot manure composting. J. Environ. Qual. 33:37–44. doi:10.2134/jeq2004.0037
- Hashimoto, A.G. 1982. Methane from cattle waste: Effects of temperature, hydraulic retention time, and influent substrate concentration on kinetic parameter (K). Biotechnol. Bioeng. 24:2039–2052. doi:10.1002/(ISSN)1097-0290
- Hashimoto, A.G., V.H. Varel, and Y.R. Chine. 1981. Ultimate methane yield from beef cattle manure: Effect of temperature, ration constituents, antibiotics and manure age. Agric. Wastes 3:241–256. doi:10.1016/0141-4607(81)90011-1
- Hu, B., C. Gu, S. Lu, and D. Guo. 2014. Influence of floor condition on the hoof lesion of dairy cow housed on open lots [in Chinese]. Heilongjiang Anim. Sci. Vet. Med. 2014 (6):42–43. doi:10.13881/j.cnki.hljxmsy.2014.0473
- Husted, S. 1994. Seasonal variation in methane emission from stored slurry and solid manures. J. Environ. Qual. 23:585–592. doi:10.2134/jeq1994.00472425002300030026x
- Kelly, C., and D.P. Chynoweth. 1981. The contributions of temperature and of the input of organic matter in controlling rates of sediment methanogenesis. Limnol. Oceanogr. 26:891–897. doi:10.4319/lo.1981.26.5.0891
- Kissinger, W.F., R.K. Koelsch, G.E. Erickson, and F.J. Klopfenstein. 2007. Characteristics of manure harvested from beef cattle feedlots. Appl. Eng. Agric. 23:357–365. doi:10.13031/2013.22685
- Le Mer, J., and P. Roger. 2001. Production, oxidation, emission and consumption of methane by soils: A review. Eur. J. Soil Biol. 37:25–50. doi:10.1016/S1164-5563(01)01067-6
- Luo, G.J., R. Kiese, B. Wolf, and K. Butterbach-Bahl. 2013. Effects of soil temperature and moisture on methane uptake and nitrous oxide emissions across three different ecosystem types. Biogeosciences 10:3205–3219. doi:10.5194/bg-10-3205-2013
- Madsen, J., B.S. Bjerg, T. Hvelplund, M.R. Weisbjerg, and P. Lund. 2010. Methane and carbon dioxide ratio in excreted air for quantification of the methane production from ruminants. Livestock Sci. 129:223–227. doi:10.1016/j.livsci.2010.01.001
- Monteny, G.J., A. Bannink, and D. Chadwick. 2006. Greenhouse gas abatement strategies for animal husbandry. Agric. Ecosyst. Environ. 112:163–170. doi:10.1016/j.agee.2005.08.015
- Møller, H.B., S.G. Sommer, and B.K. Ahring. 2004. Biological degradation and greenhouse gas emissions during pre-storage of liquid animal manure. J. Environ. Qual. 33:27–36. doi:10.2134/jeq2004.2700
- Mukhtar, S., A. Mutlu, S.C. Capareda, and C.B. Parnell. 2008. Seasonal and spatial variations of ammonia emissions from an open-lot dairy operation. J. Air Waste Manage. Assoc. 58:369–376. doi:10.3155/1047-3289.58.3.369
- Ni, J. 1999. Mechanistic models of ammonia release from liquid manure: A review. J. Agric. Eng. Res. 72:1–17. doi:10.1006/jaer.1998.0342
- Ni, J., C. Vinckier, J. Hendriks, and J. Coenegrachts. 1999. Production of carbon dioxide in a fattening pig house under field conditions. II. Release from the manure. Atmos. Environ. 33:3697–3703. doi:10.1016/S1352-2310(99)00128-4
- Owen, J.J., and W.L. Silver. 2012. Greenhouse gas emissions from dairy manure management: A review of field-based studies. Glob. Change Biol. 21:550–565. doi:10.1111/gcb.12687
- Pereira, J., D. Fangueiro, T.H. Misselbrook, J. Coutinho, and H. Trindade. 2011. Ammonia and greenhouse gas emissions from slatted and solid floors in dairy cattle houses: A scale model study. Biosyst. Eng. 109:148–157. doi:10.1016/j.biosystemseng.2011.02.011
- Pereira, J., T.H. Misselbrook, D.R. Chadwick, J. Coutinho, and H. Trindade. 2012. Effects of temperature and dairy cattle excreta characteristics on potential ammonia and greenhouse gas emissions from housing: A laboratory study. Biosyst. Eng. 112:138–150. doi:10.1016/j.biosystemseng.2012.03.011
- Rong, L., D. Liu, E.F. Pedersen, and G. Zhang. 2014. Effect of climate parameters on air exchange rate and ammonia and methane emissions from a hybrid ventilated dairy cow building. Energy Build. 82:632–643. doi:10.1016/j.enbuild.2014.07.089
- Rotz, S.K., H. Georg, S. Godbout, and H.F.A. Van den Weghe. 2010. Impact of the manure removal from slatted floor in a dairy barn on the ammonia emission. Presented at the XVIIth World Congress of the International Commission of Agricultural and Biosystems Engineering (CIGR), Québec City, Canada, June 13–17, 2010.
- Saha, C.K., G. Zhang, and J. Ni. 2010. Airflow and concentration characterization and ammonia mass transfer modelling in wind tunnel studies. Biosys. Eng. 107:328–340. doi:10.1016/j.biosystemseng.2010.09.007
- Topp, E., and E. Pattey. 1997. Soils as sources and sinks for atmospheric methane. Can. J. Soil Sci. 77:167–177. doi:10.4141/S96-107
- U.S. Environmental Protection Agency. 2012. Summary Report: Global Anthropogenic Non-CO2 Greenhouse Gas Emissions: 1990–2030. Washington, DC: Office of Atmospheric Programs, Climate Change Division, U.S. Environmental Protection Agency.
- Wang, C., B. Li, G. Zhang, H.B. Rom, and J.S. Strøm. 2006. Model estimation and measurement of ammonia emission from naturally ventilated dairy cattle buildings with slatted floor designs. J. Air Waste Manage. Assoc. 56:1252–1259. doi:10.1080/10473289.2006.10465678
- Weiland, P. 2010. Biogas production: Current state and perspectives. Appl. Microbiol. Biotechnol. 85:849–860. doi:10.1007/s00253-009-2246-7
- Whiticar, M.J., E. Faber, and M. Schoell. 1986. Biogenic methane formation in marine and freshwater environments: CO2 reduction vs. acetate fermentation—isotope evidence. Geochim. Cosmochim. Acta 50:693–709. doi:10.1016/0016-7037(86)90346-7
- Wilhoit, L.R., R.C. Axtell, R.E. Stinner, L.R. Wilhoit, R.C. Axtell, and R.E. Stinner. 1991. Estimating manure temperatures from air temperatures and results of its use in models of filth fly (Diptera: Muscidae) development. Environ. Entomol. 20:635–643. doi:10.1093/ee/20.2.635
- Ye, Z., G. Zhang, B. Li, J.S. Srøm, G. Tong, and P.J. Dahl. 2008. Influence of airflow and liquid properties on the mass transfer coefficient of ammonia in aqueous solutions. Biosyst. Eng. 100:422–434. doi:10.1016/j.biosystemseng.2008.04.016
- Yonemura, S., S. Kawashima, and H. Tsuruta. 2000. Carbon monoxide, hydrogen, and methane uptake by soils in a temperate arable field and a forest. J. Geophys. Res. Atmos. 105(D11):14347–14362. doi:10.1029/1999JD901156
- Yu, L., P.C. Wensel, J.W. Ma, and S.L. Chen. 2013. Mathematical modeling in anaerobic digestion (ad). J. Bioremediat. Biodegrad. 5(S4):S4–003. doi:10.4172/2155-6199