ABSTRACT
Burning natural gas in power plants may emit radon (222Rn) into the atmosphere. On the University Park campus of The Pennsylvania State University, atmospheric radon enhancements were measured and modeled in the vicinity of their two power plants. The three-part study first involved measuring ambient outdoor radon concentrations from August 2014 through January 2015 at four sites upwind and downwind of the power plants at distances ranging from 80 m to 310 m. For each plant, one site served as a background site, while three other sites measured radon concentration enhancements downwind. Second, the radon content of natural gas flowing into the power plant was measured, and third, a plume dispersion model was used to predict the radon concentrations downwind of the power plants. These predictions are compared to the measured downwind enhancements in radon to determine whether the observed radon concentration enhancements could be attributed to the power plants’ emissions. Atmospheric radon concentrations were consistently low as compared to the EPA action level of 148 Bq m−3, averaging 34.5 ± 2.7 Bq m−3 around the East Campus Steam Plant (ECSP) and 31.6 ± 2.7 Bq m−3 around the West Campus Steam Plant (WCSP). Significant concentrations of radon, ranging from 516 to 1,240 Bq m−3, were detected in the natural gas. The measured enhancements downwind of the ECSP averaged 6.2 Bq m−3 compared to modeled enhancements of 0.08 Bq m−3. Measured enhancements around the WCSP averaged −0.2 Bq m−3 compared to the modeled enhancements of 0.05 Bq m−3, which were not significant compared to observational error. The comparison of the measured to modeled downwind radon enhancements shows no correlation over time. The measurements of radon levels in the vicinity of the power plants appear to be unaffected by the emissions from the power plants.
Implications: Radon measurements at sites surrounding power plants that utilize natural gas did not indicate that the radon concentrations originated from the plants’ emissions. There were elevated radon concentrations in the natural gas supply flowing into the power plants, but combustion dilution puts the concentration below EPA action levels coming out of the stack, so no hazardous levels were expected downwind. Power plant combustion of natural gas is not likely to pose a radiation health hazard unless very different gas radon concentrations or combustion dilution ratios are encountered.
Introduction
Radon (222Rn) is a naturally occurring radioactive material (NORM) contained in the earth’s crust, a product of the decay series of uranium (238U) (Anspaugh, Citation2012). The element is a noble gas, so it does not react with other substances as it seeps up through the ground, and it is colorless and odorless, so not readily detectable. The fact that it seeps into and accumulates in buildings has caused radon to become the second leading cause of lung cancer in the United States (Pawel and Puskin, Citation2004). The EPA recommends mitigation of buildings whose annual mean radon concentration exceeds 148 Bq m−3 (EPA 402-R-03-003, Citation2003). While high levels of radon are typically found in buildings and uranium mines, there is concern about increased outdoor exposure due to the extraction of natural gas from uranium-rich areas, such as the Marcellus Shale in Pennsylvania (Rowan and Kraemer, Citation2012). Radon’s radioactive half-life is 3.8 days, which may be within the range of pipeline transit time to local power plants, and therefore gas-burning power plants could emit radon. It is imperative, therefore, to test the radon content of natural gas and outdoor radon levels around power plants.
Estimates of atmospheric radon concentrations in the contiguous United States range from 3.7 to 14.8 Bq m−3 (Gesell, Citation1983), and a typical measurement is about 10 Bq m−3, yet measurements have exceeded 100 Bq m−3 in areas of “high radon exhalation” (UNSCEAR, Citation2000). The National Ambient Radon Study measured an annual mean of 18.13 Bq m−3 in Harrisburg, PA (Hopper et al., Citation1991). Ambient air samples collected at a variety of sites across Pennsylvania yielded a larger range of 7.4 to 25.9 Bq m−3 (Perma-Fix Environmental Services, Citation2015).
High concentrations of radon have been detected in natural gas. The U.S. Geological Survey measured several samples at producing wells from the Upper Devonian sandstones and the Middle Devonian Marcellus Shale in Pennsylvania, finding radon levels that ranged from 37-2,920 Bq m−3 (Rowan and Kraemer, Citation2012). However, Jenkins et al. (Citation2014) determined a calibration factor for methane that corrects these values to 23.9 to 1,890 Bq m−3. In turn, the Pennsylvania Department of Environmental Protection (PADEP) measured natural gas at several sites throughout the operation system, including production sites, well sites, compressor stations, a processing plant, and two natural-gas-fired power plants. The overall range of radon measured at all sites is 111 to 5,460 Bq m−3 (Perma-Fix Environmental Services, Citation2015). These studies establish that radon is present in Pennsylvania’s natural gas supply, but they do not determine to what extent it contributes to the radon content of the atmosphere where the gas is burned.
The Pennsylvania State University (Penn State) in University Park, PA, has two steam generation plants. One, a steam-electric cogeneration facility, is adjacent to the downtown area of State College, PA. Having recently converted its primary fuel source from coal to natural gas, the plant now includes a much lower stack height. This situation necessitates an evaluation of the potential risk of radon emissions to local inhabitants. The second plant, more distant from the downtown area, has always been powered by natural gas. In this research project, we seek to determine the radon concentration in the natural gas supply to and in the atmosphere downstream from both plants. We want to determine whether observed atmospheric concentrations of radon in the vicinity of either steam plant can be attributed to its presence in the natural gas.
Materials and methods
Overview
The University Park Campus of Penn State has two power plants, providing electricity and steam to the university (). The East Campus Steam Plant (ECSP), built in 1972, burns natural gas with a backup of oil, and the West Campus Steam Plant (WCSP), built in 1929, burns both natural gas and coal at the present time. To determine whether radon in the natural gas is affecting the surrounding atmosphere, we first measured radon in the vicinity of these plants ( and ) for several months. The second step involved measuring the radon concentration of natural gas samples. Finally, we used the radon concentration in the natural gas in the source term of a simple Gaussian plume dispersion model to simulate the concentration of radon at each site around the plants in order to compare with the measurements.
Table 1. Locations of the steam plant stacks and radon measurement sites, and height above ground, noting that the elevation of University Park, PA is 377.6 m.
Ambient outdoor radon
Four sites were chosen around each plant to measure radon (). For the ECSP, these sites included the Bleachers and Morning Star House, which are typically upwind from the plant, and the Woods and Swine Farm, both typically downwind from the plant (). The ECSP resides in a small valley, and has a relatively short stack, so the measurement sites are at or above stack height (). The Bleachers site is over pavement, while the Morning Star House and Swine Farm sites are over grass. The Woods site is over natural ground cover, predominately long grass and brush. These ground characteristics could affect the exposure of these sites to radon from the soil. For the WCSP, the sites are all on top of surrounding buildings. The WCSP stack is 59.1 m tall, so the rooftop measurements are more likely to capture the plume than measurements at ground level. The rooftop sites include Walker Building, two sites on either end of Reber Building, named Reber North and Reber South, and Rider House ( and ). Walker is typically upwind, while Reber North, Reber South, and Rider House are typically downwind. It should be noted that Rider House has a much lower rooftop than the other sites, with a higher building between it and the power plant. The two upwind sites, Walker in the west and the Bleachers in the east, were considered as background. These eight sites were monitored regularly for 161 days from August 22, 2014, through January 29, 2015.
Figure 2. Stacked bar graph of wind speed categories for each set of wind directions. Each bar is a 30-degree set of wind directions, and the shades represent the strength of the wind in that direction. Wind data is taken from Walker Building’s weather station from 8/22/14 through 1/29/15.
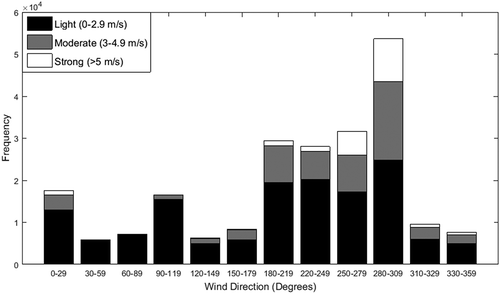
Each site had three radon detectors called electret ion chambers (EICs, Rad-Elec, Inc.), or electret passive environmental radon monitors (Kotrappa, Citation1990). EICs consist of a Teflon disk, called an electret, which is charged with a voltage and mounted inside a small chamber made of electrically conducting plastic, which minimizes the response from natural gamma radiation. When alpha particles are emitted by radon decay, they ionize air molecules, and negative ions in the chamber are collected on the positively charged electret, causing a reduction of its voltage. Short term electrets were fitted into a short-term S chamber, together called an SST, and were deployed to each site for typically 2-week periods. The voltages of the electrets are measured before and after deployment, and the drop in voltage is used to calculate a radon concentration that represents an average over the entire deployment period. The lower limit of detection of the SSTs is 11–12 Bq m−3 for a 7-day measurement (Kotrappa, Citation1990). This random sampling error varies as the inverse of the square root of the exposure time.
EICs are designed to measure indoor air, so it was necessary to weatherproof them at each site. Sets of three EICs were placed in Tyvek bags along with desiccant packets (Stieff et al., Citation2012). Tyvek is impervious to liquid water, but not impervious to water vapor or to radon, so the desiccant prevented humid air from condensing into liquid water, which would discharge the electrets. The bags were placed into vertical hanging wire baskets at each site around ECSP, hanging under the bleachers, on the back of a shed at the Morning Star House, on a tree in the woods, and on a railing at the Swine Farm. They ranged from 1 to 2 m off of the ground. On the rooftops around WCSP, tripods were fitted with wire baskets on which the bags were placed. Then, to prevent snow from blocking the airflow, a protective plastic basket was attached over each of them. They all sat approximately 1 m above the surface of the roof. All EICs experienced outdoor ambient temperature and pressure.
The EICs and Tyvek bags were tested for quality assurance before they were deployed in the field. Twenty-six EICs were placed in the basement of Walker Building and measured in two rounds: (1) EICs 1–12 inside the manufacturer’s test boxes, which have a large hole on either side to allow air to enter in, and EICs 13–26 were placed in Tyvek bags, and (2) EICs 1–12 in Tyvek bags and EICs 13–26 in the manufacturer’s test boxes. In each test round the radon concentrations were averaged for the set in the bags and compared with the average of those in the boxes.
The EICs calculate a radon concentration, c, in picocuries per liter (pCi L−1) as follows (E-PERM Manual, V.2.321):
The initial voltage, I, and the final voltage, F, of each electret are measured by a voltmeter at the start and finish of each deployment. The number of days of exposure, D, is recorded to the nearest minute. The background gamma level, BG (μR hr−1), was measured seasonally at each site with a Ludlum model 9DP pressurized ion chamber meter (Ludlum Measurements, Inc., Sweetwater, TX). G is the gamma conversion constant, and is 0.087 for the SST E-PERM configuration. The elevation correction factor, Elev CF, is 1.0 if the elevation is less than or equal to 4000 ft (1219.2 m); Penn State’s elevation is 1239 ft (377.6 m). Lastly, the calibration factor, CF, is calculated using
where, for SSTs, the constants are A = 1.69776 and B = 0.0005742. The resulting radon concentration is in standard units for radon measurement in the United States, pCi L−1, which multiplied by 37 gives the International System of Units measure of becquerels per cubic meter, Bq m−3.
The data set includes several weeks of radon concentrations, taken in triplicate. Each triplet was averaged, and then, to differentiate the effect of the power plants’ emissions at the sites, the average background was subtracted, leaving the enhanced levels of atmospheric radon. The Bleachers site is the background around the ECSP and Walker Building is the background around the WCSP because they are predominately upwind from the power plants. The background sites should primarily represent naturally occurring radon levels, having been minimally influenced by emissions from the power plants.
Finally, the background gamma radiation (BG) was measured on three occasions at each site (). The total radiation was measured on each occasion for 10 min. This source of radioactivity could be a source of error in measuring the radioactivity due to radon. An increase of 1 μR hr−1 of gamma radiation changes the apparent radon concentration (eq 1) by approximately 3.5 Bq m−3. It was necessary, therefore, to measure the gamma radiation at our sites about every month or two.
Table 2. Gamma measurements taken at sites around ECSP and WCSP in µR/hr.
Radon in natural gas
Radon monitors are not designed for sampling gases other than dry air. To safely take samples of natural gas, which is mostly flammable methane, we used a Pylon AB6A radon monitor with 600A active detector cells (Pylon Electronics Inc., Ottawa, ON, Canada). Three samples were taken from the WCSP once a week for 9 weeks by filling Tedlar bags, which were then evacuated into the detectors. These detectors are scintillation cells, commonly known as Lucas cells. They safely enclose the natural gas while the monitor detects the radon by sensing the radioactivity through a window on one end of the cell. The inner lining of the Lucas cell is coated with silver-activated zinc sulfide (ZnS) that produces light pulses when struck by the alpha particles that are emitted during radioactive decay. The monitor then has a photomultiplier tube that counts the light pulses. After taking into account radon’s radioactive daughters, which can also emit alpha particles, the monitor returns a radon concentration at the ambient temperature and pressure of the laboratory in which the samples were measured.
It is necessary to adjust the reported radon concentration, calibrated for dry air, to natural gas due to the different molecular sizes in these gas mixtures. The radioactive decay of radon releases alpha particles that travel farther on average in natural gas (mostly methane) than in air. This allows for increased interaction of the alpha particles with the internal surface of the Lucas cell, leading to greater counting efficiencies (Kitto et al., Citation2014). Since 5.1% more net counts are observed for radon in methane than in air, the calibration factor used by a Pylon AB6A calibrated in dry air must be multiplied by 1.051 (Jenkins et al., Citation2014). Therefore, we divide the reported radon concentration by 1.051 to get radon concentration for natural gas.
Natural gas samples were taken in triplicate, so the three readings are averaged and the standard deviation of the mean is determined for each set. Occasionally there was a procedural error in transferring the gas samples from the Tedlar bags into the Lucas cells. In these cases, the other two samples are averaged and the error is calculated with a sample size of 2. All discarded values were significantly lower than the other readings in the sample set.
Dispersion model
The concentration at each site over each measuring period was determined through a simple Gaussian plume dispersion equation for a continuous point source under steady-state conditions (Arya, Citation1999):
The concentration, c (Bq m−3), computed at each receptor for every half hour, is a function of the horizontal coordinates x and y, which are the downwind and crosswind distances (m) from the stack to the site, respectively, the wind speed, (m sec−1), the wind direction, the dispersion coefficients,
and
(m), and the radon emission rate, Q (Bq sec−1). Here, the vertical coordinate, z, is assumed to be 0, so the model assumes no vertical displacement from the stack to the receptor site. This simple approximation was chosen to yield the maximum possible concentration that could be observed at each receptor site. The source term, Q, is the emission rate of radon (Bq sec−1), based on the estimated radon concentration in the natural gas (Bq m−3) and the volumetric rate of gas burned at the plant during each measurement period (m3 sec−1). The natural gas usage is given as a daily volume in cubic feet, corrected to standard temperature (60ºF) and atmospheric pressure (Penn State Steam Services Plant Data Input File, 2014–2015), then converted to cubic meters per second assuming a constant rate of consumption during the day. Since the radon in natural gas was not monitored at the same time that downwind atmospheric measurements were conducted, we use the maximum concentration found in the gas to provide an upper limit for the radon concentration.
The radiation data are from the Penn State station of the SURFRAD (Surface Radiation) Network of NOAA’s Earth System Research Laboratory (Citation2015), and the wind data were measured by Penn State’s Department of Meteorology’s weather station on Walker Building. These data are collected every minute and are then averaged over each half hour from August 22, 2014, to January 29, 2015. The wind direction data were used to compute the downwind, x, and crosswind, y, distances from the stack to each receptor site.
The horizontal and vertical dispersion coefficients, and
respectively, are calculated according to the Pasquill stability type and using Briggs’s Interpolation Formulas for Open Country (Briggs, Citation1973). The Pasquill stability type was determined by dividing the net radiation, the total sum of incoming and outgoing longwave and shortwave radiation, into the following categories: strong, moderate, neutral, and stable. This, together with the surface (10 m) wind speed, yielded the Pasquill stability classes of extremely unstable (A), moderately unstable (B), slightly unstable (C), neutral (D), slightly stable (E), or moderately stable (F), shown in , adapted from Turner (Citation1970).
Table 3. Pasquill stability classes, adapted from Turner (Citation1970): A = extremely unstable, B = moderately unstable, C = slightly unstable, D = neutral, E = slightly stable, F = moderately stable.
The modeled concentrations are then compared with the observations at the eight sites around the two power plants. To accomplish this, the concentrations generated by the model for each site are averaged over each 2-week observation period. The averages of the background sites are then subtracted from the other sites’ averages to compute the modeled enhancements. These modeled enhancements of radon can then be compared with the observed enhancements at each site.
Statistical analysis
The random error, ET (%), in each EIC measurement was evaluated using
where ET, E1, E2, and E3 are all independent contributions to the total random error (Kotrappa, Citation1990). The first error component, E1, includes system component imperfections, and is experimentally determined to be about 5%. The second error component, E2, accounts for a one-volt uncertainty in both the initial and final electret readings. The third error component, E3, assumes a 10% error in the gamma background.
To independently determine this random error estimate, all 2-week, three-instrument measurements were collected, averaged, and then the three-instrument mean was subtracted to give the residual readings. Since each three-instrument deployment should measure the same radon concentration, this residual is random error. A total of 220 radon residual readings were then formed into a histogram to show the frequency with which the measurements differ from the mean. These results were compared to the instrumental errors estimated using eq 4.
To determine whether the observed radon concentrations enhancements are attributable to the power plants, the time-varying gas usage together with meteorology was used to compute a best estimate of time-varying radon concentrations using eq 3. These were computed for each site and averaged over the same time periods during which the radon detectors were deployed. The magnitude and time variability of the observed atmospheric enhancements were then compared with the modeled enhancements to test whether the observed radon concentration enhancements could be caused by the combustion of natural gas at the power plants.
New WCSP
The WCSP will complete its conversion from coal to natural gas in 2016, raising the natural gas consumption rate above that during the time of this study. To determine the potential effect, the current use of coal is converted into an equivalent value of natural gas that would be needed to produce the same amount of energy output. These daily values are added to each day’s current amount of natural gas used at WCSP. This hypothetical set of gas consumption rates is then put through the same dispersion model, again using the maximum value of radon measured in the gas and the same meteorological conditions. The 161-day average using this hypothetical gas consumption rate is determined for each site around the WCSP, representing an estimate of radon concentrations that might be experienced after the plant conversion is complete.
Results and discussion
Ambient outdoor radon
The test of the set of 26 EICs in the Tyvek bags versus the regular E-PERM boxes confirmed the results reported by Stieff et al. (Citation2012). The test showed no significant differences in radon detection through the Tyvek. In Round 1, the EICs were exposed for 3.11 days in one location in the basement of Walker Building at ambient temperature and pressure. EICs 1–12 had an average radon concentration of 66.6 Bq m−3 in the boxes, while EICs 13–26 averaged 70.3 Bq m−3 in the Tyvek bags. The standard error of each set of EICs is 2.8 Bq m−3. In Round 2, the EICs were exposed for 4.83 days in the same location as before. EICs 1–12 had an average radon concentration of 81.4 Bq m−3 in the Tyvek bags, while EICs 13–26 averaged 85.1 Bq m−3 in the boxes. The standard error of each set of EICs is 2.1 Bq m−3. Both differences are within the range of random error; any influence of the Tyvek on the EIC readings is below the detection limit of this test. The readings from the Tyvek bags is assumed to represent atmospheric concentrations without any adjustments.
On average, eq 4 yields a random error estimate for each EIC measurements of 3.7 Bq m−3 for the conditions found in this study. The distribution of the residuals in the EICs’ readings has a standard deviation of 4.7 Bq m−3 (), slightly greater than the theoretical estimate. From this point, the more conservative value of 4.7 Bq m−3 is used to represent the random error in a single EIC measurement.
Figure 3. Residual radon readings from 220 individual measurements of ambient radon. Each mean of biweekly readings taken in triplicate was removed from these measurements to determine residuals. Residuals represent instrumental random error. The mean of the entire set is 32.9 Bq m−3, and the standard deviation is 4.7 Bq m−3, as compared to the manufacturer’s instrumental random error estimate of 3.7 Bq m−3. Two residual values were removed because they were higher than 4 standard deviations above the mean.
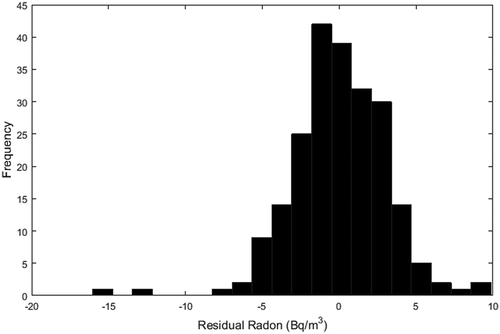
Atmospheric radon was monitored at eight sites, measured approximately biweekly from August 2014 through January 2015, at ambient outdoor temperature and pressure. The 5-month average radon concentration for each site is shown in . and present time-series plots of the same data set. The range of radon concentrations is 27 to 43 Bq m−3, far below the EPA action level for an annual mean concentration of 148 Bq m−3.
Table 4. Average radon concentrations and enhancements vs. background for each site from 9/4/14 to 1/8/15 for WCSP, 8/22/15 to 1/29/15 for ECSP.
Figure 4. Radon concentrations measured around ECSP from 8/22/14 to 1/29/15. Each reading is representative of the radon concentration integrated over 1 to 2 weeks with a standard deviation of 4.7 Bq m−3, as calculated (). Dates and values of each reading are shown in Table S.1.
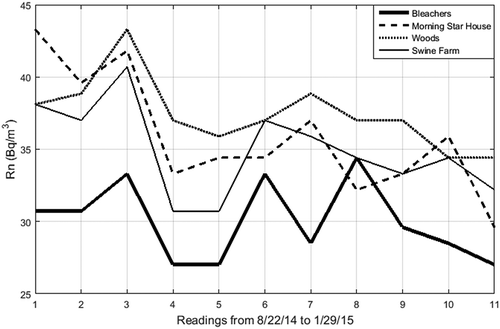
Figure 5. Radon concentrations measured around WCSP from 9/4/14 to 1/8/15. Each reading is representative of the radon concentration integrated over 1 to 2 weeks with a standard deviation of 4.7 Bq m−3, as calculated (). Dates and values of each reading are shown in Table S.1.
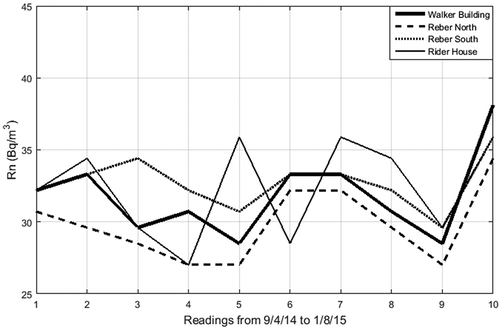
Significant spatial differences do exist across the ECSP measurement network. At the WCSP, the enhancements in radon activity level relative to our background site are only moderately larger than the standard errors (), suggesting few to no significant spatial gradients at that site. At the ECSP, however, the enhancements in radon activity level at the downwind sites are significant.
The downwind enhancement in the concentration of radon ranges from –5 to 12 Bq m−3 throughout the measurement period (). The highest average radon concentration enhancements occur at Rider House near the WCSP and the Woods near the ECSP, both of which are often downwind from their respective power plants (). Around the ECSP, the enhancements at all non-background sites are similar (). Around the WCSP, the enhancements at Reber North are always negative (below background), while at Reber South they are often positive ().
Little to no significant temporal variability in radon activity level is detected. When comparing the standard deviation of the mean enhancement at each site () to the estimated instrumental standard error for each enhancement (1.2 Bq m−3), the standard deviation of the mean for each site is slightly less than the estimated instrumental error. Any significant temporal variability would cause the observed standard error, a combination of all sources of variability, to exceed the instrumental random error. These results are inconsistent with a detectable wind-borne source of radon from the power plants since such a source would be time varying.
In summary, while the temporal variations do not support the existence of a radon source from the ECSP, and the detected enhancement is very small compared to the levels that would indicate a health concern, a significant enhancement in radon concentration downwind of the ECSP exists.
Radon in natural gas
Samples of natural gas from the WCSP collected in May through July 2015 show radon concentrations similar to those found in other studies of natural gas in the Marcellus shale region. The sampled radon concentrations from the WCSP range from 516.4 to 1,242 Bq m−3 (). The overall average of nine sets of measurements taken in triplicate from May 7 through July 2, 2015, is 892.5 Bq m−3. The minimum detectable concentration for these samples is 34 Bq m−3, based on 10-min background counting time, 30-min sample counting time, and 1 count per minute background count rate (Pylon Electronics, Inc., Citation2014). In order to investigate the worst possible conditions for a health threat, and because we did not have radon measurements for the natural gas that was burned at the time of the ambient measurements, we chose to use the maximum value measured, 1,242 Bq m−3. This value is used in the dispersion model’s source term, along with the gas consumption rate for the power plant (m3 sec−1), to get the emission rate, Q (Bq sec−1).
Table 5. Radon measured in natural gas samples from WCSP, with the standard deviation of each sample set, and the average and standard deviation for the 9 sample averages, where n is the number of natural gas samples in each set.
A first check for comparison of the radon emissions to the EPA action level is to dilute a known radon concentration in the natural gas by the plant’s air-to-fuel ratio. The ECSP has a 9:1 ratio of the volume of air to natural gas coming out of the stack, while the WCSP has a 12:1 ratio. The maximum measured radon concentration in the natural gas, 1,242 Bq m−3 would dilute to 138 Bq m−3 at the ECSP and 103.5 Bq m−3 at the WCSP before being dispersed in the atmosphere. According to these measurements, the emissions are below the EPA action level of 148 Bq m−3 upon exiting the stacks. Dispersion into the atmosphere will further reduce these concentrations.
Model output compared to observations
The dispersion model, which gives a worst-case estimate, predicts radon concentrations far below the levels that would be considered a health threat (). The predicted 2-week enhancements at the sites around the ECSP range from –0.01 to 0.60 Bq m−3 (not shown), with 161-day mean enhancements, which range across sites from 0.03 to 0.17 Bq m−3 (). Two-week average enhancements (not shown) around the WCSP range from –0.30 to 1.24 Bq m−3, with 161-day mean enhancements ranging from –0.06 to 0.23 Bq m−3 (). Given the estimated random error of 1.2 Bq m−3 in the long-term average measurements of the radon concentration enhancements (), these dispersion signals would be undetectable. Further, this conservative dispersion estimate predicts concentration enhancements from the ECSP steam plant that are 10 to 100 times lower than the observed enhancements at this site (). The observed and predicted sign of enhancements at the WCSP are anticorrelated across the sites. The observed and modeled radon enhancements also showed no significant temporal correlations (not shown). The disagreement between model and observation in the magnitude and sign of the mean enhancements suggests that the burning of natural gas containing radon does not cause the observed enhancements downwind of the ECSP, and supports the finding of no significant enhancements at the WCSP.
Table 6. Average radon concentrations and enhancements around the ECSP and WCSP, modeled with meteorological conditions and natural gas load from 8/22/14–1/29/15, compared to the observed enhancements over that time period.
New WCSP
Once the conversion of the WCSP is completed in 2016, all of the fuel will be natural gas. To estimate the dispersion of radon, the current natural gas consumption is added to an amount of natural gas that would be needed for the same energy output that the coal previously provided. This natural gas consumption rate was then modeled over the same time period and under the same meteorological conditions as previously modeled. The maximum measured radon concentration, 1,242 Bq m−3, is again applied to the natural gas for the entire time period, and the vertical displacement from stack to site is again neglected.
The estimated average radon concentrations around the converted WCSP are predicted to be 0.26 Bq m−3 at Walker Building, 0.85 Bq m−3 at Reber North, 0.26 Bq m−3 at Reber South, and 0.04 Bq m−3 at Rider House. These values are not enhancements; they are the concentrations that would be expected to be delivered to the sites by the power plant emissions. Obviously, all of the values are very far below the EPA action level. These results do not suggest that any health concern with radon will emerge from the converted natural gas plant.
Conclusion
Radon concentrations have been measured at sites surrounding two power plants that utilize natural gas on the Penn State University Park campus, and in the natural gas flowing into the power plants. Consistent with recent studies of radon in natural gas extracted from the Marcellus Shale formation in Pennsylvania, elevated radon was found in the natural gas supply to the campus power plants. Combustion dilution, however, puts the concentration below EPA action levels coming out of the stack, so no hazardous levels were expected downwind. In fact, no signal of power plant emissions could be detected in the atmosphere downwind of the plants. Small but statistically significant enhancements found downwind of the ECSP could not be attributed to emissions from the ECSP, and could be caused by spatial variability in radon emissions from the soil. This work suggests that power plant combustion of natural gas is not likely to pose a radiation health hazard unless very different gas radon concentrations or combustion dilution ratios are encountered.
Suplemental Table S1.docx
Download MS Word (142.5 KB)Funding
This work was funded by The Pennsylvania State University. We acknowledge Paul E. Moser’s assistance with data concerning gas consumption at the steam plants, access to the natural gas supply, and access to rooftop sampling sites, and Phil Jenkins’s assistance with calibration of the Pylon AB6A.
Supplemental data
Supplemental data for this article can be accessed on the publisher’s website.
Additional information
Funding
Notes on contributors
Alison G. Stidworthy
Alison G. Stidworthy is a site manager for the publicly funded element of the Bureau of Site Management at the New Jersey Department of Environmental Protection (2016).
Kenneth J. Davis
Kenneth J. Davis, Ph.D., is a professor of meteorology at Penn State University where he teaches and conducts research on the boundary layer and turbulence, climate, and earth–atmosphere interactions.
Jeff Leavey
Jeff Leavey is the manager of radiation protection for the Office of the Physical Plant at Penn State’s University Park Campus.
References
- Anspaugh, L.R. 2012. Scientific Issues Concerning Radon in Natural Gas, Texas Eastern Transmission, LP and Algonquin Gas Transmission, LLC, New Jersey–New York Expansion Project, Docket No. CP11-56. 58 pp.
- Arya, S.P. 1999. Air Pollution Meteorology and Dispersion. New York, NY: Oxford University Press.
- Briggs, G.A. 1973. Diffusion estimation of small emissions. Contribution No. 79, Atmospheric Turbulence and Diffusion Laboratory, Oak Ridge TN.
- EPA 402-R-03-003. 2003. Lifetime risk of lung cancer deaths from EPA Assessment of Risks from Radon in Homes. http://www.epa.gov/radon/index.html.
- E-PERM® System User’s Manual. Version 2.321. Rad Elec, Inc., Frederick, MD.
- Gesell, T.F. 1983. Background atmospheric 222Rn concentrations outdoors and indoors: A review. Health Phys. 45(2): 289–302. doi:10.1097/00004032-198308000-00002
- Hopper, R.D., R.A. Levy, R.C. Rankin, and M.A. Boyd. 1991. National ambient radon study. Proceedings of the 1991 International Symposium on Radon and Radon Reduction Technology, April 2–5, 1991, Philadelphia, PA. Research Triangle Park, NC: U.S. Environmental Protection Agency, 1991.
- Jenkins, P.H., J.F. Burkhart, and R.E. Camley. 2014. Errors in measurements of 222Rn in methane and carbon dioxide using scintillation cells calibrated for 222Rn in air. Health Phys. 106:397–404. doi: 10.1097/HP.0b013e3182a1a88c
- Kitto, M.E., M.A. Torres, D.K. Haines, and T.M. Semkow. 2014. Radon measurement of natural gas using alpha scintillation cells. J. Environ. Radioactivity 138: 205–7. doi:10.1016/j.jenvrad.2014.09.005
- Kotrappa, P., J.C. Dempsey, R.W. Ramsey, and L.R. Stieff. 1990. A practical E-PERM® system for indoor 222Rn measurement. Health Phys. 58(4): 461–67. doi:10.1097/00004032-199004000-00008
- Pawel, D.J., and J.S. Puskin. 2004. The U.S. Environmental Protection Agency’s assessment of risks from indoor radon. Health Phys. 87(1): 68–74. doi:10.1097/00004032-200407000-00008
- Perma-Fix Environmental Services, Inc. 2015. Technologically Enhanced Naturally Occurring Radioactive Materials (TENORM) study report. Pennsylvania Department of Environmental Protection. Rev. 0, 200 pp. http://www.dep.pa.gov/Business/Energy/OilandGasPrograms/OilandGasMgmt/Oil-and-Gas-Related-Topics/Pages/Radiation-Protection.aspx
- Pylon Electronics, Inc. 2014. Pylon® model AB6A radiation monitor manual. Ottawa, ON, Canada. No. 7940010.
- Rowan, E.L., and T.F. Kraemer. 2012. Radon-222 content of natural gas samples from Upper and Middle Devonian sandstone and shale reservoirs in Pennsylvania: Preliminary data. USGS Open-File Report Series 2012–1159. http://pubs.usgs.gov/of/2012/1159/
- Stieff, A., P. Kotrappa, and F. Stieff. 2012. The use of barrier bags with radon detectors. American Association of Radon Scientists and Technologists 2012 Symposium, 67–79. Rad Elec., Inc., Fredrick, MD. doi:10.1021/ed200071d
- SURFRAD (Surface Radiation) Network, National Oceanic and Atmospheric Administration, Earth System Research Laboratory. 2015. http://www.esrl.noaa.gov/gmd/grad/surfrad
- Turner, D.B. 1970. Workbook of atmospheric dispersion estimates. Office of Air Programs pub. no. AP-26. Research Triangle Park, NC: U.S. Environmental Protection Agency.
- United Nations Scientific Committee on the Effects of Atomic Radiation. 2000. United Nations Scientific Committee on the Effects of Atomic Radiation (UNSCEAR) 2000 Report to the General Assembly, Vol. 1, Annex B: Exposures from natural radiation sources. http://www.unscear.org/unscear/en/publications/2000_1.html