ABSTRACT
This study aims to develop a high-efficiency radical oxidation process for enhancing the dewaterability of waste activated sludge (WAS). Radical scavenging studies combined with electron paramagnetic resonance (EPR) were carried out for the direct radical identification and effectiveness evaluation of radical oxidation. The results indicated that Fe(II)-activated CaO2 can pose a superior effect on dewatering WAS due to its distinctive capacity of stable •OH production and the high reaction efficiency of regulated-released •OH with water-holding organics. The mechanism for the enhanced dewatering performance was also explored. The rupture of sludge colloidal flocs and the reduction of hydrophilic functional groups in loosely bound extracellular polymeric substances (LB-EPS) were found to be mainly responsible for the release of interstitial water and improved dewaterability, respectively. In addition, an inference about the relationship between interfacial water and zeta potential of different EPS fractions was established by the simultaneous measurement of the binding affinities of Ca2+ and Fe2+/Fe3+ for EPS and bound water content. All these results provide the direct evidence that Fe(II)-activated CaO2 is a promising pretreatment reagent for sludge disposal.
Implications: Fe(II)-activated CaO2 was first proposed to be highly effective in enhancing the dewaterability of waste activated sludge. Electron paramagnetic resonance (EPR) spectroscopy provided the direct evidence for the specific advantages of CaO2, especially the capacity of durable and efficacious •OH production leading to the excellent conditioning performance.
Introduction
Waste activated sludge (WAS), a by-product of biological wastewater treatment that contains various toxic substances such as pathogens, heavy metals, and some organic contaminants, is a source of serious environmental pollution (Yang et al., Citation2015). The generation of WAS has increased rapidly due to the accelerated economic development and urbanization in some developing countries, especially in China. Dewatering, which reduces volume, facilitates transport, and increases calorific value, is usually the primary step in WAS energy utilization and final disposal. However, the complex colloidal nature and strong hydrophilicity of WAS substantially hampers its dewaterability in the absence of pretreatment (Zhen et al., Citation2012c). In this regard, many conditioning approaches, such as coagulation, ultrasonication (Feng et al., Citation2009), microwave irradiation (Yu et al., Citation2009), freezing and thawing (Diak et al., Citation2011), and electro-dewatering (Mahmoud et al., Citation2010), have been developed. Although these methods have some potential benefits of sludge dewaterability, their application has been limited by many factors, including sludge volume increase, large consumption of energy, and complexity of implementation. Therefore, more efficient dewatering methods are still desiderated.
Advanced oxidation processes (AOPs) have been gaining attention due to the positive effects on eliminating the negative effects of WAS colloidal structure on dewatering. Fenton or Fenton-like processes and ozone are the most widely used AOPs for improving the dewaterability of WAS from different sources (Neyens and Baeyens, Citation2003; Tony et al., Citation2008; Yu et al., Citation2016; Zhang et al., Citation2014). However, the liquid or gas states of these conventional AOP reagents and the rapid decline of radicals limit their transportation and application (Pignatello et al., Citation2006). The existing researches mainly focus on the effects of pH or radical oxidation on the surface properties of organic phase and the migration and transformation of bound water in WAS (Zhang et al., Citation2014, Citation2015b; Yu et al., Citation2016). The inherent defects of liquid Fenton reagent applied in complex organic systems still need to be overcome further. Calcium peroxide (CaO2) is one of the most versatile and safest solid inorganic peroxy compounds and can be considered a “solid form” of hydrogen peroxide (H2O2) (Qian et al., Citation2013). In its natural state, CaO2 can release H2O2 slowly and maintain a stable oxidation capacity when dissolved in water (Ai et al., Citation2015). In addition, extracellular polymeric substances (EPS), various biochemicals secreted by microbes and release of cellular material products, constitute a significant fraction of sludge mass and play a key role in binding a large volume of water (i.e., bound water) (Mikkelsen and Keiding, Citation2002; Liu and Fang, Citation2003; Sheng et al., Citation2010; Flemming and Wingender, Citation2001). Especially, the negatively charged bound EPS network plays a significant role in maintaining hydrated sludge structure and could effectively prevent the release of water (He et al., Citation2015). Ca2+ shows a high affinity for binding with EPS that can aggregate WAS and thus further promote dewaterability (Arabi and Nakhla, Citation2007). There are multiple reports in the literature on the addition of CaO2 into saturated soil and ground water to accelerate the mineralization of recalcitrant organic pollutants, such as petroleum hydrocarbons and tetrachloroethene (Ndjou’Ou and Cassidy, Citation2006; Northup and Cassidy, Citation2008), whereas few are available on applying CaO2 to enhance the dewaterability of WAS. Moreover, the previous studies have mainly focused on the optimization of pretreatment condition, but the specific advantages of CaO2 compared with conventional Fenton reagent, especially the capacity of durable and efficacious •OH production, still lack direct experimental evidence to date.
The feasibility and potential advantage of CaO2 activated by ferrous ions (Fe(II)) in improving the dewatering performance of WAS were investigated in this study. A radical scavenging experiment combined with electron paramagnetic resonance (EPR) spectroscopy was carried out to identify the predominant active radicals directly in the proposed process and to analyze the reaction efficiency of •OH with organics in WAS. The mechanism of enhanced dewaterability was also investigated from the perspectives of WAS micromorphology and the variations in hydrophilic/hydrophobic functional groups of EPS. Furthermore, the effects of the binding affinities of multivalent cations (Ca2+ and Fe2+/Fe3+ introduced by Fe(II)-activated CaO2) for EPS on the interfacial water removal were discussed. These results will help provide novel insights into the development of efficient pretreatment technologies for addressing WAS management.
Materials and methods
Test materials
WAS was obtained from a wastewater treatment plant located in Shanghai, China, that has a design capacity of 150,000 m3/day. The collected sludge was stored at 4 °C before use. All the results were obtained in 5 days, and the results of dosage optimization and pH effect were obtained within 48 hours. Within the experimental period of 5 days, the pre-experiments were conducted to make sure that the basic characteristic of sludge samples didn’t change. The main general characteristics of the concentrated sludge were as follows: pH, 6.56 ± 0.2; water content, 97.95 ± 0.01%; total solid (TS), 20.45 ± 0.14 g/L; volatile solid (VS), 16.33 ± 0.49 g/L; capillary suction time (CST), 154.6 ± 14.7 sec; and specific resistance of filtration (SRF), 2.29 ± 0.06 × 1012 cm/g. All of the chemical reagents were of analytical grade unless otherwise stated.
Experimental procedure
A series of bench-scale experiments were conducted on 300-mL sludge samples in 500-mL beakers to investigate the effects of initial pH, CaO2 dosage, and Fe(II) dosage on WAS dewaterability. Fe(II) solutions (0.5 mol/L) were freshly prepared with FeSO4·7H2O before each daily bench-scale experiment. In each experiment, the reaction began with adjusting the pH of the WAS by addition of 3 M hydrochloric acid (HCl) or 3 M sodium hydroxide (NaOH). Then, the sludge sample was continuously agitated by a constant mechanical stirrer at 300 rpm for 15 min to ensure homogeneity throughout the reaction. During mixing, an appropriate amount of Fe(II) solution was added to the reaction system. Immediately after that, the set dosage of CaO2 was added to the WAS. After 30 min, the sample was collected and analyzed for its dewaterability in terms of CST and SRF.
EPS extraction
Standard procedures for EPS extraction of sludge samples using centrifugation plus ultrasonication were modified based on a method detailed elsewhere (Yu et al., Citation2007). In brief, sludge was first centrifuged at 2000 × g for 15 min at 4 °C; the supernatant was termed soluble EPS (S-EPS). The sludge pellets were then resuspended and diluted to their original volume by adding 0.05% NaCl solution. Then, the mixture was sheared using a vortex mixer (S25; IKA, Königswinter, Germany) for 2 min and then centrifuged at 5000 × g for 15 min at 4 °C. The organic matter in the collected supernatant was termed loosely bound EPS (LB-EPS). Finally, the WAS pellets remaining in the tube were resuspended to the original volume and treated with ultrasonication (40 kHz and 120 W for 2 min). The supernatant collected was termed tightly bound EPS (TB-EPS), and the remaining sediment was the pellet.
Analytical methods
The sludge dewaterability was evaluated in terms of CST and SRF. CST was determined using a standard CST apparatus (type 304B; Triton Electronics Ltd., Essex, UK) with standard chromatography-grade CST paper.
EPR spectra were recorded on a field-modulated CW Bruker ER 200 D SRC EPR spectrometer operating in X-band at room temperature (295 K), and spin adducts were quantified. The sweep width was set to 200 G, and the microwave power was set at 2.016 mW. Modulation frequency and modulation amplitude were set at 100.00 kHz and 1.00 G, respectively. The receiver gain was set to 6.32 × 104. The conversion time and sweep time were set to 40.960 msec and 41.943 sec, respectively.
After the gradient dehydration of raw and pretreated sludge in ethanol-water mixtures with different mass concentrations, the morphological analysis was conducted by scanning electron microscopy (SEM) (Prox; Phenom Company, Eindhoven, The Netherlands) at a working voltage of 10 kV. The sample preparation method is briefly as following: Before the gradient dehydration, the sludge samples were first washed by 0.1 M phosphate buffer and 2.5 wt. % glutaraldehyde solution, respectively. Secondly, after the gravitational settling, the supernatant of sludge was discarded and replaced by the ethanol-water mixtures with the ethanol mass concentrations of 50%, 60%, 70%, 80%, 90%, and 95% successively. Finally, the samples were dried in the vacuum drying oven at 35 °C and the vacuum level of 0.1 MPa.
The measurement of the EPS molecular weight (MW) distribution before and after conditioning was carried out using gel permeation chromatography (GPC) (LC-10ADVP; Shimadzu, Kyoto, Japan) equipped with a differential detector (RID-10A; Shimadzu) according to the previous report (Zhen et al., Citation2014).
Proteins (PN) and polysaccharides (PS) are the major components of EPS (Li and Yang, Citation2007). The compositions of EPS were analyzed by determination of polysaccharide and protein contents using the anthrone method (Yu et al., Citation2007) with glucose as the standard and the Lowry-Folin method (Zhen et al., Citation2012c) with bovine serum albumin (BSA) as the standard, respectively.
The three-dimensional excitation-emission matrix (EEM) fluorescence spectra were measured using a luminescence spectrometer (FluoroMax-4; HORIBA Jobin Yvon Co., Longjumeau, France). EEM spectra with subsequent scanning emission spectra from 250 to 550 nm at 5-nm increments were collected by varying the excitation wavelength from 250 to 400 nm at 5-nm increments. The excitation and emission slits were maintained at 10 nm (Zhen et al., Citation2012c).
The zeta potential of EPS was measured using a zeta potential meter (Zeta sizer Nano Z; Malvern Instruments Ltd., Worcestershire, UK) at the initial pH of the pretreated sludge. If necessary, the pH of the EPS extraction extract was adjusted with a 3 M NaOH solution or a 3 M HCl solution and the ion strength of all the samples was adjusted to the same by 0.05 wt. % NaCl solution. To explore the correlation between the zeta potential of EPS and the metal complexation on the EPS, the concentrations of calcium and iron in the S-EPS, LB-EPS, TB-EPS, and pellet were also analyzed by inductively coupled plasma optical emission spectrometry (ICP-OES; 720ES; Agilent, Santa Clara, CA, USA) after aqua regia and hydrofluoric acid digestion according to the modified standard method (Gilcreas, Citation2012). The inference about the relationship between interfacial water and EPS zeta potential was partially verified by the measurement of bound water in sludge according to the method reported by previous literature (Smith and Vesilind, Citation1995).
Results and discussion
Enhanced dewatering of WAS by Fe(II)-activated CaO2
Effect of addition ratio of Fe(II) and CaO2
In a pre-experiment, CaO2 was applied without Fe(II) under controlled pH values, and the results showed that the acidic condition is beneficial for the dewatering performance. Thus, the following experiment regarding the effect of CaO2 dosage was performed at initial pH 3. As shown in and , the CST decreased from 154.7 to 29.2 sec when the dosage of CaO2 increased from 0 to 0.05 g/g dry solid (DS). However, the CST was not reduced when the dosage of CaO2 increased further. At CaO2 dosages of 0.1 and 0.15 g/g DS, the CST reduction rates (1 − CST of pretreated WAS/initial CST) were only 21.3% and −0.13%, respectively. The SRF showed a similar pattern with the increasing addition of CaO2. After an initial decline to the lowest value of 1.68 × 1012 cm/g, the SRF increased to 4.72 × 1012 cm/g as the CaO2 dosage increased to 0.15 g/g DS. The optimal reductions of CST and SRF were obtained simultaneously at a CaO2 dosage of 0.05 g/g DS. The initial increase in efficiency can be observed at the lower CaO2 dosage. However, the influence of additional CaO2 was less at the given amount of Fe(II), which can be inferred from a lack of initiator, for example, Fe(II). Therefore, the effect of the Fe(II) dosage on dewatering WAS was investigated to determine the optimal ratio of CaO2 and Fe(II).
Figure 1. Performance of Fe(II)-activated CaO2 for enhanced dewatering of WAS. Left: CST; right: SRF. (a, b) Effect of CaO2 dosage on the dewaterability of WAS. (c, d) Effect of Fe(II) dosage on the dewaterability of WAS. (e, f) Effect of initial pH on the dewaterability of WAS.
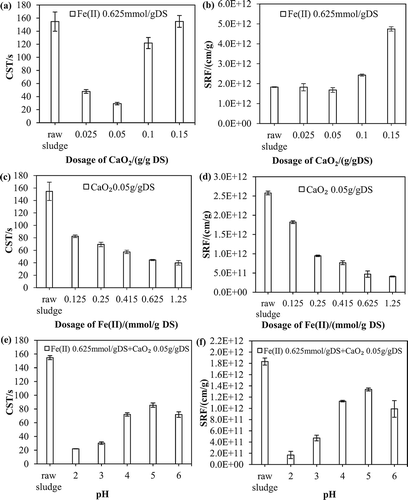
Both the CST and SRF decreased continuously as the applied Fe(II) concentration increased from 0 to 1.25 mmol/g DS in the presence of CaO2 at 0.05 g/g DS (see ). However, as Fe(II) was added over 0.625 mmol/g DS, no continuously substantial reduction in CST or SRF was observed. According to the above results, the optimal addition ratio of Fe(II) and CaO2 is 12.5 mmol/g. And 0.05 g CaO2/g DS dissolved in water liberates a theoretical maximum of 0.0236 g H2O2/g DS according to the following equation (Northup and Cassidy, Citation2008):
The mass ratio of Fe(II) to the theoretical H2O2 yield amount is 1.48, which is in accordance with other previous reports about Fenton reactions (Pignatello et al., Citation2006; Zhen et al., Citation2014).
Effect of initial pH
pH plays a key role in the dissolution of CaO2 and the release rate of H2O2. The effect of initial pH values from 2 to 6 on the dewatering performance of the Fe(II)-activated CaO2 is illustrated in and f. The dosages of CaO2 and Fe(II) were set according to the optimal results of the above section.
As shown in and , the CST and SRF increased rapidly in the initial pH range from 2 to 6, and the lowest CST and SRF values were determined to be 22.1 sec and 1.69 × 1011 cm/g, respectively, at initial pH 2 after 30 min of treatment. The rate of CaO2 dissolution might be different at different pH conditions. At higher initial pH values, the dissolution rate and the efficiency of dewatering decreased. Accordingly, less H2O2 generation resulted in less production of the •OH radical, which may lead to less reduction of CST and SRF. In addition, WAS is a very complicated matrix with various organic and inorganic substances (organic acids, amino acids, polysaccharides, and hydroxamate siderophores), which can be natural chelating agents and effective in sustaining the chemical oxidation of contaminants by modified Fenton reaction (Ndjou’Ou and Cassidy, Citation2006; Northup and Cassidy, Citation2008). pH causes different chelation capacities of organics in WAS. For example, the production rate of •OH may be promoted slightly by the presence of Fe(II) chelated by carboxyl-containing substances in aqueous phase as the pH increased from 5 to 6 (Nam et al., Citation2001). As a result, a slight decrease in CST and SRF was observed.
Comparison of conventional Fenton reagent and Fe(II)-activated CaO2
The CST of WAS pretreated by conventional Fenton reagent and Fe(II)-activated CaO2 at the same addition ratio of Fe(II) and oxidant (12.5 mmol Fe(II)/g CaO2 equivalent) is shown in . The initial pH was adjusted to 2, and the oxidant dosage was converted into CaO2 equivalent according to eq 1. The CST of raw WAS was 148.82 ± 11.59 sec. It can be seen that Fe(II)-activated CaO2 showed a better conditioning performance compared with conventional Fenton reagent at the same dosage of CaO2 equivalent. Furthermore, other reports about WAS dewatering by conventional Fenton reagent indicated that the dosage ranges of H2O2 were usually 0.1~0.3 g/g VS (Tony et al., Citation2008; Zhen et al., Citation2014). When converted into CaO2 equivalent, it is 0.21~0.63 g CaO2 equivalent/g VS, which is much higher than that of Fe(II)-activated CaO2 in this study. Conventional Fenton reagent can also be used with lime to reduce its dosage to 0.05 g/g VS and lead to the reduction of CST approximately by 90% (Liang et al., Citation2015; Yu et al., Citation2016). But the addition of lime causes the increasing volume of dewatered sludge and more complex operation process. Therefore, it can be inferred that the Fe(II)-activated CaO2 has a potential advantage for WAS dewatering.
The most critical limitation of conventional Fenton reagent is the instability of •OH instantaneously produced by liquid H2O2 in a large amount (Ndjou’Ou and Cassidy, Citation2006; Northup and Cassidy, Citation2008). The excess H2O2 could act as a scavenger and compete for •OH to inhibit oxidation of the water-holding organic compound (Muruganandham and Swaminathan, Citation2004). In this study, the release of H2O2 was autoregulated by the rate of CaO2 dissolution, so not all of the H2O2 was available at once as it would be with liquid H2O2 (Northup and Cassidy, Citation2008). As a result, the inhibitory effect and disproportionation of H2O2 catalyzed by various organic substances in WAS can be both reduced. Therefore, the utilization rate of •OH produced by Fe(II)-activated CaO2 was much higher than that produced by liquid H2O2, which would be further verified by EPR spectroscopy as described in the next section.
Contribution of radicals to WAS dewaterability
Identity of radicals by radical scavenging experiment
CaO2 dissolves in water to produce H2O2 as described by eq 1. In the presence of Fe(II) and H2O2, the following reactions proceed to produce •OH and other radicals, including HO2• and O2•− (Pignatello et al., Citation2006):
Trichloromethane (TCM) is an effective scavenger of reductants (O2•− and HO2•), and 2-propanol scavenges •OH (Ndjou’Ou and Cassidy, Citation2006). TCM and 2-propanol were therefore added to separate reactors with the Fe(II)-activated CaO2 to evaluate the contribution of •OH or other factors, O2•− and HO2•, to the observed improvement of WAS dewaterability. The conditioning concentrations were 0.05 g/g DS CaO2 and 0.625 mmol/g DS Fe(II) at an initial pH of 2. 2-Propanol and TCM may influence the viscosity and electrical conductivity of WAS liquid phase, so the addition of 2-propanol and TCM may affect the diffusion of water in CST filter paper and further increase the measured CST. In the identity experiments, raw WAS was first diluted twice. As a result, when the ratio of organic scavengers and TS in WAS was set as a constant value, the proportion of organic scavengers in WAS water can be reduced to minimize the effects of organic scavengers on the raw WAS dewaterability. The results of radical identity experiments were expressed as the variance rate of normalized CST (normalized CST = CST/total solid content in WAS). The experiments on raw WAS only with 2-propanol and TCM were also conducted as reference, respectively.
As shown in , for raw WAS only with 2-propanol or TCM, the variance rate of CST was throughout close to 1, which demonstrated the little effect of scavengers on raw WAS CST. But for WAS pretreated by Fe(II)-activated sludge, the existing of 10 g/g DS 2-propanol significantly decreased the average CST reduction efficiency (1-CST of pretreated WAS/CST of raw WAS) from 74.7% to 53.0%, whereas the addition of 10 g/g DS TCM only resulted in a CST reduction efficiency decrease from 74.7% to 69.7%. Such a difference in the CST reduction efficiency by the two radical scavengers implies a combined action of •OH, O2•−, and HO2• in the Fe(II)-activated CaO2 system, but the mechanism based on •OH is dominant for improving WAS dewaterability. Although eq 8 can also potentially produce •OH from HO2•, but is extremely slow (k = 3 L mol−1·sec−1) and can be neglected (Pignatello et al., Citation2006). To further verify the contribution of •OH, the 2-propanol dosage was increased to 20 g/g DS, and an average decrease of 44.4% in the CST reduction efficiency was observed, whereas it was just 53.0% by the addition of 10 g/g DS 2-propanol. In addition, the maximum CST reduction efficiency can be obtained within the first 5 min, as shown in . The CaO2 oxidation driven by Fe(II) is a rapid and efficient way to enhance WAS dewaterability.
Effectiveness evaluation of radical oxidation by EPR spectroscopy
EPR is a widely applied technique that allows the direct detection of species with unpaired electrons (e.g., free radicals, transition metals). In this study, the identity of radicals and other intermediates in WAS pretreated by conventional Fenton and Fe(II)-activated CaO2 at the same CaO2 equivalent dosage (0.05 g/g DS) was determined by EPR. The Fe(II) dosage was 0.625 mmol/g DS, and the initial pH was adjusted to 2. 5,5-Dimethyl-1-pyrroline-N-oxide (DMPO) was chosen as spin trap. A 1.0 mol/L DMPO solution and the samples were fully mixed with the volume ratio of 1:1 according to previous studies at different reaction times (Zhang et al., Citation2015a). The mixture was then put in a quartz capillary for EPR measurements immediately.
The EPR spectra of radicals with DMPO spin trap in pretreated WAS are shown in . The two spectra are both characterized by four split lines with 1:2:2:1 height ratio and the symmetry center is at g value of 2.005, which is typically representative of the •OH radical added to DMPO (DMPO-OH). That confirms the •OH radical–based mechanism of enhanced WAS dewaterability again. The intensity of the signal is indicated with peak-to-peak height (Ai et al., Citation2015). In the case of H2O2 (), the concentration of •OH decreased significantly with the increasing reaction time. On the contrary, the signal of DMPO-OH remained steady for Fe(II)-activated CaO2 as shown in , which reflects that Fe(II)-activated CaO2 produced •OH continuously and stably during the 10-min conditioning time. Although the stationary EPR relative intensity of DMPO-OH radicals produced by conventional Fenton reagent was higher than that of Fe(II)-activated CaO2, •OH of the former reaction can be scavenged by residual H2O2 (eq 6) (Muruganandham and Swaminathan, Citation2004); as a result, the decay of •OH signal was obvious and accordingly, the utilization rate of •OH would be limited. However, the sustained-release of H2O2 from Fe(II)-activated CaO2 could eliminate its accumulation as •OH scavenger effectively.
Figure 4. EPR spectra of radicals with DMPO spin trap in pretreated WAS: (a) conventional Fenton reagent; (b) Fe(II)-activated CaO2.
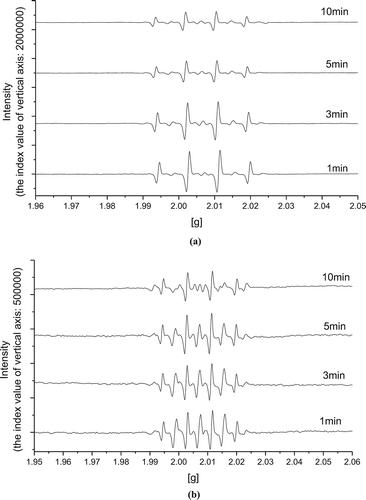
In addition, •OH reacts in well-known ways with organic compounds, principally by abstracting H from C–H, N–H, or O–H bonds to produce C-centered radical R-C• (eq 9) (Pignatello et al., Citation2006).
•C-R is the indicator of reaction between •OH and organic components in WAS. In the presence of DMPO, •C-R can be trapped by the spin trapping agent DMPO, then forming more stable adducts (•DMPO-C-R) (Ai et al., Citation2015). The triplet lines, which are symmetrical near g = 2.005, are typical for the radicals where the unpaired electron is localized on carbon (•DMPO-C-R). For example, it could be , the intermediate of the reaction between •OH and protein-like matters or amino acid in EPS (Lee et al., Citation2002; Pshezhet︠s︡kiĭ, Citation1974; More et al., Citation2014). The largest peak intensities near g value of 2.005 at 1, 3, and 5 min of Fe(II)-activated CaO2 were 229,120, 195,820, and 159,450, respectively, and all were much higher than those of conventional Fenton reagent (the corresponding peak heights at 1, 3, and 5 min were 44,655, 149,220, and 106,050, respectively). This difference clearly indicated that the utilization rate of ▪OH in the conventional Fenton system was very low and there was probably more •C-R formed in WAS pretreated by Fe(II)-activated CaO2 in the first 5 min of conditioning time. The organic intermediates formed in the first stage of the oxidation may react further with •OH and oxygen, with the overall process leading eventually to mineralization to CO2, H2O, and (if the contaminant contains heteroatoms) inorganic acids (Pignatello et al., Citation2006). It was also within the first 5 min that the maximum CST reduction efficiency can be obtained (). Hence, it could be concluded that •OH produced by Fe(II)-activated CaO2 had higher reaction efficiency with WAS organic components definitely, including those water-holding compounds, despite that higher concentration of •OH can be obtained by liquid Fenton reagent. This advantage contributed to the superior performance of Fe(II)-activated CaO2 on WAS conditioning.
Morphological analysis of WAS floc
The morphology of WAS floc before and after pretreatments was characterized through SEM. A typical structure of zoogloea can be observed in the SEM image of raw WAS (). The rod-shaped bacterial cells are entrapped by chain materials inside the sludge matrix. The chain materials are due to the presence of EPS in the form of proteins, polysaccharides, and lipids, which exist as long-chain polymers. EPS also bridge cells in floc formation with a chaotic macroporous structure. These microchannels maintain the capillary water and pose as a key obstacle for the deep dehydration of WAS.
Figure 5. SEM spectra of WAS: (a) raw sludge; (b) pretreated sludge (working voltage: 10 kV; magnification: 25000×).
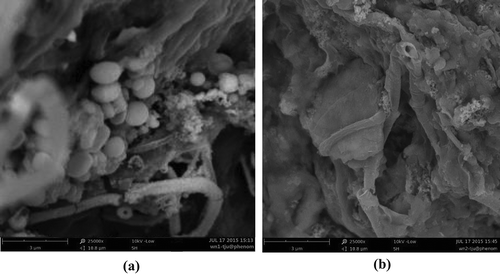
After the pretreatment of WAS under the optimal conditions obtained as described in the above section, no sign of cells attaching to or entrapped by EPS was observed, indicating that the process had already damaged the sludge floc structure and finally broken the cells completely (). The surface of pretreated sludge was smooth without prominent tiny pores. The degradation of EPS and the lysis of cells by radical oxidation transformed pretreated WAS into a homogeneous phase (Zhen et al., Citation2013). Accordingly, the interstitial water trapped inside the floc was released into the free water, which was favorable to the separation of the solid and liquid phases.
Correlation between EPS components and WAS dewaterability
MW distribution of EPS
The polymerization of EPS has a significant influence on the microstructure of WAS and can be characterized by the molecular weight (MW) distribution of EPS. The results are shown in . Two peaks at MWs of 0.3~0.35 and 30~35 kDa were present in the S-EPS of raw WAS; however, only one sharp peak at a MW of 1 kDa was detected in S-EPS from pretreated WAS. This remarkable difference was attributable to the hydrolysis of proteins and polysaccharides from bound EPS to form biomass-associated products (BAPs) (More et al., Citation2014; Zeng et al., Citation2010). For LB-EPS, the large decrease in the average MW from 39.142 to 7.465 kDa occurred after pretreatment by the Fe(II)-activated CaO2. LB-EPS brings a greater amount of bound water into the aggregates, producing highly porous sludge flocs with a lower density (Li and Yang, Citation2007). Such a sludge structure results in poor performance of sludge sedimentation and compression (Poxon and Darby, Citation1997). However, the decreasing MW of LB-EPS could lead to a denser microfloc structure and less pore water, which reduces the SRF by improving the compressibility of WAS (Li and Yang, Citation2007). shows the MW distribution of TB-EPS from raw and pretreated WAS. The peak area at a MW of 10 kDa increased significantly, but the peak of MW 0.25 kDa decreased after the sample was oxidized by Fe(II)-activated CaO2. The highly active •OH radicals inevitably caused the lysis of cells and the release of intracellular substances, such as nucleic acids and proteins (Zhen et al., Citation2012a). As a result, these macromolecules were extracted with TB-EPS, which led to an increase in the average MW of the TB-EPS fraction.
Composition of different EPS fractions
Proteins (PN) and polysaccharides (PS) are the major components of EPS (Li and Yang, Citation2007; More et al., Citation2014). The values of these components measured in this investigation are given in . As shown in , the PN concentrations were originally estimated to be 225.89 ± 4.76, 57.01 ± 1.95, and 829.68 ± 58.90 mg/L in the S-EPS, LB-EPS, and TB-EPS fractions, respectively, for the untreated WAS. After pretreatment, the PN concentration in TB-EPS declined to 89.39 ± 1.62 mg/L, decreasing by approximately 89.23%; in contrast, it increased to 557.55 ± 56.30 and 136.48 ± 5.85 mg/L in the S-EPS and LB-EPS fractions. The concentration of PS in raw and pretreated EPS displayed a similar pattern as for PN. These results illustrate that the degradation and transformation of PN and PS by the Fe(II)-activated CaO2 system were simultaneous and together contributed to the change of total EPS. As a result, the contents in the TB-EPS fraction were reduced profoundly after the conditioning, whereas those in LB-EPS and S-EPS were increased.
The concentration variation of the different EPS fractions mentioned above may be attributed to the fact that when the WAS floc is disrupted with radical oxidation, part of TB-EPS and intracellular substances are solubilized and released into the liquid phase, thereby increasing the S-EPS and LB-EPS levels. In general, a high LB-EPS content is detrimental to the WAS dewatering due to its stronger affinity for water through hydrophilic interactions and hydrogen bond forces (Bo et al., Citation2004). Nevertheless, the adverse impact on the dewatering has been controlled by the use of Fe(II)-activated CaO2, as demonstrated by the CST results of pretreated WAS in . This implies that radical oxidation accelerates the degradation of LB-EPS, which has been further verified by the GPC results mentioned above. The reduction of the MW in LB-EPS must play a significant role in improving the dewaterability of WAS. S-EPS, which are evenly distributed in the liquid phase, are more easily removed together with free water during the filtration process and therefore the influence of its increasing amount is definitely insignificant (Zhen et al., Citation2013).
EEM spectra of different EPS fractions
The content and molecular weight of EPS influence the colloidal structure of WAS significantly. In addition, the hydrophilic and hydrophobic properties of EPS are also controlling factors on the dewaterability of WAS, determining the content of bound water. EEM spectroscopy is a rapid and sensitive technique to determine the overall “fingerprint” of EPS mixtures in sludge floc (Zhen et al., Citation2012b). Therefore, EEM was applied to explore the migration and transformation of hydrophilic/hydrophobic substances and the responsible mechanisms that enhance dewaterability.
One main peak could be identified from the fluorescence spectra of S-EPS and LB-EPS in raw sludge ( and ). This main peak, located at the excitation/emission wavelengths (Ex/Em) of 275/325–395 nm, was attributed to aromatic protein-like substances, for example, tryptophan-like compounds (Wang et al., Citation2009). For the TB-EPS of raw WAS (), two peaks were located at an Ex/Em of approximately 290/315–365 nm and 340–380/425–475 nm. The first one was associated with tryptophan protein-like substances, and the second one was related to the humic acid–like organics and hydrophobic acids (Wang et al., Citation2009).
Figure 8. EEM spectra of different EPS fractions: (a) S-EPS of raw WAS; (b) S-EPS of pretreated WAS; (c) LB-EPS of raw WAS; (d) LB-EPS of pretreated WAS; (e) TB-EPS of raw WAS; (f) TB-EPS of pretreated WAS.
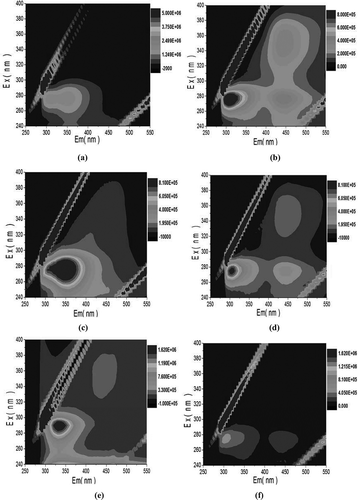
Compared with the S-EPS of raw sludge, the other two fluorescence peaks at an Ex/Em of approximately 275/425–475 nm and 340–370/425–450 nm occurred in the fluorescence spectra of S-EPS collected from pretreated WAS, which implied the dissolution of humic substances and fulvic acid-like materials, respectively, into the liquid phase during oxidation (Kunacheva and Stuckey, Citation2014). The intensity of humic acid-like substance and fulvic acid-like substance peaks also increased in the EEM spectrum of pretreated LB-EPS. The solubilization of some fluorescent substance from LB-EPS may cause the decrease of relative difference between the fluorescence intensities of protein-like matters and humic substances; as a result, the fluorescence peaks of humic acid-like substances were prominent, which also occurred in the previous report (Zhen et al., Citation2012c). In addition, for the LB-EPS of pretreated WAS, the location of the peak related to tryptophan-like protein showed a blue shift in the emission wavelength compared with that of raw LB-EPS, which implies the elimination of particular hydrophilic groups, such as carbonyl, hydroxyl, and amine groups, and a reduction in the number of aromatic rings and conjugated bonds in a chain structure (Wang et al., Citation2009; Liu et al., Citation2011). Therefore, although the content of LB-EPS increased during the oxidation process mentioned in the previous section, the deterioration of dewaterability caused by the increase of LB-EPS noted in previous reports still could be limited by the decrease in the number of hydrophilic groups in this investigation. The intensity reduction of the fluorescence peak before and after pretreatment was evident in the EEM spectra of raw TB-EPS and pretreated TB-EPS. This is in accord with the measured results of TB-EPS content. The enhanced dewaterability of WAS was closely related to the degradation and removal of the fluorescence components in TB-EPS, which was in agreement with previous reports that protein-like substances in EPS contribute more to the filtration resistance of membrane (Wang et al., Citation2009; Liu et al., Citation2011).
Zeta potential of EPS and bound water content
The surface properties of WAS are closely correlated with the stabilization of sludge floc and influence the flocculation and dewaterability. More importantly, the surface properties also pose effects on the water distribution in sludge floc by hydrogen bound and Van der Waals force. To explore the effects of Fe(II)-activated CaO2 on surface electrical properties, the zeta potential of EPS and the distribution of calcium and iron were measured simultaneously. The pH was controlled according to the pH of pretreated WAS (the pretreatment conditions: 0.05 g/g DS CaO2, 0.625 mmol/g DS Fe(II), and initial pH 2).
As shown in , the majority of cations were in the bulk solution (more than 50%), but the distribution of Ca is clearly different from that of Fe. Most of the Fe2+/Fe3+ concentrated in the bulk solution (81%) and less than 6.5% of the iron was bound to the LB-EPS and TB-EPS. By contrast, 15.7% and 25.8% of the calcium were bound to the LB-EPS and TB-EPS, respectively. The results in show that the absolute values of the zeta potential of S-EPS, LB-EPS, and TB-EPS all decreased greatly, which was inevitably due to the binding behavior of calcium and ferrous ions (Yu et al., Citation2016). According to DLVO (Derjaguin, Landau, Vervey, and Overbeek) theory, the decrease in the zeta potential must be accompanied by compressing the electric double layer (Li et al., Citation2012).
Table 1. Zeta potentials (mV) of different EPS fractions.
Based on overwhelming and diverse experiments, the term “vicinal water” or “interfacial water” refers to multiple layers of water molecules held tightly to the particle surface by hydrogen bonding in the electric double layer. Hydrogen bonding is believed to be short range but very intense, close to the particle surface, resulting in the appearance of highly structured water molecules (Vesilind, Citation1994). As a consequence, this part of water, interfacial water, in the electric double layer cannot be removed by conventional mechanical dewatering methods and is the main obstacle to deep dehydration of WAS. In this study, the compression of the double electric layer can lead to the reduction of interfacial water, so besides releasing interstitial water by destroying sludge floc, Fe(II)-activated CaO2 in addition can also transform interfacial water into bulk water by neutralizing the zeta potential and thinning the twin electrical layer. These results were partially verified by the decreasing bound water content measured by dilatometric method. The bound water content before and after the pretreatment of Fe(II)-CaO2 decreased from 2.119 ± 0.064 to 0.318 ± 0.039 g/g DS, which presaged that more water removal could be realized by mechanical dewatering method (Smith and Vesilind, Citation1995).
Conclusions
The Fe(II)-activated CaO2 was demonstrated to be effective in improving WAS dewaterability. Under the conditions comprising initial pH 2, Fe(II) of 0.625 mmol/g DS, and CaO2 of 0.05 g/g DS, an 85.7% reduction in CST and a 92.6% reduction in SRF were achieved. The improvement of dewaterability was dominated by •OH, and Fe(II)-activated CaO2 can achieve the more stable production and higher utilization rate of •OH compared with conventional Fenton reagent. The rupture of sludge colloidal flocs caused by the radical oxidation process was beneficial for the release of interstitial water, and the reduction of hydrophilic functional groups in loosely bound extracellular polymeric substances (LB-EPS) induced by •OH was found to be a contributing factor to the improvement of WAS dewaterability. In addition, the binding affinities of multivalent cations (Ca2+ and Fe2+/Fe3+ introduced by the Fe(II)-activated CaO2) for EPS thinned the twin electrical layer and transformed interfacial water into free water, which also eliminated a key obstacle to deep dehydration of WAS.
Funding
The authors wish to thank the Major Science and Technology Program for Water Pollution Control and Treatment (2013ZX07315-002) for the financial support of this study.
Additional information
Funding
Notes on contributors
Boran Wu
Boran Wu is a Ph.D. student focusing on the treatment and disposal of waste activated sludge.
Xiaoli Chai
Xiaoli Chai is a professor at State Key Laboratory of Pollution Control and Resource Reuse, Tongji University.
References
- Ai, Z., W. Jie, and Y. Li. 2015. Performance of calcium peroxide for removal of endocrine-disrupting compounds in waste activated sludge and promotion of sludge solubilization. Water Res. 71:125–139. doi: 10.1016/j.watres.2015.01.005.
- Arabi, S., and G. Nakhla. 2007. Impact of Calcium on the Membrane Fouling in Membrane Bioreactors. Proc. Water Environ. Fed. 314:134–142. doi: 10.1016/j.memsci.2008.01.037.
- Diak, J., B. Ormeci, and P. C. 2011. Freeze-thaw treatment of RBC sludge from a remote mining exploration facility in subarctic Canada. Water Sci. Technol. 63:1309–1313. doi: 10.2166/wst.2011.376.
- Feng, X., J. Deng, H. Lei, T. Bai, Q. Fan, and Z. Li. 2009. Dewaterability of waste activated sludge with ultrasound conditioning. Bioresour. Technol. 100:1074–1081. doi: 10.1016/j.biortech.2008.07.055.
- Flemming, H.C., and J. Wingender. 2001. Relevance of microbial extracellular polymeric substances (EPSs)—Part I: Structural and ecological aspects. Water Sci. Technol. 43(6):623–633.
- Gilcreas, F.W. 2012. Standard methods for the examination of water and wastewater. Am. J. Public Health Nations Health 56(3):113–X.
- He, D.Q., L.F. Wang, H. Jiang and H.Q. Yu. 2015. A Fenton-like process for the enhanced activated sludge dewatering. Chem. Eng. J. 272:128–134. doi: 10.1016/j.cej.2015.03.034.
- Jin, B., B.-M. Wilén, and P. Lant. 2004. Impacts of morphological, physical and chemical properties of sludge flocs on dewaterability of activated sludge. Chem. Eng. J. 98:115–126. doi: 10.1016/j.cej.2003.05.002.
- Kunacheva, C., and D.C. Stuckey. 2014. Analytical methods for soluble microbial products (SMP) and extracellular polymers (ECP) in wastewater treatment systems: A review. Water Res. 61(18):1–18. doi: 10.1016/j.watres.2014.04.044.
- Lee, E.J., V.I. Volkov, M.W. Byun, and C.H. Lee. 2002. Detection of free radicals in gamma-irradiated soybean paste and model system by electron spin resonance spectroscopy. Radiat. Phys. Chem. 64:61–66. doi: 10.1016/S0969-806X(01)00444-3.
- Li, X.Y., and S.F. Yang. 2007. Influence of loosely bound extracellular polymeric substances (EPS) on the flocculation, sedimentation and dewaterability of activated sludge. Water Res. 41:1022–1030. doi: 10.1016/j.watres.2006.06.037.
- Li, H., W. Yue, A. Cao, J. Huang, Z. Qi, and P. Somasundaran. 2012. The influence of additives (Ca2+, Al3+, and Fe3+) on the interaction energy and loosely bound extracellular polymeric substances (EPS) of activated sludge and their flocculation mechanisms. Bioresour. Technol. 114:188–194. doi: 10.1016/j.biortech.2012.03.043.
- Liang, J., S. Huang, Y. Dai, L. Lei, and S. Sun. 2015. Dewaterability of five sewage sludges in Guangzhou conditioned with Fenton’s reagent/lime and pilot-scale experiments using ultrahigh pressure filtration system. Water Res. 84:243–254. doi:10.1016/j.watres.2015.07.041
- Liu, Y., and H.H.P. Fang. 2003. Influences of extracellular polymeric substances (EPS) on flocculation, settling, and dewatering of activated sludge. Crit. Rev. Environ. Sci. Technol. 33:237–273.
- Liu, T., Z.L. Chen, W.Z. Yu, and S.J. You. 2011. Characterization of organic membrane foulants in a submerged membrane bioreactor with pre-ozonation using three-dimensional excitation-emission matrix fluorescence spectroscopy. Water Res. 45:2111–2121. doi: 10.1016/j.watres.2010.12.023.
- Mahmoud, A., J. Olivier, J. Vaxelaire, and A.F. Hoadley. 2010. Electrical field: A historical review of its application and contributions in wastewater sludge dewatering. Water Res. 44:2381–2407. doi: 10.1016/j.watres.2010.01.033.
- Mikkelsen, L.H., and K. Keiding. 2002. Physico-chemical characteristics of full scale sewage sludges with implications to dewatering. Water Res. 36:2451–2462. doi:10.1016/S0043-1354(01)00477-8
- More, T.T., J.S.S. Yadav, S. Yan, R.D. Tyagi, and R.Y. Surampalli. 2014. Extracellular polymeric substances of bacteria and their potential environmental applications. J. Environ. Manage. 144(144):1–25. doi: 10.1016/j.jenvman.2014.05.010.
- Muruganandham, M., and M. Swaminathan. 2004. Photochemical oxidation of reactive azo dye with UV–H2O2 process. Dyes Pigments 62:269–275. doi: 10.1016/j.dyepig.2003.12.006.
- Nam, K., W. Rodriguez, and J.J. Kukor. 2001. Enhanced degradation of polycyclic aromatic hydrocarbons by biodegradation combined with a modified Fenton reaction. Chemosphere 45:11–20. doi: 10.1016/S0045-6535(01)00051-0.
- Ndjou’Ou, A.C., and D. Cassidy. 2006. Surfactant production accompanying the modified Fenton oxidation of hydrocarbons in soil. Chemosphere 65:1610–1615. doi: 10.1016/j. chemosphere. 2006.03.036.
- Neyens, E., and J. Baeyens. 2003. A review of classic Fenton’s peroxidation as an advanced oxidation technique. J. Hazard. Mater. 98:33–50. doi: 10.1016/S0304-3894(02)00282-0.
- Northup, A., and D. Cassidy. 2008. Calcium peroxide (CaO2) for use in modified Fenton chemistry. J. Hazard. Mater. 152:1164–1170. doi: 10.1016/j.jhazmat.2007.07.096.
- Pignatello, J.J., E. Oliveros, and A. MacKay. 2006. Advanced oxidation processes for organic contaminant destruction based on fenton reaction and related chemistry. Crit. Rev. Environ. Sci. Technol. 36:1–84. doi: 10.1080/10643380500326564.
- Poxon, T.L., and J.L. Darby. 1997. Extracellular polyanions in digested sludge: Measurement and relationship to sludge dewaterability. Water Res. 31:749–758. doi: 10.1016/S0043-1354(96)00319-3.
- Pshezhetskiĭ, S.IA. 1974. EPR of Free Radicals in Radiation Chemistry. Sydney, Australia: Halsted Press.
- Qian, Y., X. Zhou, Y. Zhang, W. Zhang, and J. Chen. 2013. Performance and properties of nanoscale calcium peroxide for toluene removal. Chemosphere 91:717–723. doi: 10.1016/j.chemosphere.2013.01.049.
- Sheng, G.P., H.Q. Yu, and X.Y. Li. 2010. Extracellular polymeric substances (EPS) of microbial aggregates in biological wastewater treatment systems: A review. Biotechnol. Adv. 28:882–894. doi: 10.1016/j.biotechadv.2010.08.001
- Smith, J.K., and P.A. Vesilind. 1995. Dilatometric measurement of bound water in wastewater sludge. Water Res. 29:2621–2626. doi: 10.1016/0043-1354(95)00144-A.
- Tony, M.A., Y.Q. Zhao, J.F. Fu, and A.M. Tayeb. 2008. Conditioning of aluminium-based water treatment sludge with Fenton’s reagent: Effectiveness and optimising study to improve dewaterability. Chemosphere 72:673–677. doi: 10.1016/j.chemosphere.2008.03.032.
- Vesilind, P.A. 1994. The role of water in sludge dewatering. Water Environ. Res. 66:4–11. doi: 10.2175/WER.66.1.2.
- Wang, Z., Z. Wu, and S. Tang. 2009. Characterization of dissolved organic matter in a submerged membrane bioreactor by using three-dimensional excitation and emission matrix fluorescence spectroscopy. Water Res. 43:1533–1540. doi: 10.1016/j.watres.2008.12.033.
- Yang, G., G. Zhang, and H. Wang. 2015. Current state of sludge production, management, treatment and disposal in China. Water Res. 78:60–73. doi: 10.1016/j.watres.2015.04.002.
- Yu, G.H., P.J. He, L.M. Shao and D.J. Lee. 2007. Enzyme activities in activated sludge flocs. Appl. Microbiol. Biotechnol. 77:605–612. doi: 10.1007/s00253-007-1204-5.
- Yu, Q., H. Lei, G. Yu, X. Feng, Z. Li, and Z. Wu. 2009. Influence of microwave irradiation on sludge dewaterability. Chem. Eng. J. 155:88–93. doi: 10.1016/j.cej.2009.07.010.
- Yu, W., J. Yang, Y. Shi, J. Song, Y. Shi, J. Xiao, C. Li, X. Xu, S. He, S. Liang, X. Wu, and J. Hu. 2016. Roles of iron species and pH optimization on sewage sludge conditioning with Fenton’s reagent and lime. Water Res. 95:124–133. doi: 10.1016/j.watres.2016.03.016.
- Zhang, H., J. Yang, W. Yu, S. Luo, P. Li, X. Shen, Y. Shi, S. Zhang, J. Song, N. Ye, Y. Li, C. Yang, and S. Liang. 2014. Mechanism of red mud combined with Fenton’s reagent in sewage sludge conditioning. Water Res. 59:239–247. doi: 10.1016/j.watres.2014.04.026.
- Zhang, Q.A., Y. Shen, X.H. Fan, J.F.G. Martín, X. Wang, and Y. Song. 2015a. Free radical generation induced by ultrasound in red wine and model wine: An EPR spin-trapping study. Ultrason. Sonochem. 27:96–101. doi: 10.1016/j.ultsonch.2015.05.003.
- Zhang, W., Y. Peng, X. Yang, C. Zhan, and D. Wang. 2015b. Insights into the respective role of acidification and oxidation for enhancing anaerobic digested sludge dewatering performance with fenton process. Bioresour. Technol. 181:247–253. doi: 10.1016/j.biortech.2015.01.003.
- Zeng, R.J., G.P. Sheng, B.J. Ni, F. Fang, W.M. Xie, and H.Q. Yu. 2010. Fractionating soluble microbial products in the activated sludge process. Water Res. 44:2292–2302. doi: 10.1016/j.watres.2009.12.025.
- Zhen, G., X. Lu, X. Chai, D. Niu, Y. Li, B. Wang, Y. Zhao, A. Zhao, S. Yu, and X. Cao. 2012a. Synergetic pretreatment of waste activated sludge by Fe(II)-activated persulfate oxidation under mild temperature for enhanced dewaterability. Bioresour. Technol. 124:29–36. doi: 10.1016/j.biortech.2012.05.115.
- Zhen, G., X. Lu, Y. Li, Y. Zhao, B. Wang, S. Yu, X. Chai, D. Niu, and X. Cao. 2012b, Novel insights into enhanced dewaterability of waste activated sludge by Fe(II)-activated persulfate oxidation. Bioresour. Technol. 119:7–14. doi: 10.1016/j.biortech.2012.05.115.
- Zhen, G., X. Lu, B. Wang, Y. Zhao, X. Chai, D. Niu, and T. Zhao. 2014. Enhanced dewatering characteristics of waste activated sludge with Fenton pretreatment: Effectiveness and statistical optimization. Front. Environ. Sci. Eng. 8:267–276. doi: 10.1007/s11783-013-0530-3.
- Zhen, G., X. Lu, Y. Zhao, X. Chai, and D. Niu. 2012c. Enhanced dewaterability of sewage sludge in the presence of Fe(II)-activated persulfate oxidation. Bioresour. Technol. 116:259–265. doi: 10.1016/j.biortech.2012.01.170.
- Zhen, G.Y., X.Q. Lu, Y.Y. Li, and Y.C. Zhao. 2013. Innovative combination of electrolysis and Fe(II)-activated persulfate oxidation for improving the dewaterability of waste activated sludge. Bioresour. Technol. 136:654–663. doi: 10.1016/j.biortech.2013.03.007.